DOI:
10.1039/C5RA05452D
(Paper)
RSC Adv., 2015,
5, 59472-59479
Bacillus subtilis-based colorimetric bioassay for acute biotoxicity assessment of heavy metal ions
Received
27th March 2015
, Accepted 22nd June 2015
First published on 23rd June 2015
Abstract
This paper described a novel colorimetric bioassay based on Bacillus subtilis for acute biotoxicity assessment of heavy metal ions and real water samples. β-Galactosidase, which can catalyze the hydrolysis of o-nitrophenyl-β-D-galactopyranoside (ONPG) to produce galactose and o-nitrophenol (ONP), can be generated from Bacillus subtilis (CGMCC 1.1086) without the assistance of any inducers. When heavy metal ions exist, the activity of β-galactosidase is inhibited due to the reaction between the sulfhydryl groups in β-galactosidase and the heavy metal ions. Accordingly, the output of the ONP, a yellow chromogenic compound exhibiting a characteristic absorption peak at 420 nm, is also inhibited. Thus, acute biotoxicity of heavy metal ions can be evaluated by assessing the absorption intensity of ONP at 420 nm. To obtain an ideal colorimetric performance, working parameters such as Bacillus subtilis concentration, temperature and incubation time of the bioassay were studied and optimized. Under the optimized parameters, the acute biotoxicity of five heavy metal ions (Cu2+, Zn2+, Ni2+, Cd2+, Pb2+) and three real samples were evaluated. All the results suggest that Bacillus subtilis-based bioassay is a sensitive, economic, simple and promising alternative for acute biotoxicity assessment.
1. Introduction
Heavy metal ions are being released to the environment through human activities including mining operation, metal plating, electronic waste and the use of fertilizers. Due to their high aqueous solubility, heavy metal ions can leach into groundwater easily, which threaten people's health through bioaccumulation or direct toxicity. According to the estimation from the World Health Organization,1 by the end of 2011, almost 693 million people had suffered from drinking unsafe water with heavy metal ions as main pollutants. Therefore, assessing biotoxicity of heavy metal ions is of high priority for the healthcare need and the environmental monitoring. In the past decades, a large number of protocols have been proposed for the acute biotoxicity assessment. Traditional acute biotoxicity assessing methods usually use higher organisms such as mice,2 plants,3 daphnia,4 fish.5 However, their practical applications in emergent condition are hindered due to the high cost, long time and potential ethical responsibilities of these methods. Therefore, developing a rapid and economic method for acute biotoxicity assessment is necessary.
The ubiquitous microorganisms offering an alternative for addressing these limitations because of their short life cycle, rapid response to toxins and low cost.6 Current bacteria-based acute biotoxicity methodologies can be sub-divided into two main approaches, electrochemical methods and bioluminescent methods. Electrochemical methods rely on redox mediators, such as potassium hexacyanoferrate6,7 and benzoquinone.8,9 These mediators replace oxygen as the terminal electron acceptor in the respiration pathway of the bacteria, and the reduced mediators can be oxidized at the surface of the electrode generating electrochemical oxidation current.10 While toxic substances inhibit the respiration of bacteria, mediator reduction process will be restrained, leading to less reduced mediators, which finally cause the decrease of oxidation current. As a result, acute biotoxicity can be evaluated by measuring the decline degree of the oxidation current. However, electrochemical methods have some big flaws, including low sensitivity and the tedious process of electrode fabrication. Moreover, mediators itself at high concentration also have a certain toxicity to the bacteria, which may make some interferences on the final experiment results.11 Bioluminescent methods are mainly based on saltwater bioluminescent bacteria, such as Vibrio fischeri12,13 and Photobacterium phosphoreum.14,15 For Vibrio fischeri, when the toxic materials exist, the bioluminescence produced by respiration of the Vibrio fischeri fade due to the acute biotoxicity of the toxic materials. Therefore, the acute biotoxicity can be evaluated by detecting the decline degree of the bioluminescence intensity. It is also the mechanism of Mircotox® system,16 a successful commercialized toxicity assessing system. However, luminous bacteria are not only rare, but also expensive, and it must work in 3% saline solution, limiting their practical application to a certain extent.
β-Galactosidase existed in E. coli is commonly recognized as an inducible enzyme. It is well known that heavy metals ions, such as Cu2+, Zn2+, Ni2+, Cd2+, Pb2+, are potent irreversible inhibitors of β-galactosidase due to the reaction between the heavy metal ions and sulfhydryl group existed in β-galactosidase. However, almost all the reported E. coli-based bioassays based on β-galactosidase need a complex and time-consuming inducing process.17,18 Moreover, isopropyl-β-D-thiogalactopyranoside (IPTG), the widely used inducer, is expensive19 and toxic to bacteria20 and humans.21 There are few reports on using other bacteria that can produce β-galactosidase to assess acute biotoxicity of environmental pollutants.
Bacillus subtilis is a model of Gram-positive bacteria22 and its envelope structure is much simpler than that of Gram-negative bacteria (such as E. coli), rendering it more sensitive to toxic substances.18 In addition, it is reported that, comparing with the β-galactosidase from E. coli, the β-galactosidase coming from Bacillus subtilis is more heat stable and more resistant to some ions such as NH4+, Ca2+, Cl−.23 Furthermore, Bacillus subtilis, which is generally recognized as a kind of safe and low-cost bacteria, has been widely used in various fields, such as laboratory studies, fermentation industry,24,25 food26 and medicine.27
Herein, the Bacillus subtilis-based bioassay was fabricated to assess acute biotoxicity of heavy metal ions. In this bioassay system, β-galactosidase could be directly generated from the Bacillus subtilis (CGMCC 1.1086) without the assistance of any β-galactosidase inducers, which simplified the fabrication procedure greatly and saved the cost. The working parameters such as cell concentration, temperature and incubation time were studied in detail and optimized to obtain good colorimetric performances. The optimized Bacillus subtilis-based bioassay was finally used to assess acute biotoxicity of five heavy metal ions (Cu2+, Zn2+, Ni2+, Cd2+, Pb2+) and three practical water samples, including effluent from landfill, electroplating wastewater and inorganic wastewater from chemical laboratory. All the results suggested that Bacillus subtilis-based bioassay was a sensitive, economic, simple and promising alternative for acute biotoxicity assessment.
2. Experimental
2.1 Chemicals and reagents
Bacillus subtilis (CGMCC 1.1086) was obtained from China General Microbiological Culture Collection Center (CGMCC). Pb(NO3)3, CuSO4·5H2O, ZnSO4·7H2O, Ni(NO3)2·6H2O, Cd(NO3)2, NaCl, NaOH, Na2CO3, chloroform, sodium dodecyl sulfonate (SDS) (analytical grade) were provided by Beijing Lanyi Chemical Products Co., Ltd., China. Peptone and beef extract were obtained from Beijing Aoboxing Bio-tech Co., Ltd., China. o-Nitrophenyl-β-D-galactopyranoside (ONPG) was purchased from Alfa Aesar. All reagents were used as received without further purification. All solutions were prepared with deionized water (18.0 MΩ cm, Milli-Q Gradient System, Millipore).
The culture medium (10 g L−1 peptone, 3 g L−1 beef extract, 5 g L−1 NaCl) for Bacillus subtilis was adjusted to pH = 7.0–7.3 with NaOH (2 mol L−1) and sterilized by autoclaving. ONPG (4 mg mL−1) and all toxicants (Cu2+, Zn2+, Cd2+, Pb2+ and Ni2+) were freshly prepared before use. Effluents from landfill and electroplating wastewater were provided by Technical Institute of Physical and Chemistry, Chinese Academy of Sciences. Inorganic wastewater was taken from our chemical laboratory.
2.2 Composition determination of real water samples
The analysis of heavy metal ions in three water samples (effluents from landfill, inorganic wastewater from chemical laboratory and electroplating wastewater) were performed by inductively coupled plasma atomic emission spectrometry (ICP-AES) (Varian 710-ES ICP Optical Emission Spectrometer). The chemical oxygen demand (COD) values of these three samples were measured by the closed reflux colorimetric method with a spectrophotometer (HACH DRB 200).
2.3 Cultivation of microorganism
Bacillus subtilis was maintained on nutrient agar plate at 4 °C and replanted regularly to ensure its viability. A 300 mL flask containing 100 mL medium was inoculated from an agar plate, and grown aerobically at 37 °C on a rotary shaker at 160 rpm for 24 h to allow the Bacillus subtilis grow into its stationary phase. Bacterial cells were harvested by centrifuging at 6000 rpm for 5 min at room temperature, then washed twice with 0.85% (w/v) saline solution and finally suspended in saline solution. Optical density at 600 nm (OD600) of cell suspension was adjusted with saline solution and measured by SECOMAM UVIKONXL UV-vis spectrophotometer at 600 nm. The bacterial suspension was kept at 4 °C for less than 3 h until cells were used for assaying.
2.4 Acute biotoxicity assessment
Firstly, 350 μL of cell suspension and 50 μL of certain heavy metal ion solutions with various concentrations were added separately to the microcentrifuge tubes (experimental group). As comparison, reference incubation (control group) contained saline solution instead of 50 μL of toxicant. The obtained suspensions were mixed thoroughly and then placed in the thermostat incubator at 37 °C for a period of time (denoted as t1). Then 50 μL of ONPG solution (4 mg mL−1), 50 μL of SDS solution (0.1% w/v) and 30 μL of chloroform were introduced to each tube. The suspensions obtained were mixed well and placed in thermostat incubator at 37 °C for another period of time (denoted as t2) to make the ONPG hydrolyse to yellow o-nitrophenol (ONP), a kind of toxicity indicator used here. Finally, 400 μL of Na2CO3 solution (1 M) were added to each tube to terminate the hydrolysis reaction. The final suspensions were centrifuged at 12
000 rpm for 5 min, and the absorbance of obtained supernatant solutions were measured at 420 nm for detection of content of the ONP, the toxicity indicator, to evaluate the biological toxicity of the added heavy metal ions.
2.5 Data analysis
The biotoxicity of heavy metal ions to Bacillus subtilis is that heavy metal ions can inhibit the activity of β-galactosidase due to the reaction between the sulfhydryl group existed in β-galactosidase and the heavy metal ions. The degree of inhibition was determined by measuring the ONP absorbance values at 420 nm, the absorbance was then converted to equivalent inhibitory percentage using eqn (1). |
Inhibition (%)= (1 − absexp/abscon) × 100%
| (1) |
here, abscon is the absorbance of control group, while the absexp is the absorbance of sample.
Inhibition curves were plotted in terms of inhibition percent versus various final toxicant concentrations. The half maximal inhibitory concentration (IC50) was derived from the inhibition curve by interpolation method under the assumption that any adjacent two points conformed to the linear relationship. The each entry represented the mean of three determinations.
3. Results and discussion
3.1 Feasibility of Bacillus subtilis-based bioassay to assess acute biotoxicity
Although the whole-cell bioassays based on β-galactosidase for the acute biotoxicity assessment have been studied for many years, the bacteria are almost confined to E. coli.17,18,28 Moreover, in the reported studies, the inducers such as IPTG or lactose were usually added to the culture to induce the production of β-galactosidase. In present research, we have investigated the feasibility of replacing the E. coli by Bacillus subtilis, a Gram-positive bacteria, to assess the acute biotoxicity of heavy metal ions without the assistance of any β-galactosidase inducers, and ONPG, a chromogenic substrate, can be hydrolysed by using the β-galactosidase as catalyst to form ONP, a yellow chromogenic compound, exhibiting a characteristic absorption peak at 420 nm.29,30 The ONP can be used as the toxicity indicator to evaluate the biotoxicity of the introduced heavy metal ions (Scheme 1).
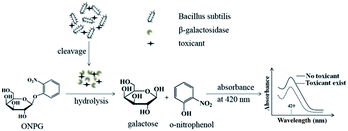 |
| Scheme 1 Conceptual schematic representation of using Bacillus subtilis-based bioassay to assess acute biotoxicity. | |
The presence of toxicants, especially heavy metal ions, can inhibit the activity of β-galactosidase due to the reaction between the sulfhydryl groups in β-galactosidase and the heavy metal ions, leading to the output of ONP will also be inhibited. Hence, a decline of absorbance at 420 nm can be observed. Therefore, according to the above discussion, if β-galactosidase can be generated from Bacillus subtilis without the assistance of any inducers, we can evaluate acute biotoxicity by using Bacillus subtilis directly.
Initial studies involved the feasibility of using Bacillus subtilis-based bioassay to assess acute biotoxicity of heavy metal ions. Fig. 1 shows the responses of Bacillus subtilis-based bioassay to Cu2+, a target toxicant. As shown in Fig. 1A, when no Cu2+ existed, supernatant solution showed deep yellow, suggesting that ONPG was hydrolysed to yellow ONP under the enzyme catalysis of β-galactosidase.31,32 It is worth pointing out that no extra pure β-galactosidase was added to this system during the experiment, consequently, the β-galactosidase appeared in present experiment must be generated from the Bacillus subtilis cells. As shown in Fig. 1B, with the concentration of Cu2+ increased from 0 to 50 mg L−1, the colors of supernatant solutions faded gradually from deep yellow to nearly colourless, and the absorbance (at 420 nm) of corresponding supernatant solutions decreased from 0.46 to 0.07, revealing that Cu2+ inhibited the activity of the β-galactosidase. Therefore, the present result clearly suggested that evaluating the acute biotoxicity of Cu2+ ions was feasible by using this Bacillus subtilis-based bioassay.
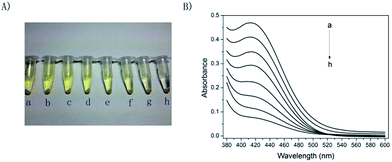 |
| Fig. 1 (A) Colors fading of supernatant solutions with the addition of Cu2+, the Cu2+ concentrations from a to h: 0, 5, 10, 15, 20, 30, 40, 50 mg L−1, respectively. (B) The corresponding absorption curve of supernatant solutions from a to h. | |
Based on the above results, we could conclude that: (1) Bacillus subtilis (CGMCC 1.1086) could generate β-galactosidase without the assistance of any inducers; (2) Bacillus subtilis-based bioassay was sensitive to Cu2+ ion, and for that reason, it was feasible to apply Bacillus subtilis-based bioassay to assess acute biotoxicity of some other heavy metal ions. Scheme 1 shows the principle of Bacillus subtilis-based bioassay to evaluate acute biotoxicity.
3.2 Performance of the Bacillus subtilis-based bioassay
The parameter optimization of the bioassay in terms of Bacillus subtilis concentration, temperature and time for colour development were studied in order to obtain a better detection results for a variety of heavy metal ions.
3.2.1 Effect of bacterial concentration. In most microorganism-related biosensors, bacterial concentration is an important parameter, since it directly influence the sensitivity of the biosensor and reproducibility of signal collection.33,34 Optical density at 600 nm (OD600) has been widely used to indicate the concentration of bacterial cell suspension. The larger value of the OD600 means the higher concentration of the cell suspension.35,36 In this study, the OD600 was also selected to indicate the bacterial concentration, and the effect of bacterial concentration was investigated to obtain an optimized colorimetric performance.Fig. 2 is the dose–response inhibition curves obtained by adding series concentrations of the Cu2+ into the solutions of different Bacillus subtilis concentrations. As shown in Fig. 2, in the range of the Cu2+ added from 0 to 40 mg L−1, the response inhibition increased with the reduction of bacterial concentrations from OD600 = 3.0 (∼1.8 × 1012 CFU mL−1), 2.7 (∼1.1 × 1012 CFU mL−1) to 2.4 (∼1.5 × 1011 CFU mL−1); but a slight decline of inhibition was observed with the further reduction of bacterial concentration from OD600 = 2.4 to OD600 = 2.1 (∼1.1 × 1011 CFU mL−1). Accordingly, as shown in the insert graph, with the reduction of bacterial concentration, the IC50 values decreased from 16.9 mg L−1 (OD600 = 3.0) to 7.4 mg L−1 (OD600 = 2.4) and then increased to 8.3 mg L−1 (OD600 = 2.1), revealing that the bioassay fabricated with the bacterial concentration of OD600 = 2.4 became most sensitive to Cu2+. Therefore, we adopted the bacterial concentration of OD600 = 2.4 as the final concentration, which is enough to obtain a sufficient response.
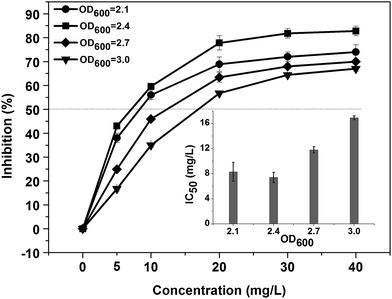 |
| Fig. 2 Effect of Bacillus subtilis concentrations on the biotoxicity assessment of Cu2+. At a certain OD600, a series concentrations of Cu2+ were assessed to obtain the inhibition value with T = 37 °C, t1 = 30 min, t2 = 30 min. The inset graph showed the IC50 values obtained at different bacterial concentration. Each entry of represented the mean of three determinations. | |
3.2.2 Effect of temperature. Since most of bacteria and enzymes are sensitive to temperature (T), it is essential to study the influence of temperature on Bacillus subtilis-based bioassay. The influence of T on acute biotoxicity assessment of Cu2+ is shown in Fig. 3. As can be observed, in a fixed Cu2+ concentration, inhibitions caused by Cu2+ became greater with the increase of T, suggesting that the performance of Bacillus subtilis-based bioassay was sensitive to temperature. At room temperature (25 °C), all the inhibition effects were lower than 30% when concentrations of Cu2+ ranged between 0 to 40 mg L−1. However, inhibition effects engendered by Cu2+ concentration at 10 mg L−1 raised to 30% and 60% when T at 30 °C and 37 °C, respectively. IC50 values were 7.4 mg L−1 at 37 °C, 25 mg L−1 at 30 °C, and more than 40 mg L−1 at 25 °C, i.e., the highest inhibition was obtained at 37 °C. Therefore, 37 °C was chosen as the optimal temperature for the next acute biotoxicity assessment.
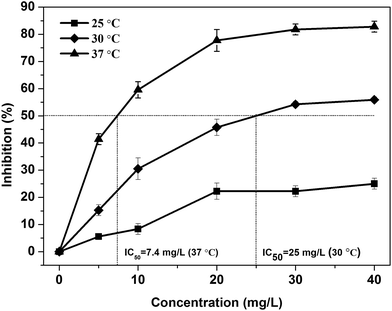 |
| Fig. 3 Effect of temperature on the acute biotoxicity assessment of Cu2+ under the condition that OD600 = 2.4, t1 = 30 min, t2 = 30 min. Each entry of inhibition represented the mean of three determinations. | |
3.2.3 Effect of incubation time. For the emergency evaluation, the required testing time is an important factor which should be taken into consideration. Incubation for t1 provided the time for toxicants to fully mix and react with Bacillus subtilis. In this period, toxicants inhibited β-galactosidase in Bacillus subtilis cells directly. Incubation for t2 aimed to ensure the inhibited β-galactosidase leave from the cleaved bacterial cells, and further react with ONPG thoroughly. Fig. 4 shows the influence of incubation time t1 and t2 under the optimal experimental conditions (OD600 = 2.4, T = 37 °C) that have been discussed above.
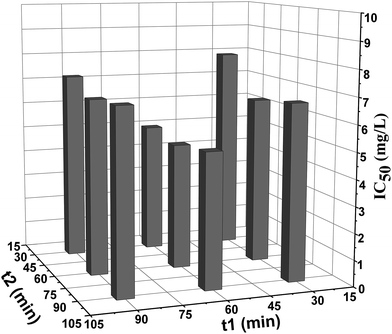 |
| Fig. 4 Effect of t1 and t2 on the acute biotoxicity assessment of Cu2+. OD600 = 2.4, T = 37 °C. | |
It can be observed that, when t2 kept constant and t1 increased, IC50 values decreased firstly and then increased. And this tendency was also observed when t1 were kept constant and t2 increased. This change tendency could be explained by the two contrary effects resulted from the prolongation of incubation time: on the one hand, toxic effect accumulated with the increase of incubation time, the sensitivity of the bioassay was improved and a lower IC50 values could be observed; on the other hand, the intrinsic activity of Bacillus subtilis or β-galactosidase would decrease with the prolongation of time, as a result, the toxic effect could not be fully reflected and sensitivity of the bioassay declined with the incubation time increasing. Considering the rapidity requirement for emergency assessment and sensitivity of the bioassay, t1 = 60 min and t2 = 30 min were the proper combination time.
Therefore, according to the above discussing, the optimal parameters for evaluate toxicity of Cu2+ were OD = 2.4, T = 37 °C, t1 = 60 min and t2 = 30 min. And these optimal parameters were employed for acute biotoxicity assessment of other heavy metal ions and real wastewater samples.
4. Acute biotoxicity assessment of heavy metal ions by Bacillus subtilis-based bioassay
Five typical heavy metal ions (Cu2+, Zn2+, Cd2+, Ni2+ and Pb2+) were investigated under the optimal experimental conditions. The responses of Bacillus subtilis-based bioassay are shown in Fig. 5. As shown in Fig. 5, for all the metal ions studied, at lower metal ions concentrations, the inhibitions rise rapidly; with the increase of heavy metal concentration, the inhibitions tended slowly. The calculated IC50 values for Cu2+, Zn2+, Cd2+, Ni2+ and Pb2+ were 5 mg L−1, 9.3 mg L−1, 27.6 mg L−1, 21.3 mg L−1 and 101.7 mg L−1, respectively.
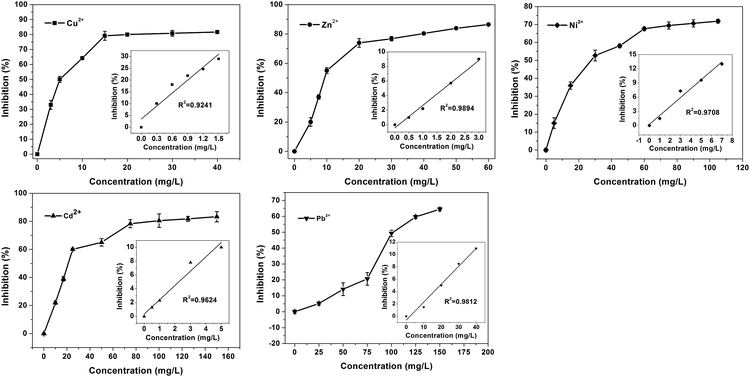 |
| Fig. 5 Responses of Bacillus subtilis-based bioassay to different heavy metal ions with various concentrations. OD600 ≈ 2.4, T = 37 °C, t1 = 60 min, t2 = 30 min. The each entry represented the mean of three determinations. | |
Furthermore, the comparisons between our Bacillus subtilis-based bioassay to other methods are shown in Table 1. Obviously, the Bacillus subtilis-based bioassay is more sensitive than the reported electrochemical methods.11,40,41 Moreover, it is comparable or even better than the reported toxicity bioassays based on β-galactosidase which generated by E. coli with the assistance of inducers.17,18 This sensitivity improvement might result from the fact that Bacillus subtilis possess comparative simpler cell membrane structure than E. coli, toxic substances can diffuse into the cell of Bacillus subtilis more easily and cause more sensitive inhibition effect. Other enzymes from Bacillus subtilis, such as α-amylase, was also used for toxicity assessment.18 However, the time for toxicity assessment basing on α-amylase was at least eight hours, which was too time-consuming. Furthermore, as shown in Table 1, for toxicity assessment of Cu2+ and Zn2+, the sensitivity of α-amylase was less than β-galactosidase. On the other hand, the acute biotoxicity order of these five heavy metal ions obtained in present experiment is Cu2+ > Zn2+ > Cd2+ > Ni2+ > Pb2+, which is in agreement with the results obtained by Microtox® method, and also consistent with the affinity of heavy metal ions toward the sulfhydryl group.37,38 All the results suggested that the our bioassay would be a promising alternative for acute biotoxicity assessment.
Table 1 Comparison of IC50 values by Bacillus subtilis-based bioassay with other methods
Toxicity assays |
Toxicants, IC50 (mg L−1) |
References |
Cu2+ |
Zn2+ |
Ni2+ |
Cd2+ |
Pb2+ |
Represents the 20% inhibitory concentration. |
Bacillus subtilis-based bioassay |
5 |
9.3 |
27.6 |
21.3 |
101.7 |
Present study |
Amperometry, E. coli |
>150 |
— |
>150 |
— |
>150 |
11 |
Amperometry, Psychrobacter sp. |
2.6 |
10.9 |
— |
47.3 |
101.1 |
39 |
Amperometry, E. coli |
44 |
— |
— |
79 |
— |
40 |
β-Galactosidase biosynthesis, E. coli |
— |
25a |
— |
15a |
— |
17 |
β-Galactosidase biosynthesis, E. coli |
34.1 |
21 |
— |
16.7 |
— |
18 |
α-Amylase biosynthesis, B. subtilis |
6.9 |
15.5 |
— |
14.5 |
— |
18 |
Nitrification inhibition, nitrifying bacteria |
41.5 |
22.6 |
— |
33.1 |
— |
41 |
Microtox®, Vibrio fischeri |
0.397 |
10.5 |
— |
56.8 |
34.6 |
42 |
It is worth noting that β-galactosidase has been used for rapid quantitative detection of heavy metal ions in the water body and the detection limit can reach ppb region.37 In our work, although the β-galactosidase (Bacillus subtilis)-based biosensor was also used as the study object, the researching purposes of the two kinds of works are totally different. The former is detecting the concentration of the heavy metals analytically, while our purpose is to evaluate the total biological toxicity of the water by using the IC50 as toxicity index to provide an early warning and response. IC50, a toxicity index that has been widely used to indicate the toxicity of a substance in toxicology, is neither the concentration value of the solution, nor the value of the detection limit. In a word, IC50 is an index that was used to evaluate the level of toxicity of some poisons.
Although the main purpose of our research was assessing the acute biotoxicity of heavy metal ions, the limits of detection (LODs) of these five heavy metal ions based on the dose–response curves were also investigated. The dose–response curve of each heavy metal ion in low concentration was linear fitted, as shown in the inset of Fig. 5. The LODs were calculated according to the equation: limit of detection = 3σb/s, where σb is the standard deviation of blank measures, s is the slope between inhibition versus heavy metal ion concentration.43 The LODs of Cu2+, Zn2+, Cd2+, Ni2+ and Pb2+ were 0.007 mg L−1, 0.043 mg L−1, 0.07 mg L−1, 0.05 mg L−1 and 0.3 mg L−1, respectively. The LODs of these five heavy metal ions obtained by our method were compared with that of other approaches, and the results were shown in Table 2. It is obvious that all the ICP,45 electrochemical methods46,47 and fluorescent methods48,49 show high sensitivity to heavy metal ions detection and meet the concentration limits of heavy metal ions in drinking water provided by WHO.44 It should be noted that the LODs of our method is comparable with that obtained by paper-based pure β-galactosidase bioassay.37 However, apart from the Cu2+ detection, the LODs of other four heavy metal ions of our method were much higher than the WHO standard. The gap between the LODs of our method and limits of drinking water result from the fact that the purpose of our research is assessing the acute biotoxicity of heavy metal ions rather than detect them analytically. In a word, our purpose was to assess biological toxicity of the target water (usually polluted water), rather than evaluating the concentration of the poison (such as heavy metal ions) in pure water or drinking water. Moreover, the acute toxicity normally refers to the toxicity caused by high concentrations of toxicants in a short period of time,50 which is suitable for our method. While for the detection of trace elements in clean water, our method is not applicable.
Table 2 Comparison of limit of detection values by Bacillus subtilis-based bioassay for Cu2+, Zn2+, Ni2+, Cd2+ and Pb2+ with other methods
Methods |
Limit of detection (μg L−1) |
References |
Cu2+ |
Zn2+ |
Ni2+ |
Cd2+ |
Pb2+ |
Colorimetric, Bacillus subtlis (β-galactosidase) |
7 |
43 |
70 |
50 |
300 |
Present study |
Guidelines for drinking-water quality, WHO |
2000 |
— |
70 |
3 |
10 |
44 |
Inductively coupled plasma optical emission spectrometry (ICP-OES) |
0.04 |
0.05 |
001 |
0.01 |
0.34 |
45 |
Colorimetric, β-galactosidase-based paper assay |
20 |
— |
230 |
20 |
140 |
37 |
Electrochemical, MWCNTs-Nafion/Bismuth composite electrodes |
— |
— |
— |
0.025 |
0.04 |
46 |
Electrochemical, multilayer paper-based device |
— |
— |
— |
1 |
1 |
47 |
Fluorescent, graphene quantum dots–aptamer |
— |
— |
— |
— |
0.124 |
48 |
Fluorescent, genetic engineered E. coli |
— |
— |
— |
31.9 |
8.2 |
49 |
5. Evaluating acute biotoxicity of real samples
We have further evaluated the acute biotoxicity of three real water samples by using our bioassay, the practical sample including the effluent from landfill, inorganic wastewater from chemical laboratory and electroplating wastewater. As shown in Fig. 6, inhibitions caused by the three real samples were 19.5% for the inorganic wastewater from chemical laboratory, 31% for the effluent from landfill and 75.9% for electroplating wastewater, respectively. The inhibition differences should be related to the composition differences of these three real samples. As shown in Table 3, both electroplating wastewater and effluent from landfill contained much heavy metal ions and organic pollutants than laboratory wastewater, and the electroplating wastewater had more heavy metal ions. It is known that β-galactosidase is more sensitive to heavy metal ions than organic compounds.51 Thus, it is reasonable that electroplating wastewater showed the highest toxicity in the three samples, and the second one is effluent from landfill. In conclusion, the present results suggested that it was promising to use Bacillus subtilis-based bioassay for practical sample assessment.
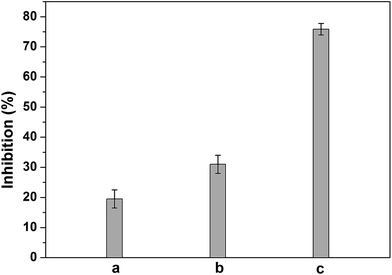 |
| Fig. 6 Acute biotoxicity evaluation of three real water samples by Bacillus subtilis-based bioassay: (a) inorganic wastewater from chemical laboratory; (b) effluent from landfill; (c) electroplating wastewater. The each entry represented the mean of three determinations. | |
Table 3 Compositions of real samples: effluent from landfill, electroplating wastewater and wastewater from chemical laboratory
Real samples |
Heavy metal ions |
COD (mg L−1) |
Ni |
Cu |
Zn |
Cr |
Effluent from landfill |
48 |
— |
— |
0.2 |
19 203 |
Electroplating wastewater |
30–80 |
30–60 |
50 |
— |
5760 |
Wastewater from chemical laboratory |
0.7 |
0.4 |
— |
— |
754 |
6. Conclusion
We present an acute biotoxicity bioassay based on the Bacillus subtilis. Acute biotoxicity of Cu2+, Zn2+, Cd2+, Ni2+ and Pb2+ were assessed under optimized conditions, and the IC50 values determined were 5 mg L−1 for Cu2+, 9.3 mg L−1 for Zn2+, 27.6 mg L−1 for Ni2+, 21.3 mg L−1 for Cd2+, 101.7 mg L−1 for Pb2+, respectively. Acute biotoxicity order of these five heavy metal ions was Cu2+ > Zn2+ > Cd2+ > Ni2+ > Pb2+. These results obtained were comparable or even better than those of other reported acute biotoxicity assays. Moreover, acute biotoxicity of three real samples were also assessed. The present bioassay offered an economic, rapid and sensitive alternative for toxicity screening of chemicals and environmental water monitoring.
Acknowledgements
The authors appreciate the supports of the Key Project of Beijing Natural Science Foundation (No. 2120002), the international Science & Technology Cooperation Program of China (Most-Japan joint project, No. 2013DFG50150), the Natural Foundation of Sciences of the People's Republic of China (No. 21175144).
Notes and references
- UNICEF, Progress on sanitation and drinking water: 2013 update, World Health Organization, NewYork, Geneva, 2013 Search PubMed.
- K.-T. Yong, W.-C. Law, R. Hu, L. Ye, L. Liu, M. T. Swihart and P. N. Prasad, Chem. Soc. Rev., 2013, 42, 1236–1250 RSC.
- S. Sharma, S. Sharma, N. Upreti and K. P. Sharma, Toxicol. Environ. Chem., 2009, 91, 109–120 CrossRef CAS PubMed.
- C. R. Janssen and G. Persoone, Environ. Toxicol. Chem., 1993, 12, 711–717 CAS.
- W. H. van der Schalie, T. R. Shedd, P. L. Knechtges and M. W. Widder, Biosens. Bioelectron., 2001, 16, 457–465 CrossRef CAS.
- K. Catterall, D. Robertson, S. Hudson, P. R. Teasdale, D. T. Welsh and R. John, Talanta, 2010, 82, 751–757 CrossRef CAS PubMed.
- N. Pasco, J. Hay and J. Webber, Biomarkers, 2001, 6, 83–89 CrossRef CAS PubMed.
- J. Li, Y. Yu, Y. Wang, J. Qian and J. Zhi, Electrochim. Acta, 2013, 97, 52–57 CrossRef CAS PubMed.
- J. Qian, J. Li, D. Fang, Y. Yu and J. Zhi, RSC Adv., 2014, 4, 55473–55482 RSC.
- K. Morris, H. J. Zhao and R. John, Aust. J. Chem., 2005, 58, 237–245 CrossRef CAS.
- C. Liu, T. Sun, Y. Zhai and S. Dong, Talanta, 2009, 78, 613–617 CrossRef CAS PubMed.
- C. X. Wang, A. Yediler, D. Lienert, Z. J. Wang and A. Kettrup, Chemosphere, 2002, 46, 339–344 CrossRef CAS.
- S. Park and K. Choi, Ecotoxicology, 2008, 17, 526–538 CrossRef CAS PubMed.
- H. Dizer, E. Wittekindt, B. Fischer and P. D. Hansen, Chemosphere, 2002, 46, 225–233 CrossRef CAS.
- M. T. Garcia, N. Gathergood and P. J. Scammells, Green Chem., 2005, 7, 9–14 RSC.
- S. Girotti, E. N. Ferri, M. G. Fumo and E. Maiolini, Anal. Chim. Acta, 2008, 608, 2–29 CrossRef CAS PubMed.
- A. Reinhartz, I. Lampert, M. Herzberg and F. Fish, Environ. Toxicol. Water. Qual., 1987, 2, 193–206 CrossRef CAS PubMed.
- K. Guven, S. Togrul, F. Uyar, S. Ozant and D. I. De Pomerai, Enzyme Microb. Technol., 2003, 32, 658–664 CrossRef CAS.
- A. Fernandez, J. Ruiz, G. Caminal and J. Lopez-Santin, Anal. Chem., 2010, 82, 5728–5734 CrossRef CAS PubMed.
- A. Marbach and K. Bettenbrock, J. Biotechnol., 2012, 157, 82–88 CrossRef CAS PubMed.
- http://www.thermoscitificbio.com/uploadedFiles/Resources/r039-product-information.pdf, accessed, 2014.
- V. Barbe, S. Cruveiller, F. Kunst, P. Lenoble, G. Meurice, A. Sekowska, D. Vallenet, T. Wang, I. Moszer, C. Medigue and A. Danchin, Microbiology, 2009, 155, 1758–1775 CrossRef CAS PubMed.
- P. J. Anema, Biochim. Biophys. Acta, Gen. Subj., 1964, 89, 495–502 CAS.
- Y. Kubo, A. P. Rooney, Y. Tsukakoshi, R. Nakagawa, H. Hasegawa and K. Kimura, Appl. Environ. Microbiol., 2011, 77, 6463–6469 CrossRef CAS PubMed.
- H. Xu, S. Jia and J. Liu, Afr. J. Biotechnol., 2011, 10, 779–788 CAS.
- A. S. Deboer and B. Diderichsen, Appl. Microbiol. Biotechnol., 1991, 36, 1–4 CrossRef CAS.
- I. B. Sorokulova, I. V. Pinchuk, M. Denayrolles, I. G. Osipova, J. M. Huang, S. M. Cutting and M. C. Urdaci, Dig. Dis. Sci., 2008, 53, 954–963 CrossRef PubMed.
- G. Cenci, G. Morozzi and G. Caldini, Bull. Environ. Contam. Toxicol., 1985, 34, 188–195 CrossRef CAS.
- E. Taqieddin and M. Amiji, Biomaterials, 2004, 25, 1937–1945 CrossRef CAS PubMed.
- H. Cieslinski, J. Kur, A. Bialkowska, I. Baran, K. Makowski and M. Turkiewicz, Protein Expression Purif., 2005, 39, 27–34 CrossRef CAS PubMed.
- J. Lederberg, J. Bacteriol., 1950, 60, 381–392 CAS.
- K. A. J. Nina Irwin, Molecular Cloning: A Laboratory Manual, Cold Spring Harbor Laboratory Press, Cold Spring Harbor, New York, 3th edn, 2001, vol. 1, pp. 1613–1797 Search PubMed.
- J. W. Choi, K. W. Park, D. B. Lee, W. Lee and W. H. Lee, Biosens. Bioelectron., 2005, 20, 2300–2305 CrossRef CAS PubMed.
- X. Tang, T. Zhang, B. Liang, D. Han, L. Zeng, C. Zheng, T. Li, M. Wei and A. Liu, Biosens. Bioelectron., 2014, 60, 137–142 CrossRef CAS PubMed.
- K. Francois, F. Devlieghere, A. R. Standaert, A. H. Geeraerd, I. Cools, J. F. Van Impe and J. Debevere, J. Appl. Microbiol., 2005, 99, 1503–1515 CrossRef CAS PubMed.
- N.-C. Yip, F. J. Rawson, C. W. Tsang and P. M. Mendes, Biosens. Bioelectron., 2014, 57, 303–309 CrossRef CAS PubMed.
- S. M. Z. Hossain and J. D. Brennan, Anal. Chem., 2011, 83, 8772–8778 CrossRef CAS PubMed.
- C. Preininger and O. S. Wolfbeis, Biosens. Bioelectron., 1996, 11, 981–990 CrossRef CAS.
- X. Wang, M. Liu, X. Wang, Z. Wu, L. Yang, S. Xia, L. Chen and J. Zhao, Biosens. Bioelectron., 2013, 41, 557–562 CrossRef CAS PubMed.
- J. Li, Y. Yu, J. Qian, Y. Wang, J. Zhang and J. Zhi, Analyst, 2014, 139, 2806–2812 RSC.
- D. J. B. Dalzell, S. Alte, E. Aspichueta, A. de la Sota, J. Etxebarria, M. Gutierrez, C. C. Hoffmann, D. Sales, U. Obst and N. Christofi, Chemosphere, 2002, 47, 535–545 CrossRef CAS.
- G. Rosen, A. Osorio-Robayo, I. Rivera-Duarte and D. Lapota, Arch. Environ. Contam. Toxicol., 2008, 54, 606–611 CrossRef CAS PubMed.
- X. Lv, J. Liu, Y. L. Liu, Y. Zhao, M. L. Chen, P. Wang and W. Guo, Sens. Actuators, B, 2011, 158, 405–410 CrossRef CAS PubMed.
- World Health Organization, Guidelines for drinking-water quality, Geneva, 4th edn, 2011 Search PubMed.
- L. Zhao, S. Zhong, K. Fang, Z. Qian and J. Chen, J. Hazard. Mater., 2012, 239, 206–212 CrossRef PubMed.
- H. Xu, L. Zeng, S. Xing, Y. Xian and G. Shi, Electroanalysis, 2008, 20, 2655–2662 CrossRef CAS PubMed.
- P. Rattanarat, W. Dungchai, D. Cate, J. Volckens, O. Chailapakul and C. S. Henry, Anal. Chem., 2014, 86, 3555–3562 CrossRef CAS PubMed.
- Z. S. Qian, X. Y. Shan, L. J. Chai, J. R. Chen and H. Peng, Biosens. Bioelectron., 2015, 68, 225–231 CrossRef CAS PubMed.
- S. Cerminati, F. C. Soncini and S. K. Checa, Chem. Commun., 2015, 51, 5917–5920 RSC.
- J. Philp, C. French, S. Wiles, J. Bell, A. Whiteley and M. Bailey, Emerging Organic Pollutants in Waste Waters and Sludge, 2004, vol 1, 5, pp. 165–225 Search PubMed.
- C. Apartin and A. Ronco, Environ. Toxicol., 2001, 16, 117–120 CrossRef CAS PubMed.
|
This journal is © The Royal Society of Chemistry 2015 |
Click here to see how this site uses Cookies. View our privacy policy here.