Synthesis and physico-chemical properties of the first water soluble Cu(II)@hemicryptophane complex†
Received
30th September 2014
, Accepted 10th December 2014
First published on 10th December 2014
Abstract
A hemicryptophane ligand soluble in water at neutral pH was obtained thanks to the derivatization of the cyclotribenzylene unit with three carboxylate groups. The corresponding Cu(II) complex was then synthesized and its spectroscopic and electrochemical properties in water were investigated, showing that water solubilisation retains the geometry of the complex around the metal center but strongly affects its redox properties, compared to previously reported Cu(II)@hemicryptophane complexes soluble in organic solvents.
Introduction
Hemicryptophanes1 have revealed to be attractive molecular receptors2 and they have been used for the design of supramolecular catalysts3 and molecular propellers or gyroscopic systems.4 In particular, the introduction of a vanadium atom in the cavity of hemicryptophanes has for instance been found to yield oxidovanadium-hosts acting as haloperoxidase enzyme mimics catalyzing sulfoxidation reactions.3a
As a general statement, the versatility and modularity of these host compounds have proven particularly suitable to mimic enzymatic systems by combining a lipophilic cavity and a catalytic site.5–9 By this way, original nanoreactors can be synthesized and give rise to artificial metallo-enzymes. Such systems have been already studied in organic solvents.10 Obviously, most biocatalytic processes in nature occur in aqueous media and to obtain a better understanding and control of such biological processes, any synthetic systems should be able to work in water.11 Water solubilisation of biomimetic entities is highly challenging,12 and very few water-soluble metalloenzyme models have been reported in the literature.13
Recently, we have reported several supramolecular systems, based on hemicryptophane ligands, combining a biomimetic copper(II) coordination core and a lipophilic cavity.14 Cu(II) complexes have been found to be efficient catalysts in organic solution for the conversion of cyclohexane to cyclohexanol/cyclohexanone, using H2O2 as the oxygen source. Both the stability and the selectivity of the catalyst have been improved by encapsulating the active site.3d We have also described the first synthesis of a water-soluble hemicryptophane host and its recognition properties toward choline in a basic aqueous medium.15
By combining both water solubility and Cu(II) complexation in a unique cage molecule we expected a water-soluble metallo-enzyme model, presenting a copper site encaged in a closed-shell cavity. Herein, we report the synthesis of the first water-soluble copper@hemicryptophane complex together with its spectroscopic characterization and redox properties.
Results and discussion
Synthesis of the water-soluble complex
Hemicryptophane 1 was synthesized in 6 steps, following a previously published procedure (Scheme S-1†).15 The strongly basic conditions (pH = 12) which are required to solubilize 1 in water are not compatible with its use in biological environments. The substitution of the phenol groups in 1 by carboxylate moieties was performed in order to obtain the hemicryptophane 3, which was found to be soluble in water at physiological pH (pH ≈ 7). The strategy used to synthesize compound 3 is depicted in Scheme 1. The first step involves the reaction of compound 1 with tert-butyl-bromoacetate in DMF in the presence of Cs2CO3 to yield the protected tri-ester derivative 2. Deprotection of both the amine and the ester functions in 2, using trifluoroacetic acid, afforded the water-soluble hemicryptophane 3. Following this synthetic pathway, host 3 has been obtained from commercially available products in 8 steps with an overall yield of 6%.
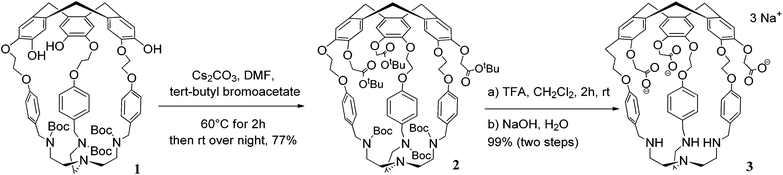 |
| Scheme 1 Synthesis of the water soluble ligand 3. | |
The 1H NMR spectrum of 3 in D2O (pD ≈ 7) indicates that this molecule is on average of C3 symmetry. It displays the expected signals for the cyclotribenzylene unit (Fig. 1): two singlets for the aromatic protons, and the characteristic AB system for the ArCH2 bridges. Two doublets for the aromatic protons of the linkers and the multiplets for the OCH2 groups are also observed in the spectra. The NCH2 protons of the tren unit appear as a complex pattern between 2.0 and 2.25 ppm.
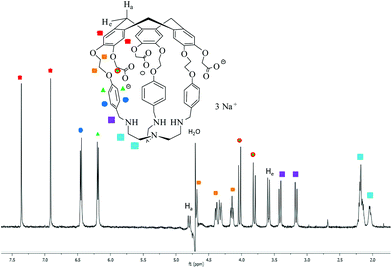 |
| Fig. 1
1H NMR spectrum (500.1 MHz, D2O, 298 K, pD ≈ 7) of hemicryptophane 3. | |
The hemicryptophane copper(II) complex was then synthesized by the reaction of 3 with a stoichiometric amount of copper(II) perchlorate in water to produce Cu(II)@3 isolated in a 50% yield as a pale green solid (Scheme 2).
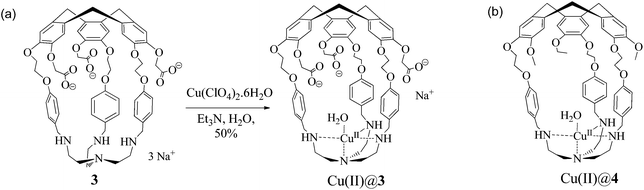 |
| Scheme 2 (a) Synthesis of the water soluble Cu(II)@hemicryptophane 3 complex. (b) Structure of its non-water soluble parent Cu(II)@4. | |
Electronic spectroscopy
The near-IR/vis absorption spectrum of the hemicryptophane complex Cu(II)@3 in CH2Cl2 displays a broad asymmetrical band centred at 850 nm which is a diagnostic signature supporting the trigonal-bipyramidal geometry of the complexed copper(II) ions (Fig. 2). These observations are fully consistent with those previously observed with the parent Cu(II)@4 complex soluble in organic solvents (Scheme 2(b)).14,16
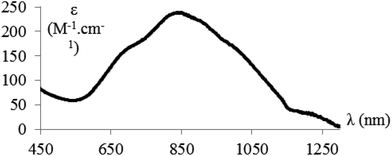 |
| Fig. 2 Near-IR/vis spectrum of Cu(II)@3 (H2O–NaOH, pH ≈ 8, 298 K). | |
EPR measurements
The EPR spectrum of the Cu(II)@3 complex was recorded in frozen water at 70 K (Fig. 3). A pseudo-axial EPR signal (g1 = 2.015, g2 = 2.135 and g3 = 2.220) with a resolved hyperfine structure (A1 = 193 MHz, A2 = 158 MHz and A3 = 363 MHz) was obtained, giving a R value (g2–g1)/(g3–g2) equal to 1.41.17 These values (g1 < 2.04 and R > 1) are typical for a N4X coordination sphere with a distorted geometry close to trigonal-bipyramidal geometry.18 The g-values are very similar to that previously obtained with the Cu(II)@4 complex (g1 = 2.010, g2 = 2.136, g3 = 2.220; R = 1.50),14 indicating that water solubilisation did not induce strong distortion of the initial geometry. Complexes Cu(II)@3 and Cu(II)@4 present a five-coordination sphere with the copper ion bound to the four nitrogen atoms of the tren unit and to one solvent molecule.
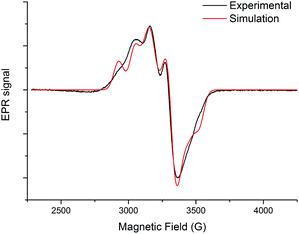 |
| Fig. 3 EPR spectra of Cu(II)@3 in frozen water solution (H2O–NaOH, pH ≈ 8, T = 70 K) (black line: experimental spectra; red line: simulated spectra). Experimental conditions: ν = 9.655 GHz, power = 2 mW and modulation amplitude = 2.0 G. Best fit parameters: g1 = 2.015, g2 = 2.135 and g3 = 2.220, A1 = 193 MHz, A2 = 158 MHz and A3 = 363 MHz for a lwpp = 70 G Gaussian lineshape. | |
Electrochemical studies
The CV curve recorded for Cu(II)@3 in an electrolytic aqueous medium exhibits a fully irreversible reduction wave Epc = −0.59 V attributed to the formation of Cu(I)@3 at the electrode interface (Fig. 4). This irreversible feature, observed at all the investigated scan rates, i.e. from 0.02 to 10 V s−1, is due to the existence of an isomerisation process triggered by the electrochemical reduction of the Cu(II) center. The associated EC process19 can indeed be modelled as a classic square scheme involving changes in the coordination sphere around the Cu(I) center in the pentacoordinated Cu(I)@3 species to produce a more stable tetrahedral Cu(I) complex noted Cu(I)@3′.20 These hypotheses are supported by the observation of an irreversible oxidation wave at Epa = 0.14 V on the reverse scan, the large amplitude of the potential shift seen between Epc and Epa being in agreement with a net decrease of the number of coordinated atoms on the copper center, from 5 in Cu(II)@3 to 4 in Cu(I)@3′. It should be mentioned that a similar EC process was postulated for Cu(II)@4 in dichloromethane; the reduction wave and the associated re-oxidation being observed under these conditions at Epc = −0.91 V/ECS and Epa = 0.45 V/ECS, respectively.14
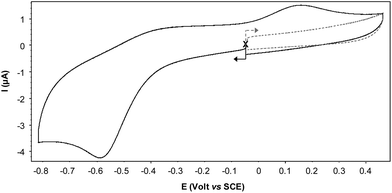 |
| Fig. 4 Cyclic voltammogram of Cu(II)@3 recorded under argon in water (NaNO3 (0.1 M), NaOH (1.5 mM)) (carb., ∅ = 3 mm; ν = 0.05 V s−1). | |
These differences observed between organic and aqueous media highlight the key role of water which is most probably involved both in the solvation and coordination shells of the cupric and cuprous complexes.
Conclusion
In conclusion, the synthesis of the first water soluble copper@hemicryptophane complex has been described. This represents a rare example of water-soluble cage compound trapping a copper ion in the confined space of its cavity. The physico-chemical studies indicated that the solubilisation in water affects only weakly the geometry of the complex, but strongly modifies its redox behavior. This novel water-soluble metallo-enzyme model featuring a copper(II) site encaged in a closed-shell cavity opens up the way to new bio-inspired catalytic systems.
Experimental section
General methods
All reactions were carried out under argon by means of an inert gas/vacuum double manifold and standard Schlenk techniques. Dichloromethane was first dried over molecular sieves and then passed through an activated alumina column followed by an argon flush on a solvent station. 1H and 13C NMR spectra were recorded at 500.10 MHz and 125.76 MHz, respectively. Chemical shifts are reported relative to the residual protonated solvent signal (CDCl3), or relative to residual ethanol for the spectra in D2O. Mass spectra were recorded by the Centre de Spectrométrie de Masse, Institute of Chemistry, Lyon. Compound 1 was prepared according to the published procedure.15 EPR spectra were obtained from a Bruker EMX spectrometer and fitted with Easyspin21 software.
Cyclic voltammetry (CV) data were recorded using an ESP-300 Biologic potentiostat equipped with a 1 A/48 V booster and an analog linear scan generator. All the experiments were conducted under an argon atmosphere in a standard one-compartment, three-electrode electrochemical cell placed in a faraday cage. An automatic ohmic drop compensation procedure was systematically implemented prior to recording CV data. All the electrodes were purchased from ALS Co. Ltd. Vitreous carbon (Φ = 3 mm) working electrodes were polished with 1 mm diamond paste before each recording. A saturated ECS electrode was used as a reference. HPLC grade water + sodium nitrate (0.1 M) was used as the electrolyte.
Syntheses
Hemicryptophane 2.
Hemicryptophane 1 (257 mg, 0.212 mmol) was dissolved in anhydrous DMF (20 mL) and Cs2CO3 (276 mg, 0.848 mmol) was added to the solution which was stirred at 60 °C for 15 minutes. Then tert-butylbromoacetate (0.141 mL, 0.945 mmol) was added and the mixture was stirred at 60 °C for 2 hours, then at room temperature overnight. The solvent was removed and CHCl3 (20 mL) and H2O (20 mL) were added to the residue. The two layers were separated and the aqueous phase was extracted with CHCl3 (20 mL). The combined organic solutions were washed with H2O (2 × 20 mL) and dried over anhydrous Na2SO4. The solvent was evaporated and the crude product was purified by column chromatography on silica gel, using CH2Cl2 and a gradient of EtOAc (10% to 50%) as eluents. Compound 2 was obtained as a foamy white solid (252 mg, 77%). ESI-MS m/z observed 1551.7991 ([M + H]+, calculated 1551.7991 for C87H115N4O21). 1H NMR (CDCl3, 298 K, 500.10 MHz): δ 7.13–6.91 (br, 6H, ArH), 6.91–6.82 (br, 6H, ArH), 6.82–6.66 (br, 6H, ArH), 4.65 (d, 3H, J = 13.7 Hz, ArCH2Ar), 4.56 (d, 3H, J = 16.0 Hz, CH2COO) 4.48–4.05 (m, 21H, CH2COO; O(CH2)2O; ArCH2N), 3.47 (d, 3H, J = 13.7 Hz, ArCH2Ar), 3.07–2.60 (br, 6H, N(CH2)2N), 2.50–2.15 (br, 6H, N(CH2)2N), 1.51–1.32 (br, 54H, C(CH3)3 BOC and tBu-ester). 13C NMR (CDCl3, 298 K, 125.76 MHz): δ 168.59 (CH2C(O)O) 157.97, 155.92 and 155.36 (NC(O)O), 147.49 (CAr), 147.30 (CAr), 134.00 (CAr), 133.08 (CAr), 130.99 (CAr), 129.42 (CAr), 128.74 (CAr), 118.77 (CAr), 117.53 (CAr), 115.19 (CAr), 82.06 (OC (CH3)3), 79.70 (OC(CH3)3), 68.49 and 68.25 (OCH2), 66.77 (OCH2), 52.92 (N(CH2)2N), 50.45 and 49.78 (NCH2Ar), 45.12 (N(CH2)2N), 36.52 (ArCH2Ar), 28.67 (CH3), 28.26 (CH3). Mp: 229.185 °C. IR ν = 2974, 2918, 1749, 1686, 1612, 1509, 1456, 1408, 1365, 1246, 1143 cm−1.
Hemicryptophane 3.
Trifluoroacetic acid (TFA, 780 μL, 10 mmol) was added to a solution of hemicryptophane 2 (120 mg, 77 μmol) in CH2Cl2 (2 mL). The mixture was stirred for 2 h at room temperature and the solvent and TFA were removed under vacuum. Several additions and evaporations of CHCl3 were carried out to facilitate the evaporation of TFA. Distilled water (1.5 mL) and NaOH (19.6 mg, 490 μmol) were added to the residue and the mixture was stirred for 30 minutes. Water was then evaporated to give 3 as a yellow solid (quantitative). ESI-MS m/z observed 1081.4500 ([M + 2H]−, 1081.4452 calculated for C60H65N4O15). 1H NMR (D2O, 298 K, 500.10 MHz): δ 7.36 (s, 3H, ArH), 6.91 (s, 3H, ArH), 6.45 (d, 6H, J = 8.4 Hz, ArH), 6.19 (d, 6H, J = 8.4 Hz, ArH), 4.80 (d, 3H, J = 13.8 Hz, ArCH2Ar), 4.69–4.65 (m, 3H, O(CH2)2O), 4.42–4.36 (m, 3H, O(CH2)2O), 4.36–4.28 (m, 3H, O(CH2)2O), 4.19–4.10 (m, 3H, O(CH2)2O), 4.03 (d, 3H, J = 15.4 Hz, OCH2COO), 3.80 (d, 3H, J = 15.4 Hz, OCH2COO), 3.59 (d, 3H, J = 13.8 Hz, ArCH2Ar), 3.41 (d, 3H, J = 13.6 Hz, ArCH2N), 3.17 (d, 3H, J = 13.6 Hz, ArCH2N), 2.25–2.00 (m, 12H, N(CH2)2N). 13C NMR (D2O, 298 K, 125.76 MHz): δ 176.07 ((C
O)O), 157.68 (CArO), 145.85 (CArO), 145.47 (CArO), 133.41 (CAr), 132.79 (CAr), 130.00 (CArH), 129.27 (CAr), 116.38 (CArH), 115.82 (CArH), 114.19 (CArH), 69.12 (O(CH2)2O), 66.71 (OCH2C(O)O), 66.32 (O(CH2)2O), 52.92 (N(CH2)2N), 50.51 (ArCH2N), 44.79 (N(CH2)2N), 35.26 (ArCH2Ar). Mp: 219.3 °C. IR ν = 3400, 2923, 2856, 1681, 1605, 1509, 1425, 1335, 1259, 1204, 1176, 1127 cm−1.
Complex Cu(II)@hemicryptophane 3.
To a solution of hemicryptophane 3 (15.6 mg, 14 μmol) in milli-Q water (3 mL) were added Cu(ClO4)2·6H2O (5.1 mg, 14 μmol) and Et3N (9 μL, 67 μmol). The solution was stirred for 5 minutes and water and Et3N were removed under vacuum. Milli-Q water (1.5 mL) was added and the blue solid was triturated in this solvent. The solution was centrifuged and the supernatant was removed. This last step was repeated with 1 mL of milli-Q water and the residue was dried under vacuum. Pale green solid was obtained (7.9 mg, 50%). ESI-MS m/z observed 1142.3646 ([M]−, calculated 1142.3591 for C60H63CuN4O15). EPR (H2O–NaOH; pH = 8; 70 K) g1 = 2.015 (A1 = 193 MHz); g2 = 2.135 (A2 = 158 MHz); g3 = 2.220 (A3 = 363 MHz). Vis-near IR spectroscopy (H2O, 298 K) λmax = 850 nm, ε = 237 M−1 cm−1, shoulder band around 700 nm, ε = 165 M−1 cm−1. Cyclic voltammetry: Epc = −0.34 V and Epa = 0.30 V vs. E1/2 [FcMeOH0/+] (H2O, NaOH (1.5 mM), 12-CuII (5 × 10−4 M), NaNO3 (0.1 M), working electrode: carbon, 50 mV s−1).
Notes and references
- J. Canceill, A. Collet, J. Gabard, F. Kotzyba-Hibert and J.-M. Lehn, Helv. Chim. Acta, 1982, 65, 1894–1897 CrossRef CAS
.
-
(a) S. Le Gac and I. Jabin, Chem. – Eur. J., 2008, 14, 548–557 CrossRef CAS PubMed
;
(b) O. Perraud, V. Robert, A. Martinez and J.-P. Dutasta, Chem. – Eur. J., 2011, 17, 4177–4182 CrossRef CAS PubMed
;
(c) L. Wang, G.-T. Wang, X. Zhao, X.-K. Jiang and Z.-T. Li, J. Org. Chem., 2011, 76, 3531–3535 CrossRef CAS PubMed
;
(d) O. Perraud, A. Martinez and J.-P. Dutasta, Chem. Commun., 2011, 47, 5861–5863 RSC
;
(e) O. Perraud, V. Robert, A. Martinez and J.-P. Dutasta, Chem. – Eur. J., 2011, 17, 13405–13408 CrossRef CAS PubMed
;
(f) O. Perraud, V. Robert, H. Gornitzka, A. Martinez and J.-P. Dutasta, Angew. Chem., Int. Ed., 2012, 51, 504–508 CrossRef CAS PubMed
.
-
(a) A. Martinez and J.-P. Dutasta, J. Catal., 2009, 267, 188–192 CrossRef CAS PubMed
;
(b) Y. Makita, K. Sugimoto, K. Furuyosho, K. Ikeda, S. I. Jujiwara, T. Shin-ike and A. Ogawa, Inorg. Chem., 2010, 49, 7220–7222 CrossRef CAS PubMed
;
(c) Y. Makita, K. Ikeda, K. Sugimoto, T. Fujita, T. Danno, K. Bobuatong, M. Ehara, S. I. Jujiwara and A. Ogawa, J. Organomet. Chem., 2012, 26, 706–707 Search PubMed
;
(d) O. Perraud, A. B. Sorokin, J.-P. Dutasta and A. Martinez, Chem. Commun., 2013, 49, 1288–1290 RSC
.
-
(a) A. Martinez, V. Robert, H. Gornitzka and J.-P. Dutasta, Chem. – Eur. J., 2010, 16, 520–527 CrossRef CAS PubMed
;
(b) A. Martinez, L. Guy and J.-P. Dutasta, J. Am. Chem. Soc., 2010, 132, 16733–16734 CrossRef CAS PubMed
;
(c) N. S. Khan, J. M. Perez-Aguilar, T. Kaufmann, P. A. Hill, O. Taratula, O.-S. Lee, P. J. Carroll, J. G. Saven and I. J. Dmochowski, J. Org. Chem., 2011, 76, 1418–1424 CrossRef CAS PubMed
.
- M. Raynal, P. Ballester, A. Visal-Ferran and P. W. N. M. Van Leeuwen, Chem. Rev., 2014, 43, 1660–1733 RSC
.
- M. Raynal, P. Ballester, A. Visal-Ferran and P. W. N. M. Van Leeuwen, Chem. Rev., 2014, 43, 1734–1787 RSC
.
- A. J. Kirby, Angew. Chem., Int. Ed. Engl., 1996, 35, 707–724 CrossRef CAS
.
- J. K. M. Sanders, Chem. – Eur. J., 1998, 4, 1378–1383 CrossRef CAS
.
- J.-M. Lehn, Rep. Prog. Phys., 2004, 67, 245–249 CrossRef
.
-
J. W. Steed and J. L. Atwood, in Supramolecular Chemistry, Wiley-VCH, Weinheim, 2nd edn, 2009 Search PubMed
.
- G. V. Oshovky, D. N. Reinhoudt and W. Verboom, Angew. Chem., Int. Ed., 2007, 46, 2366–2393 CrossRef PubMed
.
- E. Klein, Y. Ferrand, N. P. Barwell and A. P. Davis, Angew. Chem., Int. Ed., 2008, 47, 2693–2696 CrossRef CAS PubMed
.
-
(a) R. Breslow, Acc. Chem. Res., 1995, 28, 146 CrossRef CAS
;
(b) E. Engeldinger, D. Armspach and D. Matt, Chem. Rev., 2003, 103, 4147 CrossRef CAS PubMed
;
(c) D. M. Homden and C. Redshaw, Chem. Rev., 2008, 108, 5086 CrossRef CAS PubMed
;
(d) G. Thiabaud, A. Brugnara, M. Carboni, N. Le Poul, B. Colasson, Y. Le Mest and O. Reinaud, Org. Lett., 2012, 14, 2500–2503 CrossRef CAS PubMed
.
- O. Perraud, J.-B. Tommassino, V. Robert, L. Khrouz, B. Albela, L. Bonneviot, J.-P. Dutasta and A. Martinez, Dalton Trans., 2013, 42, 1530–1535 RSC
.
- A. Schmitt, V. Robert, J.-P. Dutasta and A. Martinez, Org. Lett., 2014, 16, 2374–2377 CrossRef CAS PubMed
.
-
(a) M. Duggan, N. Ray, B. Hathaway, G. Tomlinson, P. Brint and K. J. Pelin, J. Chem. Soc., Dalton Trans., 1980, 8, 1342–1348 RSC
;
(b) F. Thaler, C. D. Hubbard, F. W. Heinemann, R. Van Eldik, S. Schindler, I. Fabian, A. M. Dittler-Klingemann, F. Ekkehardt Hahn and C. Orvig, Inorg. Chem., 1998, 37, 4022–4029 CrossRef CAS PubMed
.
- D. E. Billing, R. J. Dudley, B. J. Hathaway and A. Tomlinso, J. Chem. Soc. A, 1971, 691–696 RSC
.
- R. J. Dudley, B. J. Hathaway, P. G. Hodgson, P. C. Power and D. J. Loose, J. Chem. Soc., Dalton Trans., 1974, 1005–1009 RSC
.
-
(a) C. Bucher, E. Duval, J.-M. Barbe, J.-N. Verpeaux, C. Amatore and R. Guilard, C. R. Acad. Sci., Ser. IIc: Chim., 2000, 3, 211–222 CrossRef CAS
;
(b) C. Amatore, J.-M. Barbe, C. Bucher, E. Duval, R. Guilard and J.-N. Verpeaux, Inorg. Chim. Acta, 2003, 356, 267–278 CrossRef CAS
;
(c) C. Bucher, J.-C. Moutet, J. Pecaut, G. Royal, E. Saint-Aman, F. Thomas, S. Torelli and M. Ungureanu, Inorg. Chem., 2003, 42, 2242–2252 CrossRef CAS PubMed
;
(d) C. Bucher, J.-C. Moutet, J. Pecaut, G. Royal, E. Saint-Aman and F. Thomas, Inorg. Chem., 2004, 43, 3777–3779 CrossRef CAS PubMed
;
(e) U. Darbost, V. Penin, E. Jeanneau, C. Félix, F. Vocanson, C. Bucher, G. Royal and I. Bonnamour, Chem. Commun., 2009, 6774–6776 RSC
.
- The driving force of most reorganization reactions reported so far in the literature for electrogenerated Cu(I) complexes is the stabilization of the Cu(I) ion in a tetrahedral environment: see for instance ref. 18 or J.-P. Sauvage, J.-P. Collin, S. Durot, J. Frey, V. Heitz, A. Sour and C. Tock, C. R. Chim., 2010, 13, 315–328 CrossRef CAS PubMed
.
- S. Stoll and A. Schweiger, J. Magn. Reson., 2006, 178, 42–55 CrossRef CAS PubMed
.
Footnote |
† Electronic supplementary information (ESI) available: Synthesis of 1, 1H and 13C NMR spectra of compounds 2 and 3. See DOI: 10.1039/c4ob02085e |
|
This journal is © The Royal Society of Chemistry 2015 |
Click here to see how this site uses Cookies. View our privacy policy here.