DOI:
10.1039/C5RA05124J
(Paper)
RSC Adv., 2015,
5, 42362-42368
Simultaneously enhanced tensile strength and fracture toughness of epoxy resins by a poly(ethylene oxide)-block-carboxyl terminated butadiene-acrylonitrile rubber dilock copolymer
Received
23rd March 2015
, Accepted 6th May 2015
First published on 6th May 2015
Abstract
A PEG-b-CTBN diblock copolymer was synthesized via the esterification reaction of hydroxy terminated methoxypolyethylene glycols (PEG) in the presence of a carboxyl-terminated butadiene-co-acrylonitrile copolymer (CTBN) with 4-dimethylaminopyridine (DMAP) as the catalyst. Epoxy thermosets were modified via the formation of a nanostructure. The result of transmission electronic microscopy (TEM) shows spherical domains were homogeneously dispersed into the continuous epoxy matrix and the size is nano-scale. It was noted that the glass transition temperatures decreased with an increase in the content of PEG-b-CTBN, which was evidenced by Dynamic Mechanical Analysis (DMA) and a Differential Scanning Calorimeter (DSC). Through mechanical tests, we found the tensile strength and fracture toughness of epoxy resins were simultaneously enhanced by nanostructured PEG-b-CTBN.
1 Introduction
Epoxy resins are a most important class of thermosetting resins for many engineering applications (i.e., aerospace, electrical etc.) because of their high strength and stiffness, resistance to chemicals, good dielectric behavior, low shrinkage during curing, corrosion and microbial organisms and good thermal characteristics. Neat epoxy resins, however, have very low crack growth resistance and are one of the most brittle polymeric materials. So their applications as matrices for high performance composites are greatly limited.
The approaches to toughen brittle epoxy resin with reactive liquid rubbers (LR), thermoplastic resin, inorganic whiskers and rigid nanoparticles etc., have comprehensively been studied. Dadfar1 demonstrated that the fracture toughness of modified resin increased by carboxyl terminated butadiene-acrylonitrile rubber (CTBN) content, whereas tensile strength of composites decreased. Chaudhary2 indicated that the izod impact strength and fracture energies (GIC) increased by 33% and 150%, respectively, on introduction of 3% amine-functionalized poly(styrene). Milad Zamanian3 reported that the addition of the nanosilica particles did not affect the glass transition temperature, but the critical stress intensity factors (KIC) increased linearly with the volume fraction of fillers. In general, liquid rubbers could greatly improve the fracture toughness and impact resistance of the composite, while significantly lose strength of epoxy resin. Although thermoplastic resins can overcome the disadvantage of rubber elastomer relatively, the toughening effect was less than rubber elastomer. In terms of inorganic whiskers and rigid nanoparticles, the dispersity of these particles and interfacial adhesion were relatively poor.
Recently, nano-rubber particles have gained much attention, because the interactions between thermosetting matrix and modifiers can further optimize, thus the mechanical properties of materials were significantly improved, which has been called “toughening by nanostructures”.4 Yang Zhao's5 study indicated that the tensile strength and fracture toughness are simultaneously enhanced by the addition of nano-rubber particles. Tang6 demonstrated that adding powered rubber to epoxy/liquid rubbers binary composites impact resistance of epoxy composites were remarkable improved, and tensile properties showed slight change compared with the neat epoxy. As for the epoxy binary composites with nano-sized rubber particles, although stirrer provided a high shear force, small agglomerates still remain present in the matrix. In addition, the composite cannot be well mixed owing to the increased viscosity.
Block copolymer, because of the different properties of the blocks, exhibits an overall performance. And the interaction between different blocks limits the size of the block copolymer in matrix, which could lead to the formation of nanostructures. Block copolymer modified epoxy resins have generated significant interest since it was demonstrated that the combination could lead to nanostructured thermosets. It has been identified that the formation of the nanostructures could follow either self-assembly7,8 or reaction-induced microphase separation (RIMS) mechanism.9,10 Compared with the aforementioned methods, block copolymer may preserve the advantage of liquid rubbers and offset the disadvantage of powered rubber particles. In this work, amphiphilic diblock copolymer was prepared by PEG and CTBN. Because PEG is miscible with epoxy thermosets after and before curing reaction cured with aromatic amine (i.e. MOCA) due to the formation of the intermolecular hydrogen bonding interactions11 whereas CTBN is one of the most classic successful case of toughen epoxy. Nanostructure thermosetting composites was prepared through reaction-induced microphase separation own to the difference in miscibility of carboxyl terminated butadiene-acrylonitrile rubber (CTBN)12 and polyethylene glycols blocks with epoxy resin after and before curing reaction. The morphology, and its effect on mechanical properties of epoxy resin were investigated.
2 Experimental
2.1 Materials
The carboxyl-terminated butadiene-acrylonitrile rubber (CTBN 1300 × 18) was supplied by Emerald Company, USA, and it has a quoted molecular weight to be Mn = 3125 g mol−1. Methoxypolyethylene glycols was obtained from Aladdin with an average molecular weight to be Mn = 1000 g mol−1. The catalyst is 4-dimethylaminopyridine (DMAP), supplied by kelong Co., China. Epoxy resin used in this work is diglycidyl ether of bisphenol A-based (DGEBA) epoxy resin E-51, which was obtained from Jiangsu Wuxi Resin Plant, China. 3,3′-Dichloro-4,4′-diamino diphenyl methane (MOCA) which was purchased from Changshan beier Co., Ltd. (China) is used as curing agent.
2.2 Synthesis of PEG-b-CTBN
Poly(ethylene glycol)-block-carboxyl terminated butadiene-acrylonitrile rubber diblock copolymer (PEG-b-CTBN) was synthesized via the esterification reaction with 4-dimethylaminopyridine (DMAP) as the catalyst. Prior to polymerization, polyethylene glycol was carefully dried at 75 °C for a night in a vacuum. Methoxypolyethylene glycols with Mn = 1000 g mol−1 (10 g), CTBN with Mn = 3125 g mol−1 (31.25 g) and DMAP (0.12 g) were charged into a three-neck round bottom flask adapted with a mechanical stirrer. A slight excess of CTBN was used to ensure that hydroxy terminals were completely reacted. The mixture was immersed in a thermostated oil bath between 100–110 °C under N2 purge for 17 h without using any solvent. After the reaction, the mixture was slowly cool down at room temperature under N2 purge. Considering high reaction temperature and nitrogen purging, it is believed water byproduct was removed continuously during the reaction and thus enhance the reaction equilibrium to the condensation products. The resulting polymer was purify three times and dried in a vacuum oven prior to use.
2.3 Preparation of epoxy thermosets containing PEG-b-CTBN
The desired amount of DGEBA and PEG-b-CTBN was mixed at 80 °C for 5 min with vigorous stirring and the curing agent (MOCA) was added with continuous stirring until transparent and homogenous mixtures were obtained. The mixtures was degassed under vacuum, and poured into preheated Teflon moulds and subjected to the thermal curing at 150 °C for 2 h plus 180 °C for 2 h for post curing. The thermosets containing PEG-b-CTBN up to 20 wt% were prepared. The preparation of PEG–CTBN modified epoxy composites was shown in Scheme 1.
 |
| Scheme 1 Preparation of PEG–CTBN modified epoxy composites. | |
2.4 Measurement and characterization
2.4.1 Fourier transform infrared spectroscopy (FTIR). FTIR spectrum was obtained by a Fourier transform infrared spectrometer (Nicolet 560) at room temperature (25 °C). The block copolymer was mixed with potassium bromide (KBr) pellets to press into the small disks for measurements. The spectra were recorded over the spectral range 400–4000 cm−1.
2.4.2 Nuclear magnetic resonance spectroscopy (NMR). The NMR measurements were carried out on a DRX-400 (Bruker Company, Germany) 400 MHz NMR spectrometer to obtain 1H-NMR spectra at 25 °C. The samples were dissolved in CDCl3.
2.4.3 Transmission electron microscopy (TEM). The dispersion of diblock copolymer and phase morphology in nanocomposites was observed by a transmission electron microscope (TEM; Tecnai G2 F20, FEI, USA), at an acceleration voltage of 120 kV. The ultrathin sections with a thickness of 100 nm were cryogenically microtomed by using an ultra-microtome (EM UC7, LEICA, Germany). The section sample was stained with OSO4 to increase the contrast.
2.4.4 Differential scanning calorimeter (DSC). Differential scanning calorimeter was used to determine glass transition temperature and prove the miscibility of PEG. Cured materials (about 7.7–8.6 mg) were placed in hermetically sealed aluminum pans. A Netzsch Instruments DSC-204 was used in the analysis. Specimens were first equilibrated at 20 °C for 3 min, subsequently, the samples were scanned from 20 to 200 °C at a heating rate of 20 °C min−1. Glass transition temperature (Tg) was taken as the midpoint of heat capacity change.
2.4.5 Dynamic mechanical analysis (DMA). Dynamic mechanical experiment was performed at 40 Hz with a heating rate of 3 °C min−1 from 60 to 200 °C with the three-point bending mode by using a TA Instruments Q800 (USA) apparatus. The samples were rectangular bars with size of 20 mm × 10 mm × 4 mm.
2.4.6 Scanning electron microscopy (SEM). The fracture surface of the specimens after fracture toughness tests were observed by scanning electron microscope (SEM; JSM-5900, JEOL, Tokyo, Japan) instrument with an acceleration voltage of 5 kV. The fracture surfaces were coated with thin layers of gold to ensure surface conductivity during observation.
2.4.7 Mechanical properties. Tensile properties of the cured specimens were measured with an Instron (Instron 5567, Instron, USA) universal testing instrument at a rate of 10 mm min−1 according to GB/T 1040.2-2006. Test specimens were examined for each composition and the average result of five highest readings at peak load was reported as tensile strength. The strain values at the breaking point were also used to characterize the properties of the composite. All mechanical values were taken from an average of five samples.The izod notched impact strength of the cured specimens was tested with an Izod Impact Tester (MTS Co.) according to GB/T 2567-2008. The size of the tested specimen was 4 mm × 10 mm × 80 mm. All mechanical property values were obtained by averaging the five experimental values.
2.4.8 Fracture toughness measurements. Fracture toughness was measured by the notched three-point bending test with a crosshead speed of 2 mm−1 according to the standard of ASTM E399. The schematic diagram of the three-point bending specimens is shown in Scheme 2. The critical stress intensity factors (KIC) were calculated using the following equation: |
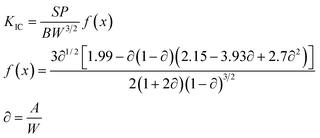 | (1) |
where S is the tested span, P the peak load, f the shape factor, B the specimen thickness, W the specimen width and A the crack length. Before measurement, all the specimens were annealed at 80 °C for 6 h and at least five successful measurements were used to obtain the average values of the measurements.
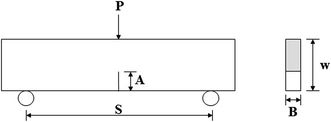 |
| Scheme 2 Schematic diagram of three-point bending specimen for the measurement of critical stress intensity factor (KIC). | |
3 Results and discussion
3.1 Synthesis of PEG-b-CTBN diblock copolymer
The FTIR spectrum of PEG–CTBN is shown in Fig. 1. The absorption bands at 2860 cm−1 indicate the presence of methylene (–CH2–) moieties in PEG, assignable to the stretching vibration of C–H bonds. The bands at 3431.2 cm−1 and 1105.2 cm−1 are attributed to the hydroxy group and C–O–C in PEG. The carbonyls (C
O) and –CH
CH2 are characteristic of the stretching vibration at 1714.7 and 1641 cm−1, respectively. The band at 2237 cm−1 is attributed to the stretching vibration of –CN group in CTBN. The bands at 970.4 and 724.2 cm−1 are ascribed to the out-of-plane bending vibration
C–H bonds in trans-1,4 and cis-1,4 moieties in CTBN, respectively. The weak absorption at 917 cm−1 is attributed to the out-of-plane bending vibration of 1,2-structures in the CTBN subchains. The ester carbonyls (C
O) of PEG-PDMS are characteristic of the stretching vibration at 1730.7 cm−1. The spectral results indicate that the resulting polymer combined the structural features from both PEG and CTBN.
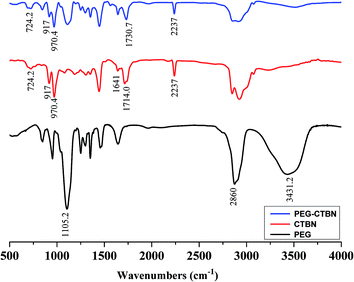 |
| Fig. 1 FT-IR spectra of pure PEG and PEG-b-CTBN diblock copolymer. | |
The 1H NMR spectrum of the copolymer is shown in Fig. 2. The 1H-NMR (CDCl3 ppm): 1.25 (–CH2–C–CN, –CH2–C–C
C), 2.18–2.5 (–CH2–C
C–CH2–, –C–CH–C
C), 2.5–2.8 (–C–CH–CN), 3.65 (–CH2–CH2–O–), 4.9–5.3 (–C
CH2), 5.3–5.7 (–CH
CH–, –CH
C), 7.26 (CDCl3). The results are consistent with the results of FTIR, which indicated the success of synthesis PEG-b-CTBN. The results are consistent with the results of FTIR, which indicated the success of synthesis PEG-b-CTBN.
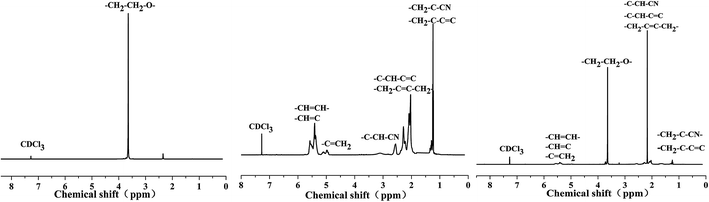 |
| Fig. 2 1H NMR spectrum of PEG (a), CTBN (b) and PEG-b-CTBN (c) diblock copolymer. | |
3.2 Nanostructures in epoxy thermosets containing PEG-b-CTBN
In the range of compositions investigated, all the thermosetting blends of epoxy with PEG-b-CTBN were homogenous and transparent, indicating that no macroscopic phase separation occurred at least on the scale exceeding the wavelength of visible light. The TEM micrograph of the thermosets containing 15 wt% PEG-b-CTBN is presented in Fig. 3. The dark objects are assigned to CTBN microphases since the CTBN containing C
C double bonds can be preferentially stained with OsO4 whereas the epoxy matrix that is miscible with PEG was almost unaffected. It is seen that spherical CTBN domains with the average size about 10 nm were homogeneously dispersed into the continuous epoxy matrix, which indicate that PEG-b-CTBN was possessed the microphase-separated morphology in the epoxy thermosets and the success of preparing nanoscale composite.
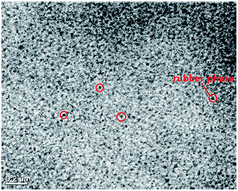 |
| Fig. 3 The TEM micrograph of the thermosets containing 15 wt% PEG-b-CTBN. | |
3.3 Differential scanning calorimetry (DSC)
Glass transition temperature Tg of the neat and diblock copolymer modified epoxy resins was measured by means of DSC. The variation in Tg for epoxy systems with respect to PEG–CTBN content is given in Fig. 4. It known that the melting transition of the PEG is about 66.2 °C. But all the nanostructured thermosets containing the diblock copolymer shown in Fig. 4 did not exhibit the melting transition of PEG blocks, suggesting that the PEG subchains in the thermosets are not crystallizable. It is proposed that the PEG subchains were miscible with aromatic amine-cured epoxy matrix and were interpenetrated with the crosslinked epoxy networks. The behavior of miscibility can also be evidenced by the depression of the glass temperatures (Tg) of epoxy matrix.
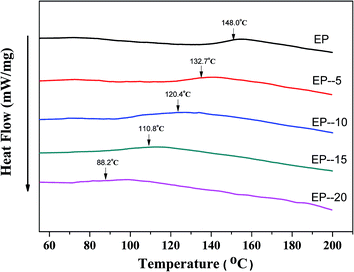 |
| Fig. 4 The DSC curves of epoxy and the nanostructured epoxy thermosets. | |
It is seen that the Tg of the nanostructured thermosets are decreased with increasing the concentration of the diblock copolymer. The decreased Tg is responsible for the plasticization effect of the PEG blocks on the epoxy matrix and the lower glass transition temperature of CTBN blocks. The DSC results indicate that the epoxy thermosets containing the diblock copolymer are microphase-separated.
3.4 Dynamic mechanical properties (DMA)
For comparison, the nanostructured thermosets containing diblock copolymer were further subjected to dynamic mechanical analysis (DMA). Dynamic mechanical loss factor (tan
δ) versus temperature of neat epoxy resin, and representative modified composites of 5, 10, 15 and 20 wt% of PEG-b-CTBN are depicted in Fig. 5. The MOCA-cured pure epoxy exhibited a well-defined major relaxation peak centered at 170.7 °C, which is responsible for the glass–rubber transition of the crosslinked polymer. It is seen that adding the diblock copolymer into the thermosets, the α transition shifted to the lower temperatures and the Tg of epoxy matrix decreased with increasing the content of the diblock copolymer. The decreased Tg is ascribed to the plasticization of PEG subchains of the block copolymer on the epoxy matrix and the lower Tg of rubber. Besides, the width of peaks (tan
δ = 0.2) became wider by adding the diblock copolymer.
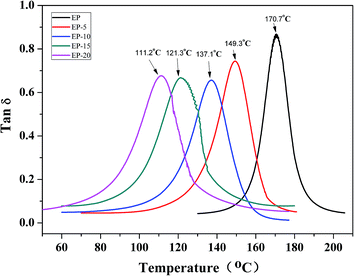 |
| Fig. 5 The dynamic mechanical spectra of epoxy and the nanostructured thermosets. | |
3.5 Mechanical properties
The tensile testing of modified epoxy resins on the diblock copolymer content is shown in Fig. 6. The curve first rises and then emergences a platform. Generally, due to the soft nature of rubber, addition of a rubber modifier to a thermoset resin enhances the elongation at break dramatically, on the contrary, the tensile strength decreases sharply. But it easy to observe that the tensile strength and the elongation at break both increased compared with that of the pure epoxy in this study.
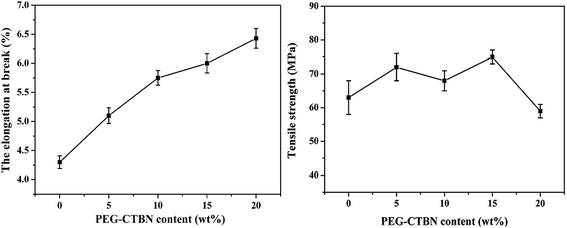 |
| Fig. 6 Tensile properties of epoxy and the nanostructured thermosets. | |
The explanation could elaborate as below. The addition of soft phase into brittle epoxy resin would reduce residual internal stresses in cured epoxy systems.13 And PEG-b-CTBN was homogenously dispersed in the epoxy resin matrix at the nanometer scale, which will greatly optimize the interactions between the matrix and the modifier. All of these positive factors lead to an enhanced strength. On the other hand, due to the low strength of soft CTBN phases, these negative elements would decrease the tensile strength.14 When the content was further increased, the contribution by the positive and negative factors achieves a balance.
The impact strength of the nanostructured thermosets containing PEG-b-CTBN triblock copolymer was evaluated by Izod Impact Tester. The impact strength of the neat and the diblock copolymer modified epoxy resins was presented in Fig. 7. The result has a similar tendency with tensile strength and can be explained by the fractured surfaces after impact testing. Fractured surfaces of the unmodified and diblock copolymer modified epoxy resins after impact testing are examined by SEM, and the results of SEM are shown in Fig. 8. The smooth fracture surfaces of the unmodified epoxy resin exhibit the typical characteristics of brittle fracture (Fig. 8a), which is ascribe to its relatively poor resistance. With increase the content of diblock copolymer, white lines on the surface were observed (Fig. 8b–d), which corresponds to the improved fracture toughness. The results of SEM has a great agreement of the results of impact strength.
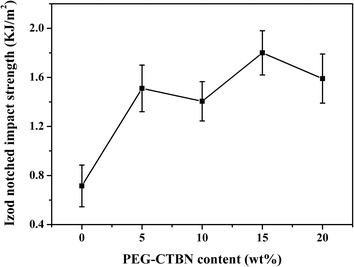 |
| Fig. 7 Impact properties of epoxy and the nanostructured thermosets. | |
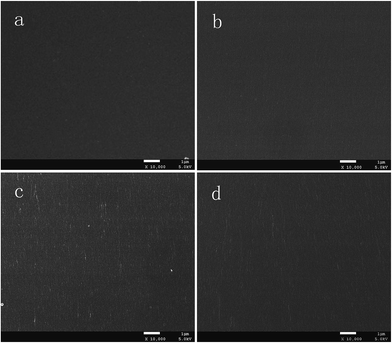 |
| Fig. 8 SEM images of the fracture surfaces of epoxy and the nanostructured thermosets [(a) 0 wt%; (b) 5 wt%; (c) 15 wt%; (d) 20 wt% PEG-b-CTBN diblock copolymer]. | |
The critical-stress-intensity factor KIC of the composites as a function of the PEG–CTBN content is shown in Fig. 9. It is seen that the KIC values of all the composites are higher than that of the neat epoxy, indicating that the epoxy thermoset was significantly toughened with the introduction of PEG–CTBN. The values of KIC increased with increasing the content of PEG–CTBN. The value of KIC attains its maximum (1.61 MN m−3/2) while the concentration of PEG–CTBN is 15 wt%. The KIC value is 1.5 times that of the neat epoxy (1.08 MN m−3/2).
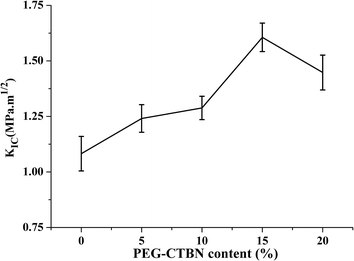 |
| Fig. 9 Plots of KIC as function of modifiers for the nanostructured epoxy thermosets containing PEG–CTBN copolymer. | |
The toughening mechanisms responsible for the increase of the fracture toughness of epoxy resins by rubber have been reported preciously in detail.4,15–19 The pioneers revealed that most of the toughening mechanisms are available for micro-sized rubber toughened epoxy resins and the mechanisms are rubber-size dependent.20,21 The crack-tip shear yielding argument suggests that shear yielding should be the dominant toughing mechanism when the size of the rubber particles is smaller than the size of the plastic zone.4,20,21 And plastic shear yielding near the crack tip is considered to be the main source of energy dissipation for modified epoxy resins by rubber particle smaller than 200 nm.21 In this study, the size of the diblock copolymer is about 10 nm. The crack tip was blunted by the shear yielded deformation, which bring about the reduction of stress concentration near the crack tip and block the crack propagation. Consequently the fracture toughness was improved.
Compared to the thermosets modified with the liquid rubber, the toughness improvement of the thermosets via the formation of the nanostructures could display the following advantage. Firstly, the diblock copolymer were homogenously dispersed in the epoxy resin matrix at the nanometer scale, which will greatly optimize the interactions between the matrix and the modifier. Secondly, because of the appearance of hydrogen bond between PEG and the secondary hydroxyl groups of MOCA-cured epoxy resins, the toughness effects is better. Finally, achieving the same effects, the amount of diblock copolymer is smaller than the amount of rubber particles or liquid rubber.
4 Conclusions
In this investigation, the diblock copolymer was synthesized and used to modify DGEBA epoxy resin. Nanostructure thermosetting composites was prepared through reaction-induced microphase separation own to the difference in miscibility of carboxyl terminated butadiene-acrylonitrile rubber (CTBN) and polyethylene glycols blocks with epoxy resin after and before curing reaction. The effect of adding diblock copolymer on the properties of epoxy resin was investigated. The result of SEM shows that the diblock copolymer were homogeneously dispersed in the epoxy matrix on nano-scale. Thermal properties analysis showed that the Tg decreased with increasing the content of diblock copolymer, which is responsible for the plasticization effect of the PEG blocks on the epoxy matrix and the addition of soft CTBN blocks. The test of mechanical properties and fracture toughness indicate the tensile strength and fracture toughness of the composites were simultaneously enhanced by nanostructured PEG-b-CTBN compared with that of the pure epoxy. The enhancements of strength and toughness were attributed to the reduction of residual internal stresses produced during curing of epoxy matrix, the increase of interaction between epoxy and modifier, the miscibility of PEG blocks, and the blockage of crack propagation during fracture by homogeneously dispersed soft CTBN phases in epoxy matrix.
Acknowledgements
The authors would like to thank the National Natural Science Foundation of China (51273118), the Science & Technology Pillar Program of Sichuan (2013FZ0006) and the Fundamental Research Funds for the Central Universities of China (2015SCU11008) for financial support, and the Analytical and Testing Center of Sichuan University for providing SEM measurements.
Reference
- M. Dadfar and F. Ghadami, Effect of rubber modification on fracture toughness properties of glass reinforced hot cured epoxy composites, Mater. Des., 2013, 47, 16–20 CrossRef CAS PubMed
. - S. Chaudhary, et al., Amine-functionalized poly(styrene) microspheres as thermoplastic toughener for epoxy resin, Polym. Compos., 2015, 36, 174–183 CrossRef CAS PubMed
. - M. Zamanian, et al., Fracture toughness of epoxy polymer modified with nanosilica particles: particle size effect, Eng. Fract. Mech., 2013, 97, 193–206 CrossRef PubMed
. - L. Ruiz-Pérez, et al., Toughening by nanostructure, Polymer, 2008, 49(21), 4475–4488 CrossRef PubMed
. - Y. Zhao, et al., Simultaneously enhanced cryogenic tensile strength and fracture toughness of epoxy resins by carboxylic nitrile-butadiene nano-rubber, Composites, Part A, 2013, 55, 178–187 CrossRef CAS PubMed
. - L.-C. Tang, et al., Mechanical properties and fracture behaviors of epoxy composites with multi-scale rubber particles, Mater. Chem. Phys., 2013, 141(1), 333–342 CrossRef CAS PubMed
. - M. Blanco, et al., Nanostructured thermosets from self-assembled amphiphilic block copolymer/epoxy resin mixtures: effect of copolymer content on nanostructures, Polym. Int., 2010, 59(4), 523–528 CrossRef CAS PubMed
. - M. A. Hillmyer, et al., Self-assembly and polymerization of epoxy resin-amphiphilic block copolymer nanocomposites, J. Am. Chem. Soc., 1997, 119(11), 2749–2750 CrossRef CAS
. - W. Fan, L. Wang and S. Zheng, Double Reaction-induced Microphase Separation in Epoxy Resin Containing Polystyrene-block-poly(ε-caprolactone)-block-poly(n-butyl acrylate) ABC Triblock Copolymer, Macromolecules, 2010, 43(24), 10600–10611 CrossRef CAS
. - R. Yu, et al., Reaction-Induced Microphase Separation in Epoxy Thermosets Containing Block Copolymers Composed of Polystyrene and Poly(ε-caprolactone): Influence of Copolymer Architectures on Formation of Nanophases, Macromolecules, 2012, 45(22), 9155–9168 CrossRef CAS
. - Q. Guo, et al., Miscibility, crystallization kinetics and real-time small-angle X-ray scattering investigation of the semicrystalline morphology in thermosetting polymer blends of epoxy resin and poly(ethylene oxide), Polymer, 2001, 42(9), 4127–4140 CrossRef CAS
. - K. Yamanaka, Y. Takagi and T. Inoue, Reaction-induced phase separation in rubber-modified epoxy resins, Polymer, 1989, 30(10), 1839–1844 CrossRef CAS
. - G. Yang, S.-Y. Fu and J.-P. Yang, Preparation and mechanical properties of modified epoxy resins with flexible diamines, Polymer, 2007, 48(1), 302–310 CrossRef CAS PubMed
. - N. Chikhi, S. Fellahi and M. Bakar, Modification of epoxy resin using reactive liquid (ATBN) rubber, Eur. Polym. J., 2002, 38(2), 251–264 CrossRef CAS
. - S.-Y. Fu, et al., Effects of particle size, particle/matrix interface adhesion and particle loading on mechanical properties of particulate–polymer composites, Composites, Part B, 2008, 39(6), 933–961 CrossRef PubMed
. - J. M. Dean, et al., Nanostructure toughened epoxy resins, Macromolecules, 2003, 36(25), 9267–9270 CrossRef CAS
. - A. C. Garg and Y.-W. Mai, Failure mechanisms in toughened epoxy resins—A review, Compos. Sci. Technol., 1988, 31(3), 179–223 CrossRef CAS
. - R. Bagheri and R. A. Pearson, Role of particle cavitation in rubber-toughened epoxies: 1. Microvoid toughening, Polymer, 1996, 37(20), 4529–4538 CrossRef CAS
. - R. Bagheri and R. A. Pearson, Role of particle cavitation in rubber-toughened epoxies: II. Inter-particle distance, Polymer, 2000, 41(1), 269–276 CrossRef CAS
. - R. Pearson and A. Yee, Toughening mechanisms in elastomer-modified epoxies, J. Mater. Sci., 1989, 24(7), 2571–2580 CrossRef CAS
. - H. Azimi, R. Pearson and R. Hertzberg, Fatigue of rubber-modified epoxies: effect of particle size and volume fraction, J. Mater. Sci., 1996, 31(14), 3777–3789 CrossRef CAS
.
|
This journal is © The Royal Society of Chemistry 2015 |