Selective detection of Al3+ and citric acid with a fluorescent amphiphile†
Received
1st October 2014
, Accepted 6th November 2014
First published on 12th November 2014
Abstract
An amphiphilic fluorescent dye with a disulfonated BODIPY head group and a heptadecyl side chain is described. In buffered aqueous solution, the amphiphile can form aggregates with a critical micelle concentration of ∼20 μM. The aggregation of the dye is associated with a strong quenching of its fluorescence. Al3+ promotes aggregation, whereas other metal ions have a much smaller effect, in particular when histidine is added as masking agent. The Al3+-induced aggregation can be used to sense Al3+ in the low micromolar concentration range with high selectivity. Furthermore, we demonstrate that a dye–Al3+ mixture can be used as a sensing ensemble for the detection of citric acid. The assay allows quantifying the citric acid content of commercial beverages such as energy drinks.
Introduction
The analyte-induced aggregation of photoluminescent molecules has been used extensively for sensing purposes. Recently published examples include optical sensors for the detection of pyrophosphate,1 biogenic amines,2 oxalic acid,3 DNA,4 Hg2+,5 K+,6 ATP,7 heparin,8 pH,9 glucose,10,11 and Mg2+.12 Luminescent polymers1–5 have often been used in this context, but other types of compounds such as metal complexes,7b–9 fluorescent amphiphiles,7a,10 quantum dots,11 and polypyridyl ligands12 have been employed as well. Conceptually, this sensing approach is complementary to the analyte-induced disassembly of receptor-dye aggregates, commonly referred to as indicator displacement assays (IDAs).13 We have recently shown that amphiphiles with polysulfonated fluorescent head groups can be employed as molecular probes for the detection of spermine14 and aminoglycosides.15 In both cases, the polycationic analytes are assumed to undergo a multivalent interaction16 with the anionic amphiphile, thereby facilitation micellation. This process is associated with a change of the optical properties of the fluorescent head group, thereby allowing the detection of the analyte (Scheme 1).
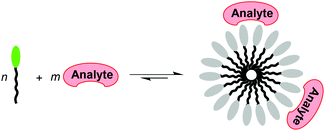 |
| Scheme 1 The analyte-induced aggregation of amphiphiles can be used for sensing purposes if aggregation induces a change in the optical properties of the amphiphile. | |
We hypothesized that a similar approach could be used for the detection of Al3+ ions. Sensing of Al3+ is of interest because of its pharmacological effects. At high doses, Al3+ can be neurotoxic.17 Furthermore, the accumulation of Al3+ in the human body has been associated with Alzheimer's disease.18 Given its biological relevance, it is not surprising that numerous optical probes for Al3+ have been reported.19–22 However, these sensing systems often require substantial amounts of organic co-solvents21,22b,c or they suffer from interference from other metal ions.20a,d,f So far, there are few studies about the detection of Al3+ by analyte-induced aggregation of fluorophores.22 Below, we show that an amphiphilic dye with a disulfonated BODIPY head group and a heptadecane side chain can be used to sense low micromolar concentrations of Al3+ in buffered aqueous solution with high selectivity. Furthermore, we show that an amphiphile–Al3+ mixture can be used as a sensing ensemble for the detection of citric acid.23
Results and discussion
For our studies, we synthesized the amphiphilic dyes 3 and 4 containing a disulfonated BODIPY head group and alkyl side chains of different lengths (3: undecyl; 4: heptadecyl). The dyes were obtained by sulfonation of the easily accessible precursors 1 and 2 with chlorosulfonic acid in analogy to a known procedure (Scheme 2).24 The sulfonated BODIPY was chosen as fluorescent head group because of the high quantum yield of this fluorophore. Furthermore, we expected an emission maximum of higher than 500 nm, which would be well suited for sensing applications because of reduced interference from background fluorescence.25
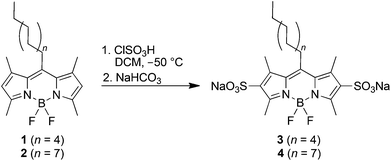 |
| Scheme 2 Synthesis of the fluorescent dyes 3 and 4. | |
Both amphiphiles were characterized by NMR spectroscopy and mass spectrometry. The aggregation of the dyes in buffered aqueous solution (10 mM MOPS buffer, pH 7.0) was investigated by concentration-dependent fluorescence spectroscopy. For dye 4, we observed a shift of the fluorescence emission maximum from 504 to 534 nm (λex. = 490 nm) upon increasing the concentration from 0.21 to 105 μM (Fig. 1, top). A critical micelle concentration (cmc) of ∼20 μM was determined by linear extrapolation of the relative fluorescence emission intensity at 534 and 505 nm (Fig. 1, bottom).
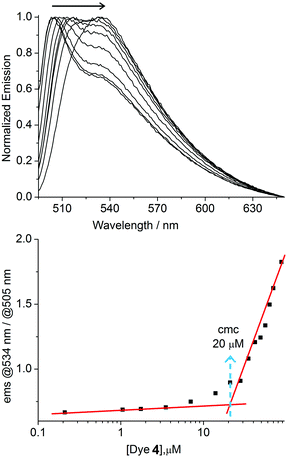 |
| Fig. 1 Top: normalized fluorescence emission spectra (λex = 490 nm) of buffered aqueous solutions (10 mM MOPS, pH 7.0) containing different amounts of dye 4 (0.21–105 μM). Bottom: relative fluorescence intensity at 534 and 505 nm of the same solutions. | |
Similar experiments were performed with dye 3 having a shorter undecyl side chain. No evidence for aggregation was observed in the concentration range between 1 μM and 1 mM. The formation of micellar aggregates by dye 4 at concentrations above 20 μM was substantiated by dynamic light scattering (DLS) experiments. At a concentration of [4] = 50 μM, we were able to observe aggregates with an average hydrodynamic diameter of ∼13 nm (see ESI†).
We hypothesized that metal cations could induce the aggregation of 4. Therefore, we have measured the fluorescence spectra of solutions containing dye 4 in the presence of different metal salts ([Mn+] = 60 μM; stock solutions in MeOH). For these studies, a dye concentration of [4] = 4.0 μM was chosen. This value is slightly below the cmc of the amphiphile. Most metal salts had a very small effect on the fluorescence emission. For CuCl2 and for AlCl3, however, substantial fluorescence quenching was observed (Fig. 2, top). The most pronounced change was found for AlCl3, the addition of which resulted in nearly complete quenching of the fluorescence.
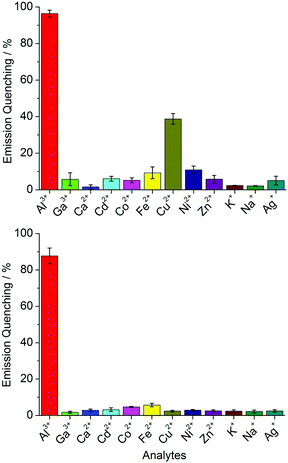 |
| Fig. 2 Top: fluorescence emission quenching (λex = 490 nm; λem = 505 nm) of buffered aqueous solutions (10 mM MOPS, pH 7.0, H2O with 0.6 vol% MeOH) of dye 4 (4.0 μM) in the presence different metal cations (60 μM). Bottom: measurements in the presence of the masking agent histidine (5.0 mM). The values are averages of three independent measurements. | |
Control experiments with dye 3 support the hypothesis of analyte-induced aggregation. Only minor fluorescence quenching was observed with Al3+ (see ESI, Fig. S8†), indicating that a simple complexation between the BODIPY head group and Al3+ is not responsible for the optical changes observed for 4. Experiments with the solvatochromic probe Nile Red are in line with these results. When Al3+ was added to solutions containing dye 4 (4.0 μM) and Nile Red (6.0 μM), an increased fluorescence at 660 nm was observed (see ESI, Fig. S4†). This increase can be attributed to the encapsulation of Nile Red in a hydrophobic domain.26 Because of the low concentration of dye 4 under sensing conditions, we were not able to confirm aggregation by DLS.
In order to enhance the selectivity for Al3+, we explored different masking agents. The amino acid histidine, a known chelate ligand for transition metal ions,27 was found to give good results. In the presence of 5.0 mM histidine, none of the metal ions gave a significant fluorescence change apart from Al3+ (Fig. 2, bottom). For the latter, an emission quenching of nearly 90% was observed.
Fluorescence titration experiments with solutions of 4 and different amounts of AlCl3, CuCl2, ZnCl2, NiCl2, and Cd(NO3)2 (0–135 μM) showed that it is possible to selectively sense low micromolar concentrations of Al3+ with a detection limit of approximately 3 μM (3σo) (Fig. 3). The good selectivity was further confirmed by measuring the fluorescence of solutions containing dye 4 (4.0 μM), histidine (5.0 mM), AlCl3 (20 μM) and an additional metal salt (20 μM). In all cases a fluorescence quenching of around 40% was observed (ESI, Fig. S9†).
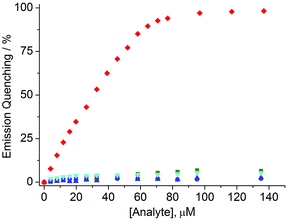 |
| Fig. 3 Fluorescence emission quenching (λex = 490 nm; λem = 505 nm) of buffered aqueous solutions (10 mM MOPS, pH 7.0, H2O with 0–1.3 vol% MeOH) containing dye 4 (4.0 μM), histidine (5.0 mM), and different amounts of Al3+ (red symbols), Cd2+ (cyan symbols), Cu2+ (olive symbols), Ni2+ (blue symbols), or Zn2+ (violet symbols). The data points are averages of three independent measurements. The errors are less than 4%. | |
Citric acid is known to bind Al3+ with high affinity and selectivity.20c,28 Therefore, it seemed possible to use citric acid for the disassembly of dye 4–Al3+ aggregates. This is indeed the case. When citric acid was added to a buffered aqueous solution containing dye 4 (4.0 μM) and AlCl3 (120 μM), an increased fluorescence emission at 505 nm was observed (Fig. 4), suggesting the formation of monomeric 4. It is thus possible to use a mixture of 4 and Al3+ as a sensing ensemble for the detection of citric acid via a turn-on fluorescence signal.29 The titration data depicted in Fig. 4 could be used to sense citric acid in the low micromolar concentration range with a detection limit of approximately 5 μM (3σo).
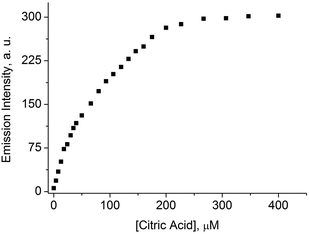 |
| Fig. 4 Fluorescence emission intensity (λex = 490 nm; λem = 505 nm) of buffered aqueous solutions (10 mM MOPS, pH 7.0, H2O with 1.2 vol% MeOH) containing dye 4 (4.0 μM), Al3+ (120 μM), and different amounts of citric acid (0–400 μM). The data points are averages of three independent measurements. The errors are less than 4%. | |
The selectivity of this assay turned out to be very good. Several biological relevant carboxylic acids were tested (400 μM), most of which gave a negligible optical response (Fig. 5). Only tartaric acid resulted in a fluorescence signal, but its intensity was only 1/3 of that of citric acid. We have also tested the influence of glucose, fructose, or sucrose (400 μM in each case) on the sensing system. These carbohydrates gave a negligible fluorescence response.
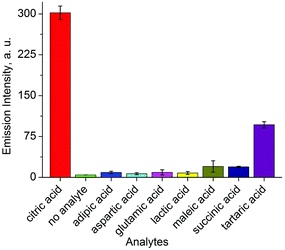 |
| Fig. 5 Fluorescence emission intensity (λex = 490 nm; λem = 505 nm) of buffered aqueous solutions (10 mM MOPS, pH 7.0, H2O with 1.2 vol% MeOH) of dye 4 (4.0 μM) and Al3+ (120 μM) in the presence different analytes (400 μM). The values are averages of three independent measurements. | |
The good selectivity and sensitivity of our citric acid assay prompted us to examine the possibility to detect and quantify citric acid in commercial beverages. Three energy drinks, two soft drinks, and one mineral water were chosen as representative samples. First, we have determined the content of citric acid in these samples by 1H NMR spectroscopy. This analytical technique is well suited for such an analysis because the signals of the CH2 group of citric acid are well separated in the spectra, allowing for a reasonably precise integration (see ESI†). We then determined the citric acid concentration of the samples using a mixture of dye 4 and AlCl3 as a sensing ensemble. The fluorescence signal was converted into a concentration value by using the calibration curve depicted in Fig. 4. As shown in Fig. 6, the match between the values obtained by NMR and by fluorescence spectroscopy is remarkably good.
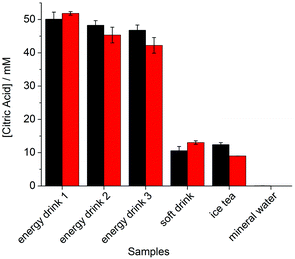 |
| Fig. 6 Concentration of citric acid in drinks as determined by 1H NMR spectroscopy (black bars) and by the dye 4–Al3+ sensing ensemble (red bars). Experimental details are given in the ESI.† | |
Conclusions
The amphiphilic fluorescent dye 4 with a disulfonated BODIPY head group and a heptadecyl side chain can be used to sense Al3+ in the low micromolar concentration range with high selectivity. The optical response is due to analyte-induced aggregation of the dye. From an application point of view, it is noteworthy that the assay can be performed in aqueous solution at neutral pH without the need of large amounts of organic co-solvents. Citric acid, a known chelator for Al3+, can reverse the aggregation of 4. It is thus possible to use a mixture of 4 and Al3+ as a turn-on fluorescence sensor for citric acid. As proof of concept, we have shown that it is possible to detect the citric acid concentration in commercial beverages. Overall, our results provide further evidence for the utility of fluorescent amphiphiles in supramolecular analytical chemistry.
Experimental section
General
All chemicals and solvents were purchased from standard suppliers and used without further purification. MOPS buffer (10 mM MOPS buffer, pH 7.0) was prepared by dissolving 3-(N-morpholino) propanesulfonic acid in bidistilled water. HCl and NaOH solutions were used to adjust the pH of the buffer. 1H and 13C NMR spectra were recorded on Bruker Advance DPX 400 and 800 instruments at 25 °C. Multiplicities of the 1H NMR signals are assigned as following: s (singlet), d (doublet), t (triplet), m (multiplet). DLS measurements were performed with a Zetasizer nano ZS90 (Malvern) instrument. High resolution mass spectra were recorded with a Waters Q-TOF Ultima (ESI-TOF) instrument. The dyes 1 and 2 were prepared in analogy to a known procedure (see ESI†).24
Synthesis of dye 3
A solution of chlorosulfonic acid (49.8 μL, 0.75 mmol) in CH2Cl2 (5 mL) was added dropwise over 20 min under stirring to a cooled (−50 °C) solution of compound 1 (100 mg, 0.25 mmol) in CH2Cl2 (30 mL). The ice bath was then removed and the stirred mixture was warmed to RT, resulting in the formation of a red precipitate. The precipitate was isolated by filtration, washed with CH2Cl2, and redissolved in aqueous bicarbonate solution (10 mL, 40 mM). The solution was dried under vacuum. Purification by column chromatography (SiO2; eluent: CHCl3–MeOH–H2O; 7
:
3
:
0.5) gave 3 as a red solid (61 mg, 87 μmol, 35%). 1H NMR (400 MHz, CD3OD): δ = 0.80 (t, J = 7.0 Hz, 3 H, CH3), 1.15–1.35 (m, 14 H, CH2), 1.46 (p, J = 8.0 Hz, 2 H, CH2), 1.55–1.64 (m, 2 H, CH2), 2.65 (s, 6 H, CH3), 2.69 (s, 6 H, CH3), 3.09–3.13 (m, 2 H, CH2). 13C NMR (100 MHz, CD3OD): δ = 13.0, 13.03, 13.39, 22.32, 28.20, 29.06, 29.29, 29.31, 29.84, 31.55, 31.65, 130.57, 134.34, 139.61, 150.99, 153.49. ESI-MS calcd for C24H35BF2N2O6S2 [(M − 2Na)−2] m/z = 280.1001 found 280.1006.
Synthesis of dye 4
A solution of chlorosulfonic acid (39.9 μL, 0.60 mmol) in CH2Cl2 (5 mL) was added dropwise over 20 min under stirring to a cooled (−50 °C) solution of compound 2 (100 mg, 0.20 mmol) in CH2Cl2 (30 mL). The ice bath was then removed and the stirred mixture was warmed to RT, resulting in the formation of a red precipitate. The precipitate was isolated by filtration, washed with CH2Cl2, and redissolved in aqueous bicarbonate solution (10 mL, 40 mM). The solution was dried under vacuum. Purification by column chromatography (SiO2; eluent: CHCl3–MeOH–H2O; 7
:
3
:
0.5) gave 4 as a red solid (20.7 mg, 30 μmol, 15%). 1H NMR (800 MHz, CD3OD): δ = 0.80 (t, J = 7.0 Hz, 3 H, CH3), 1.15–1.35 (m, 26 H, CH2), 1.46 (p, J = 8.0 Hz, 2 H, CH2), 1.55–1.61 (m, 2 H, CH2), 2.65 (s, 6 H, CH3), 2.69 (s, 6 H, CH3), 3.10–3.12 (m, 2 H, CH2). 13C NMR (200 MHz, CD3OD): δ = 13.0, 13.07, 13.39, 22.36, 28.21, 29.10, 29.27, 29.35, 29.38, 29.41, 29.90, 31.56, 31.69, 130.57, 134.32, 139.62, 151.02, 153.47. ESI-MS calcd for C30H47BF2N2O6S2 [(M − 2Na)−2] m/z = 322.1471 found 322.1469.
Fluorescence measurements
Stock solutions of dye 3 (1.0 mM) and dye 4 (105 μM) were prepared in MOPS buffer (10 mM, pH 7.0) and stock solutions of the metal salts (NiCl2, ZnCl2, AlCl3, CuCl2, Cd(NO3)2: 2 mM; NiCl2, ZnCl2, AlCl3, CuCl2, CaCl2, KCl, NaCl, AgCl, Ga(acac)3, Cd(NO3)2, Fe(ClO4)2, Co(C2H3O2)2: 10 mM) were prepared in methanol. Stock solutions of histidine (100 mM) and carboxylic acid analytes (citric acid: 20 mM; citric acid, adipic acid, aspartic acid, glutamic acid, lactic acid, maleic acid, succinic acid, tartaric acid: 100 mM) were prepared in bidistilled water. The samples were prepared by mixing aliquots of the corresponding stock solutions with MOPS buffer in quartz cuvettes. The final volume of all samples was 1.5 mL. The fluorescent signal was measured 3 minutes after sample preparation. A Varian Cary Eclipse fluorescence spectrophotometer was employed for these measurements.
Acknowledgements
This work was supported by funding from the Swiss National Science Foundation, the Ecole Polytechnique Fédérale de Lausanne (EPFL), and the Marie Curie Initial Training Network ReAD (Z.K.).
Notes and references
- X. Zhao and K. S. Schanze, Chem. Commun., 2010, 46, 6075 RSC.
- A. Satrijo and T. M. Swager, J. Am. Chem. Soc., 2007, 129, 16020 CrossRef CAS PubMed.
- H. Sun, F. Feng, M. Yu and S. Wang, Macromol. Rapid Commun., 2007, 28, 1905 CrossRef CAS.
- J. W. Hong, W. L. Hemme, G. E. Keller, M. T. Rinke and G. C. Bazan, Adv. Mater., 2006, 18, 878 CrossRef CAS.
- Y. Tang, F. He, M. Yu, F. Feng, L. An, H. Sun, S. Wang, Y. Li and D. Zhu, Macromol. Rapid Commun., 2006, 27, 389 CrossRef CAS.
- J. Kim, D. T. McQuade, S. K. McHugh and T. M. Swager, Angew. Chem., Int. Ed., 2000, 39, 3868 CrossRef CAS.
-
(a) X. Li, X. Guo, L. Cao, Z. Xun, S. Wang, S. Li, Y. Li and G. Yang, Angew. Chem., Int. Ed., 2014, 53, 7809 CrossRef CAS PubMed;
(b) M. C.-L. Yeung and V. W.-W. Yam, Chem. Sci., 2013, 4, 2928 RSC.
- M. C.-L. Yeung and V. W.-W. Yam, Chem. – Eur. J., 2011, 17, 11987 CrossRef CAS PubMed.
- C. Y.-S. Chung, S. P.-Y. Li, M.-W. Louie, K. K.-W. Lo and V. W.-W. Yam, Chem. Sci., 2013, 4, 2453 RSC.
- Y.-J. Huang, W.-J. Ouyang, X. Wu, J. S. Fossey, T. D. James and Y.-B. Jiang, J. Am. Chem. Soc., 2013, 135, 1700 CrossRef CAS PubMed.
- L. Zhang, Z.-Y. Zhang, R.-P. Liang, Y.-H. Li and J.-D. Qiu, Anal. Chem., 2014, 86, 4423 CrossRef CAS PubMed.
- A. Fermi, G. Bergamini, M. Roy, M. Gingras and P. Ceroni, J. Am. Chem. Soc., 2014, 136, 6395 CrossRef CAS PubMed.
-
(a) G. Ghale and W. M. Nau, Acc. Chem. Res., 2014, 47, 2150 CrossRef CAS PubMed;
(b) P. Wu, C. Xu and X. Hou, Appl. Spectrosc. Rev., 2013, 48, 629 CrossRef;
(c) X. Lou, D. Ou, Q. Li and Z. Li, Chem. Commun., 2012, 48, 8462 RSC;
(d) K. Severin, Curr. Opin. Chem. Biol., 2010, 14, 737 CrossRef CAS PubMed;
(e) B. T. Nguyen and E. V. Anslyn, Coord. Chem. Rev., 2006, 250, 3118 CrossRef CAS PubMed.
- Z. Köstereli and K. Severin, Chem. Commun., 2012, 48, 5841 RSC.
- Z. Köstereli, R. Scopelliti and K. Severin, Chem. Sci., 2014, 5, 2456 RSC.
- A. Barnard and D. K. Smith, Angew. Chem., Int. Ed., 2012, 51, 6572 CrossRef CAS PubMed.
-
(a) S. C. Bondy, Neurotoxicology, 2010, 31, 575 CrossRef CAS PubMed;
(b) V. Kumar and K. D. Gill, Arch. Toxicol., 2009, 83, 965 CrossRef CAS PubMed.
-
(a) J. R. Walton, J. Alzheimer's Dis., 2013, 35, 7 CAS;
(b) L. Tomljenovic, J. Alzheimer's Dis., 2011, 23, 567 CAS;
(c) V. Frisardi, V. Solfrizzi, C. Capurso, P. G. Kehoe, B. P. Imbimbo, A. Santamato, F. Dellegrazie, D. Seripa, A. Pilotto, A. Capurso and F. Panza, J. Alzheimer's Dis., 2010, 20, 17 CAS;
(d) A. C. Miu and O. Benga, J. Alzheimer's Dis., 2006, 10, 179 CAS.
- Review article: S. Das, M. Dutta and D. Das, Anal. Methods, 2013, 5, 6262 RSC.
- For recent examples of fluorogenic Al3+ detection in water with less than 2% of co-solvents see:
(a) S. Kim, J. Y. Noh, S. J. Park, Y. J. Na, I. H. Hwang, J. Min, C. Kim and J. Kim, RSC Adv., 2014, 4, 18094 RSC;
(b) T.-Y. Zhou, L.-P. Lin, M.-C. Rong, Y.-Q. Jiang and X. Chen, Anal. Chem., 2013, 85, 9839 CrossRef CAS PubMed;
(c) H. Wang, F. He, R. Yan, X. Wang, X. Zhu and L. Li, ACS Appl. Mater. Interfaces, 2013, 5, 8254 CrossRef CAS PubMed;
(d) S. M. Supian, T. L. Ling, L. Y. Heng and K. F. Chong, Anal. Methods, 2013, 5, 2602 RSC;
(e) D. Maity and T. Govindaraju, Chem. Commun., 2012, 48, 1039 RSC;
(f) X. Sun, Y.-W. Wang and Y. Peng, Org. Lett., 2012, 14, 3420 CrossRef CAS PubMed;
(g) S. Sen, T. Mukherjee, B. Chattopadhyay, A. Moirangthem, A. Basu, J. Marek and P. Chattopadhyay, Analyst, 2012, 137, 3975 RSC;
(h) T.-H. Ma, M. Dong, Y.-M. Dong, Y.-W. Wang and Y. Peng, Chem. – Eur. J., 2010, 16, 10313 CrossRef CAS PubMed.
- For recent examples of fluorogenic Al3+ detection in organic solvents or water–organic solvent mixtures see:
(a) L. Cao, C. Jia, Y. Huang, Q. Zhang, N. Wang, Y. Xue and D. Du, Tetrahedron Lett., 2014, 55, 4062 CrossRef CAS PubMed;
(b) W.-H. Ding, W. Cao, X.-J. Zheng, W.-J. Ding, J.-P. Qiao and L.-P. Jin, Dalton Trans., 2014, 43, 6429 RSC;
(c) A. Dhara, A. Jana, N. Guchhait, P. Ghosh and S. K. Kar, New J. Chem., 2014, 38, 1627 RSC;
(d) A. Barba-Bon, L. Calabuig, A. M. Costero, S. Gil, R. Martinez-Manez and F. Sancenon, RSC Adv., 2014, 4, 8962 RSC;
(e) Y. Chen, Y. Mi, Q. Xie, J. Xiang, H. Fan, X. Luo and S. Xia, Anal. Methods, 2013, 5, 4818 RSC;
(f) Y.-W. Liu, C.-H. Chen and A.-T. Wu, Analyst, 2012, 137, 5201 RSC;
(g) S. Kim, J. Y. Noh, K. Y. Kim, J. H. Kim, H. K. Kang, S.-W. Nam, S. H. Kim, S. Park, C. Kim and J. Kim, Inorg. Chem., 2012, 51, 3597 CrossRef CAS PubMed;
(h) A. Barba-Bon, A. M. Costero, S. Gil, M. Parra, J. Soto, R. Martinez-Manez and F. Sancenon, Chem. Commun., 2012, 48, 3000 RSC;
(i) D. Maity and T. Govindaraju, Eur. J. Inorg. Chem., 2011, 5479 CrossRef CAS;
(j) H. M. Park, B. N. Oh, J. H. Kim, W. Qiong, I. H. Hwang, K.-D. Jung, C. Kim and J. Kim, Tetrahedron Lett., 2011, 52, 5581 CrossRef CAS PubMed;
(k) F. K.-W. Hau, X. He, W. H. Lam and V. W.-W. Yam, Chem. Commun., 2011, 47, 8778 RSC;
(l) A. Sahana, A. Banerjee, S. Das, S. Lohar, D. Karak, B. Sarkar, S. K. Mukhopadhyay, A. K. Mukherjee and D. Das, Org. Biomol. Chem., 2011, 9, 5523 RSC;
(m) S. H. Kim, H. S. Choi, J. Kim, S. J. Lee, D. T. Quang and J. S. Kim, Org. Lett., 2010, 12, 560 CrossRef CAS PubMed;
(n) D. Maity and T. Govindaraju, Inorg. Chem., 2010, 49, 7229 CrossRef CAS PubMed;
(o) Y.-W. Wang, M.-X. Yu, Y.-H. Yu, Z.-P. Bai, Z. Shen, F.-Y. Li and X. Z. You, Tetrahedron Lett., 2009, 50, 6169 CrossRef CAS PubMed.
-
(a) Y.-B. Ruan, A. Depauw and I. Leray, Org. Biomol. Chem., 2014, 12, 4335 RSC;
(b) T.-T. Wei, J. Zhang, G.-J. Mao, X.-B. Zhang, Z.-J. Ran, W. Tan and R. Yu, Anal. Methods, 2013, 5, 3909 RSC;
(c) T. Han, X. Feng, B. Tong, J. Shi, L. Chen, J. Zhi and Y. Dong, Chem. Commun., 2012, 48, 416 RSC.
- For optical sensors for citric acid see:
(a) C.-Y. Li, Y. Zhou, Y.-F. Li, X.-F. Kong, C.-X. Zou and C. Weng, Anal. Chim. Acta, 2013, 774, 79 CrossRef CAS PubMed;
(b) K. Ghosh and A. R. Sarkar, Org. Biomol. Chem., 2011, 9, 6551 RSC;
(c) M. I. Burguete, F. Galindo, S. V. Luis and L. Vigara, Dalton Trans., 2007, 4027 RSC;
(d) K. Ghosh, T. Sen and R. Fröhlich, Tetrahedron Lett., 2007, 48, 2935 CrossRef CAS PubMed;
(e) C. Schmuck and M. Schwegmann, Org. Biomol. Chem., 2006, 4, 836 RSC;
(f) L. Fabbrizzi, F. Foti and A. Taglietti, Org. Lett., 2005, 7, 2603 CrossRef CAS PubMed;
(g) D. Parker and J. Yu, Chem. Commun., 2005, 3141 RSC;
(h) L. A. Cabell, M. D. Best, J. J. Lavigne, S. E. Schneider, D. M. Perreault, M.-K. Monahan and E. V. Anslyn, J. Chem. Soc., Perkin Trans. 2, 2001, 315 RSC;
(i) A. Metzger and E. V. Anslyn, Angew. Chem., Int. Ed., 1998, 37, 649 CrossRef CAS.
- M. Shah, K. Thangaraj, M.-L. Soong, L. T. Wolford, J. H. Boyer, I. R. Politzer and T. G. Pavlopoulos, Heteroat. Chem., 1990, 1, 389 CrossRef CAS.
- N. Boens, V. Leen and W. Dehaen, Chem. Soc. Rev., 2012, 41, 1130 RSC.
- M. C. A. Stuart, J. C. van de Pas and J. B. F. N. Engberts, J. Phys. Org. Chem., 2005, 18, 929 CrossRef CAS.
- R. J. Sundberg and R. B. Martin, Chem. Rev., 1974, 74, 471 CrossRef CAS.
- S. Chen, Y.-M. Fang, Q. Xiao, J. Li, S.-B. Li, H.-J. Chen, J.-J. Sun and H.-H. Yang, Analyst, 2012, 137, 2021 RSC.
- For sensing ensembles based on micellar aggregates see:
(a) P. Bandyopadhyay and A. K. Ghosh, Sens. Lett., 2011, 9, 1249 CrossRef CAS PubMed;
(b) T. Riis-Johannessen, K. Schenk and K. Severin, Inorg. Chem., 2010, 49, 9546 CrossRef CAS PubMed;
(c) T. Riis-Johannessen and K. Severin, Chem. – Eur. J., 2010, 16, 8291 CrossRef CAS PubMed;
(d) F. Mancin, P. Scrimin, P. Tecilla and U. Tonellato, Coord. Chem. Rev., 2009, 253, 2150 CrossRef CAS PubMed;
(e) P. Pallavicini, Y. A. Diaz-Fernandez and L. Pasotti, Coord. Chem. Rev., 2009, 253, 2226 CrossRef CAS PubMed;
(f) M. Cametti, A. Dalla Cort and K. Bartik, ChemPhysChem, 2008, 9, 2168 CrossRef CAS PubMed.
Footnote |
† Electronic supplementary information (ESI) available: Synthetic procedures and experimental details. See DOI: 10.1039/c4ob02095b |
|
This journal is © The Royal Society of Chemistry 2015 |
Click here to see how this site uses Cookies. View our privacy policy here.