DOI:
10.1039/C4RA15482G
(Paper)
RSC Adv., 2015,
5, 19859-19864
Cross-linking of poly(vinyl alcohol) with N,N′-methylene bisacrylamide via a radical reaction to prepare pervaporation membranes
Received
3rd December 2014
, Accepted 4th February 2015
First published on 4th February 2015
Abstract
To retain the hydroxyl group of poly(vinyl alcohol) (PVA), ammonium persulfate (APS) was used to initiate the polymerization of hydrogen on the PVA chain. Rather than hydroxyl-based cross-linking, the generated PVA macromolecule radicals were cross-linked with N,N′-methylene bisacrylamide (MBA) to prepare PVA membranes. The PVA membranes were shown to be successfully cross-linked via FT-IR characterization, as well as measurements of swelling degree and gel fraction. With increasing the content of cross-linker, the swelling degree of the membranes decreased, and the gel fraction increased. The pervaporation performance of the membrane was investigated by separating 95 wt% ethanol aqueous solutions. The cross-linked PVA membrane containing 0.5 wt% cross-linker yielded a high permeate water content (99 wt%) and a total flux of 353 g m−2 h−1 at 40 °C. As the feed temperature increased, the total flux increased, while the permeate water content decreased. The cross-linked membrane exhibited good durability over long-term operation.
Introduction
Pervaporation (PV) is an energy-saving membrane process that is used to separate azeotropic and close-boiling mixtures, dehydrate organic solvents, and concentrate organic aqueous solutions.1–6 Among these applications, PV dehydration of organic solvents has obvious technical and economic advantages.7 PV dehydration performance is dependent on the membrane material.
Hydrophilic polymers are widely used in the PV dehydration of alcohols and other organic solvents.8–13 Poly(vinyl alcohol) (PVA)14 is an attractive hydrophilic membrane material due to its low cost, excellent membrane formation properties as well as mechanical strength, thermal stability, and chemical resistance.15–18
The outstanding hydrophilicity of PVA is due to an abundance of hydroxyl groups in the linear PVA chain. This hydroxyl-rich structure facilitates PVA swelling in aqueous solutions; such swelling leads to decrease membrane selectivity. To prevent excessive swelling, modified methods such as grafting,19,20 blending,21,22 hybridization,15,23 substitution24 and cross-linking have been investigated. Cross-linking is the most popular method used to minimize swelling, and many cross-linked PVA membranes have been reported using different cross-linking agents. These cross-linking agents include carboxylic acids and carboxylic acid derivatives,25–29 aldehydes30 and silane coupling agents.31
However, these cross-linking methods all react the hydroxyl group with the cross-linker, consuming hydroxyl groups in the PVA chains and decreasing membrane hydrophilicity. Katz et al. cross-linked PVAs to prepare reverse osmosis membranes by radiation, generating membranes with high water permeabilities and low selectivities between water and salt.32 In another work, PVA was modified and insolubilized via a reaction with potassium peroxydisulfate (K2S2O8), then used to fabricate reverse osmosis membranes on tubular poly(ether sulfone) ultrafiltration substrates.33 The cross-linked PVA reverse osmosis membrane had a flux of 47.1 L m−2 h−1 with a rejection of 46% for 2000 ppm NaCl aqueous solution at 20 °C and 2 MPa. Although PVA membranes cross-linked by radical reaction retain strong hydrophilicities, the degree of cross-linking in these membranes is low, as self-cross-linking of radicals does not readily occur. Because both hydrophilicity and degree of cross-linking are important factors for higher pervaporation membrane flux and selectivity, retaining the hydroxyl group of poly(vinyl alcohol) (PVA) via a radical reaction in the presence of cross-linker is a desirable cross-linking method.
N,N′-Methylene bisacrylamide (MBA) is water-soluble and has two terminal vinyl groups that can react with radicals. In this work, APS was used as initiator, PVA/PAN composite membranes cross-linked with MBA in the presence of APS were prepared by a coating method. The swelling degree and gel fraction of cross-linked PVA membranes were determined. The PV dehydration performance of PVA/PAN composite membranes was studied under different operating conditions. In addition, the stability of cross-linked PVA composite membranes was also investigated.
Experimental
Materials and instruments
PVA with a polymerization degree of 1700 and an alcoholysis degree of 99% was provided by Chongqing Chuanwei Petrochemical Engineering Co., Ltd. (China). (NH4)2S2O8 (AR) and ethanol (AR) were provided by Shanghai Chemical Reagent Co., Ltd. (China). MBA (CP) was obtained from Aladdin Reagent Co., Ltd. (China). Poly(acrylonitrile) (PAN) plate ultrafiltration membranes were purchased from Shanghai Megavision Membrane Engineering & Technology Co., Ltd. (China). Deionized water was generated in the lab.
A gas chromatograph (GC-950, China), Fourier transform infrared (FT-IR) spectrometer (Nexus 670, America) and Scanning Electron Microscope (Ultra 55, Germany) were used.
Membrane preparation
PVA was dissolved in deionized water and stirred at 90 °C for 6 h to form a 5 wt% aqueous solutions. When cooled to room temperature, APS and MBA were added, and the mixture was stirred for 6 h. The solution was then degassed to produce the membrane casting solution. To prepare the composite membrane, the degassed solution was cast onto a PAN ultrafiltration membrane covering a glass plate using a lab-made casting knife. The casting film was heated in an oven at 80 °C for 4 h to allow the cross-linking reaction to occur as shown in Fig. 1. Homogeneous cross-linking membrane was prepared by casting the mixture dope onto a glass plate and treated as described above.
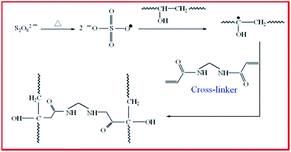 |
| Fig. 1 Cross-linking reaction between PVA, APS and MBA. | |
Fourier transform infrared (FT-IR) spectroscopy
FT-IR spectroscopy was used to characterize the chemical composition of the membranes before and after cross-linking. All samples were scanned by a Nexus 670 FT-IR spectrometer with a scanning range of 800–4000 cm−1.
Scanning electron microscopy (SEM)
The surface and cross-section morphologies of PAN ultrafiltration and cross-linked PVA/PAN membranes were visualized with SEM. Membrane cross-sections were broken in liquid nitrogen and then dried in an oven. All samples were sputter-coated with gold before characterization.
Degree of swelling
Cross-linked homogeneous membranes with equal dimensions were weighed and immersed in deionized water at 25 °C for 24 h to allow the equilibrium state to be reached. The swollen membranes were removed from water, rapidly wiped with filter paper, and weighed immediately. The degree of swelling (S) of each membrane was determined by eqn (1): |
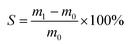 | (1) |
where m1 and m0 are the weights of wet and dry membranes, respectively.
Gel fraction
Each cross-linked homogeneous membrane was weighed and placed in a flask filled with deionized water at 100 °C for 12 h. The wet membranes were then removed from water and dried in a vacuum oven at 50 °C for 24 h. The treated membranes were weighed, and the gel fraction (G) was expressed as eqn (2): |
 | (2) |
where m1 and m0 are the weights of the treated and original membranes, respectively.
Pervaporation experiments
Pervaporation experiments were carried out in a lab-made piece of equipment depicted in Fig. 2. The membrane had an effective area of 18.1 cm2. The feed solution was a 95 wt% ethanol aqueous solutions, and the temperature was controlled by a constant-temperature water bath. A vacuum pump was used to maintain the downstream pressure at 200 Pa. Prior to each experiment, the feed solution was allowed to circulate for 2 h. Then, the vacuum pump was turned on, and the permeate was collected in a liquid nitrogen-cooled permeate collection tube. The compositions of the permeate and feed solutions were analysed by gas chromatography. Each membrane was tested three times, and average values are reported.
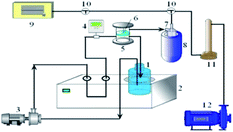 |
| Fig. 2 Pervaporation setup (1-feed tank, 2-constant temperature bath, 3-circular pump, 4-thermometer, 5-membrane cell, 6-membrane, 7-permeate collection tube, 8-cold trap, 9-vacuometer, 10-three-way glass stopcock, 11-desiccator, 12-vacuum pump). | |
The separation performance of PVA/PAN composite membranes is characterized by the permeation flux (J) and the separation factor (α).
The permeation flux (J, g m−2 h−1) can be obtained as eqn (3):
|
 | (3) |
where
W (g),
A (m
2), and
t (h) are the weight of permeate solution, the effective area of the membrane, and the collection time, respectively.
The separation factor (α) can be defined as follows:
|
 | (4) |
where
yi and
yj are the mass fractions of water in the permeate and feed solution, and
xi and
xj are the mass fractions of ethanol in the permeate and feed solution, respectively.
Results and discussion
FT-IR results
The original and cross-linked PVA membranes can be characterized by their FT-IR spectra, shown in Fig. 3. The distinct broad adsorption bands at 3000–3500 cm−1 correspond to the hydroxyl group. Compared to the original PVA membrane, the cross-linked membrane exhibits a new adsorption band near 3100 cm−1; this band corresponds to the N–H group of the cross-linker. Meanwhile, the relative intensity of the hydroxyl group decreases a little of the cross-linked membrane. This is due to the presence of initiator and cross-linker in PVA casting solutions, the PVA content in cross-linked membrane decreases. For the cross-linked membrane, the relative intensity of the C–H bands near 2900 cm−1 are lower than the original membrane, indicating that the number of C–H groups in the PVA membrane decreased after cross-linking. The 1750 cm−1 peak corresponds to C
O; this peak is broadened after cross-linking due to the carbonyl group of the cross-linked polymer. The peaks at 1450 and 1200 cm−1 are due to stretching vibrations of C–H and C–O groups, respectively. The intensity of these two peaks in the cross-linked PVA membrane increased because the N–H and C–N groups of cross-linker overlap with the C–H and C–O groups.
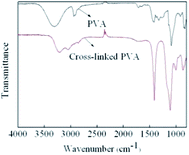 |
| Fig. 3 FTIR spectra of the original and cross-linked PVA membranes. | |
SEM
Fig. 4 contains SEM micrographs of the surface and cross-section of the PAN support membrane as well as the cross-linked PVA/PAN composite membrane. The cross-linked PVA/PAN composite membrane consists of two layers: the PAN support layer and the cross-linked PVA selective layer. These two layers are tightly integrated. The surface of the PAN support membrane is porous, while the cross-linked PVA/PAN composite membrane has a dense surface. A selective layer 10 μm thick was observed on the PAN support layer, indicating that the PVA membrane was successfully cast on the PAN support membrane.
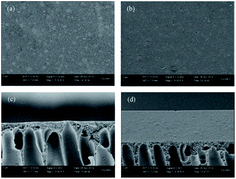 |
| Fig. 4 SEM micrographs of the PAN support membrane and the cross-linked PVA/PAN composite membrane: (a) surface of PAN; (b) surface of PVA/PAN; (c) cross-section of PAN; (d) cross-section of PVA/PAN. | |
Degree of swelling
The effect of cross-linking degree on the swelling degree of membrane in pure water was determined. The variation of swelling degree with cross-linker content is shown in Fig. 5. When cross-linker content was increased, the swelling degree decreased from 52% to 41%. When the cross-linker content exceeded 0.5 wt%, only a slight change in swelling degree was observed. The degree of cross-linking increases with MBA content, decreasing the mobility of the PVA chains. As a result, water cannot easily penetrate into the PVA membrane to cause swelling.
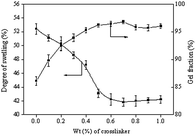 |
| Fig. 5 Variations of degree of swelling and gel fraction of membrane with cross-linker content. | |
The free volume of the polymer is also decreased by the limited mobility of the PVA polymer chains. By decreasing the free volume of the polymer, the degree of swelling was also decreased. The degree of swelling remained nearly constant when cross-linker content exceeded 0.5 wt%, indicating that cross-linking degree changes little when the amount of mixed cross-linker is above a certain concentration.
Gel fraction
Fig. 5 shows the variation of gel fraction of cross-linked membranes with cross-linker content. Gel fraction is a simple and effective method to verify whether PVA membranes are cross-linked. Pristine PVA membranes are fully dissolved in hot water. However, cross-linked PVA membranes are not soluble in hot water. Therefore, gel fraction reflects the cross-linking degree of the membranes.
As seen in Fig. 5, when the cross-linker content in the casting solution was increased, the gel fraction of the cross-linked membranes increased. Similar to the degree of swelling, the gel fraction of the membranes remained nearly constant when the cross-linker content exceeded 0.5 wt%. This phenomenon could be explained by increased cross-linking efficiency resulting from the cross-linker. When the cross-linker is included in the membrane casting solution, PVA macromolecule chains are more easily cross-linked. Therefore, the soluble fraction of the membranes decreased, and the gel fraction of the membranes increased. However, further increases in cross-linker content may not affect the gel fraction of PVA membranes, as the cross-linking degree of PVA membranes does not increase when the cross-linker exceeds a certain concentration.
Pervaporation performance
The pervaporation performance of membranes is closely related to feed temperature. To study the influence of feed temperature, four temperatures (40 °C, 50 °C, 60 °C, 70 °C) were considered. Experiments were carried out using aqueous ethanol solutions containing 5 wt% water at different temperatures. The downstream pressure was maintained at 200 Pa. The effect of feed temperature on total flux and permeate water content is shown in Fig. 6.
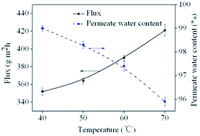 |
| Fig. 6 Flux and permeate water content as a function of feed temperature. | |
Similar to results reported in the literature, Fig. 6 indicates that total flux increases as feed temperature is increased. For example, flux increased from 353 g m−2 h−1 to 422 g m−2 h−1 as the temperature was increased from 40 °C to 70 °C. The relationship of feed temperature and permeate flux can be calculated by the Arrhenius equation:
|
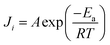 | (5) |
where
J (g m
−2 h
−1) is the permeate flux of component
i,
A is constant.
Ea (kJ mol
−1) is the activation energy,
R (J mol
−1 K
−1) is gas constant, and
T (K) is feed temperature.
As the feed temperature is increased, PVA chain mobility strengthens, increasing the free volume of the membrane. This increase in free volume allows feed components to permeate the membrane more easily, increasing total permeate flux. Arrhenius plot of ln
J versus 1/T for the cross-linked PVA membranes are displayed in Fig. 7. The Ea values of ethanol and water are 47.02 kJ mol−1, 4.46 kJ mol−1, respectively. As because water has a lower activation energy than ethanol, the permeate flux of ethanol tended to increase faster than that of water when the feed temperature was increased, leading to lower selectivity.
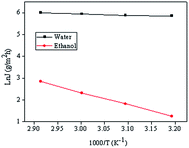 |
| Fig. 7 Arrhenius plot of ln J versus 1/T for the cross-linked PVA membrane. | |
PV performance is also related to feed concentration. Experiments were carried out using ethanol aqueous solutions containing 1, 5, 10 and 15 wt% water at 40 °C. The results are shown in Fig. 8. When water concentration of feed was increased, flux increased, and the permeate water content decreased. The reason can be explained as follows. The membrane would be plasticized or swollen by high concentration of water, enhancing the free volume of the membrane. As a result, the transfer resistance of water and ethanol decreased, the permeate flux increased. Meanwhile, more ethanol could diffuse through the membrane, and the permeate water content decreased.
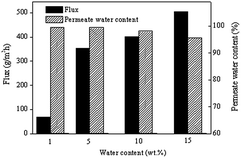 |
| Fig. 8 Effect of feed water concentration on pervaporation performance. | |
Table 1 compares the flux and separation factor of the PVA membranes from this work with other cross-linked PVA membranes for the pervaporation separation of ethanol–water mixtures. Table 1 clearly indicates that the cross-linked PVA membranes from this work have the highest flux and separation factor. This result is due to the fact that cross-linking of PVA membranes with aldehydes, carboxylic acids and silane coupling agents consume a portion of the hydroxyl groups in PVA, decreasing membrane hydrophilicity. In contrast, the PVA membranes cross-linked with MBA in this work retained the hydroxyl groups, and the membranes exhibit good pervaporation performance.
Table 1 Comparison of pervaporation performance of cross-linked PVA membranes for dehydration of ethanol–water mixtures
Cross-linker |
Temp. (°C) |
Feed water content (wt%) |
Flux (g m−2 h−1) |
Separation factor |
Ref. |
Glutaraldehyde |
40 |
5 |
94 |
100 |
34 |
Glutaraldehyde |
40 |
10 |
280 |
104 |
35 |
Maleic acid |
30 |
20 |
201 |
22 |
36 |
Maleic acid |
50 |
5 |
60 |
93 |
37 |
Fumaric acid |
60 |
20 |
217 |
779 |
15 |
Sulfosuccinic acid |
40 |
10 |
54 |
50 |
38 |
γ-Aminopropyltriet-hoxysilane |
50 |
5 |
36 |
537 |
39 |
Tetraethoxysilane |
40 |
15 |
50 |
329 |
40 |
Sulfated zirconia |
50 |
5 |
10 |
263 |
41 |
MBA |
40 |
5 |
353 |
3781 |
This work |
Membrane durability
To investigate the pervaporation performance stability of membranes, experiments were carried out at 40 °C for 48 h with aqueous ethanol solutions containing 5 wt% water. Membrane stabilities are illustrated in Fig. 9. The downstream pressure was maintained at 200 Pa. As shown in Fig. 9, the permeate water content remained above 99 wt%, even as operating time was increased. Total membrane flux changed only slightly from 353 g m−2 h−1 to 346 g m−2 h−1 after 48 h. This performance is most likely observed because PVA membranes cross-linked by MBA in the presence of APS cannot swell significantly in 95 wt% ethanol aqueous solutions. The membranes therefore exhibit good long-term stability when used for the dehydration of aqueous ethanol mixtures.
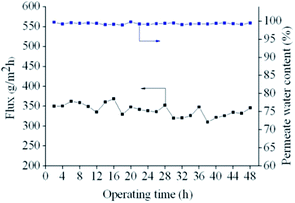 |
| Fig. 9 Membrane stability for the separation of 95 wt% ethanol aqueous solutions at 40 °C. | |
Conclusions
PVA pervaporation membranes were prepared via cross-linking of PVA and MBA in the presence of APS for the separation of ethanol–water mixtures. The swelling degree and gel fraction of the membranes were affected by the content of cross-linker. Cross-linked membranes containing 0.5 wt% cross-linker exhibited high permeate water content (99 wt%) and a total flux of 353 g m−2 h−1 for the pervaporation separation of 95 wt% ethanol aqueous solutions. Total flux was found to increase with increasing feed temperature, while the selectivity was found to decrease. Moreover, the cross-linked membranes also maintained good performance when operated for 48 h. The cross-linked PVA/PAN composite membranes in this work are therefore excellent candidates for the dehydration of ethanol–water mixtures.
Acknowledgements
The authors acknowledge financial support for this work from the Zhejiang Provincial Natural Science Foundation of China (no. LR12B06001), the National Basic Research Program of China (2011CB710804), National Hi-Tech Research and Development Program of China (2012AA022303), and the State Key Laboratory of Motor Vehicle Biofuel Technology (2013008).
Notes and references
- J. Chen, H. Huang, L. Zhang and H. Zhang, RSC Adv., 2014, 4, 24126–24130 RSC.
- Y. X. Bai, J. W. Qian, C. F. Zhang, L. Zhang, Q. F. An and H. L. Chen, J. Membr. Sci., 2008, 325, 932–939 CrossRef CAS.
- X. Y. Qu, H. Dong, Z. J. Zhou, L. Zhang and H. L. Chen, Ind. Eng. Chem. Res., 2010, 49, 7504–7514 CrossRef CAS.
- K. Sunitha, S. V. Satyanarayana and S. Sridhar, Carbohydr. Polym., 2012, 87, 1569–1574 CrossRef CAS.
- S. Li, F. Qin, P. Qin, M. N. Karim and T. Tan, Green Chem., 2013, 15, 2180 RSC.
- J. Tang, K. K. Sirkar and S. Majumdar, J. Membr. Sci., 2013, 447, 345–354 CrossRef CAS.
- W. Kaminski, J. Marszalek and A. Ciolkowska, Chem. Eng. J., 2008, 135, 95–102 CrossRef CAS.
- K. S. V. Krishna Rao, B. Vijaya Kumar Naidu, M. C. S. Subha, M. Sairam, N. N. Mallikarjuna and T. M. Aminabahvi, Carbohydr. Polym., 2006, 66, 345–351 CrossRef.
- S. Qiu, L. G. Wu, G. Z. Shi, L. Zhang, H. L. Chen and C. J. Gao, Ind. Eng. Chem. Res., 2010, 49, 11667–11675 CrossRef CAS.
- B. Smitha, G. Dhanuja and S. Sridhar, Carbohydr. Polym., 2006, 66, 463–472 CrossRef CAS.
- P. Wei, X. Y. Qu, H. Dong, L. Zhang, H. L. Chen and C. J. Gao, J. Appl. Polym. Sci., 2013, 130, 3979–3984 CAS.
- P. Wei, X. Y. Qu, H. Dong, L. Zhang, H. L. Chen and C. J. Gao, J. Appl. Polym. Sci., 2013, 128, 3390–3397 CrossRef CAS.
- A. K. Thakur, S. Gahlot, V. Kulshrestha and V. K. Shahi, RSC Adv., 2013, 3, 22014–22022 RSC.
- Y. Sakurada, A. Sueoka and M. Kawahashi, Polym. J., 1987, 19, 501–513 CrossRef CAS.
- Z. Huang, H. Guan, W. Tan, X. Qiao and S. Kulprathipanja, J. Membr. Sci., 2006, 276, 260–271 CrossRef CAS.
- S. B. Kuila and S. K. Ray, Carbohydr. Polym., 2014, 101, 1154–1165 CrossRef CAS PubMed.
- T. Uragami, H. Matsugi and T. Miyata, Macromolecules, 2005, 38, 8440–8446 CrossRef CAS.
- K. Zhou, Q. G. Zhang, G. L. Han, A. M. Zhu and Q. L. Liu, J. Membr. Sci., 2013, 448, 93–101 CrossRef CAS.
- N. Alghezawi, O. Şanlı, L. Aras and G. Asman, Chem. Eng. Process., 2005, 44, 51–58 CrossRef CAS.
- T. M. Aminabhavi and H. G. Naik, J. Appl. Polym. Sci., 2002, 83, 273–282 CrossRef CAS.
- Y. Q. Dong, L. Zhang, J. N. Shen, M. Y. Song and H. L. Chen, Desalination, 2006, 193, 202–210 CrossRef CAS.
- W. Lin, Q. Li and T. Zhu, J. Ind. Eng. Chem., 2012, 18, 941–947 CrossRef CAS.
- W. W. Hu, X. H. Zhang, Q. G. Zhang, Q. L. Liu and A. M. Zhu, J.
Appl. Polym. Sci., 2012, 126, 778–787 CrossRef CAS.
- X. Liu, J. Chen, J. Zou, H. Duan, J. Li, C. Chen and P. Meng, J. Appl. Polym. Sci., 2011, 119, 2584–2594 CrossRef CAS.
- H. M. Guan, T. S. Chung, Z. Huang, M. L. Chng and S. Kulprathipanja, J. Membr. Sci., 2006, 268, 113–122 CrossRef CAS.
- M. N. Hyder, R. Y. M. Huang and P. Chen, J. Membr. Sci., 2009, 326, 363–371 CrossRef CAS.
- P. Das, S. K. Ray, S. B. Kuila, H. S. Samanta and N. R. Singha, Sep. Purif. Technol., 2011, 81, 159–173 CrossRef CAS.
- S. S. Xia, X. L. Dong, Y. X. Zhu, W. Wei, F. J. Xiangli and W. Q. Jin, Sep. Purif. Technol., 2011, 77, 53–59 CrossRef CAS.
- H.-K. Yuan, J. Ren, X.-H. Ma and Z.-L. Xu, Desalination, 2011, 280, 252–258 CrossRef CAS.
- W. H. Lin, Q. Li and T. R. Zhu, J. Ind. Eng. Chem., 2012, 18, 941–947 CrossRef CAS.
- S. Razavi, A. Sabetghadam and T. Mohammadi, Chem. Eng. Res. Des., 2011, 89, 148–155 CrossRef CAS.
- M. G. Katz and T. Wydeven, J. Appl. Polym. Sci., 1981, 26, 2935–2946 CrossRef CAS.
- D. Bezuidenhout, M. J. Hurndall, R. D. Sanderson and A. J. van Reenen, Desalination, 1998, 116, 35–43 CrossRef CAS.
- C. K. Yeom, S. H. Lee and J. M. Lee, J. Appl. Polym. Sci., 2001, 79, 703–713 CrossRef CAS.
- V. S. Praptowidodo, J. Mol. Struct., 2005, 739, 207–212 CrossRef CAS.
- B. V. Di Carlo and A. C. Habert, J. Mater. Sci., 2013, 48, 1457–1464 CrossRef.
- Y. M. Wei, Z. L. Xu, F. A. Qusay and K. Wu, J. Appl. Polym. Sci., 2005, 98, 247–254 CrossRef CAS.
- J. W. Rhim, S. W. Lee and Y. K. Kim, J. Appl. Polym. Sci., 2002, 85, 1867–1873 CrossRef CAS.
- Q. G. Zhang, Q. L. Liu, Z. Y. Jiang and Y. Chen, J. Membr. Sci., 2007, 287, 237–245 CrossRef CAS.
- T. Uragami, K. Okazaki, H. Matsugi and T. Miyata, Macromolecules, 2002, 35, 9156–9163 CrossRef CAS.
- K. J. Kim, S. H. Park, W. W. So and S. J. Moon, J. Appl. Polym. Sci., 2001, 79, 1450–1455 CrossRef CAS.
|
This journal is © The Royal Society of Chemistry 2015 |
Click here to see how this site uses Cookies. View our privacy policy here.