DOI:
10.1039/C5RA07396K
(Paper)
RSC Adv., 2015,
5, 58609-58615
A new chiral electrochemical sensor for the enantioselective recognition of naproxen enantiomers using L-cysteine self-assembled over gold nanoparticles on a gold electrode
Received
23rd April 2015
, Accepted 16th June 2015
First published on 17th June 2015
Abstract
A chiral electrochemical sensor for analyzing the enantiomeric composition of chiral compounds is reported. The method is based on the difference between the interactions of naproxen (Nap) enantiomers with a chiral modified electrode surface. A chiral surface was synthesized on a gold electrode modified with gold nanoparticles and L-cysteine self-assembled monolayers (SAMs). Scanning electron microscopy (SEM), cyclic voltammetry (CV) and electrochemical impedance spectroscopy (EIS) were used to study the enantioselective interaction between the chiral surface and the Nap enantiomers. The results showed that the modified electrode is more stereoselective for S-Nap than for R-Nap. An examination of the characteristics of the chiral interface, including the response time, the effect of enantiomer concentration, and the stability, was performed. Then, the chiral selective interface was used to determine the Nap enantiomers within mixtures by measuring the change in the peak current with different mixture compositions. The results suggested that the proposed chiral biosensor with high sensitivity and selectivity can be used for the recognition of Nap enantiomers.
Introduction
Recognition of chiral drugs is an important and attractive subject in the field of life science since individual isomers of a drug often differ in their bioactivities and pharmacological activities and undergo different pharmacokinetic and metabolic fates in biological systems.1,2 The enantiomers of a chiral molecule may exhibit different biological activities. One of the enantiomers may be effective, while the other may be ineffective or even cause serious side-effects.3 Chemical analysis of chiral compounds, due to the different physiological and therapeutic properties of their enantiomers, is a concern of science and technology researchers.4,5
Naproxen (Nap), i.e., 2-(6-methoxy-naphthyl)propanoic acid, is a member of the 2-arylpropionic acid (profen) family of non-steroidal anti-inflammatory drugs (NSAIDs). NSAIDs including Nap are commonly employed to reduce ongoing inflammation, pain and fever, since they are able to block6 the cyclo-oxygenase (Cox) enzymes (Cox-1 and Cox-2), that both produce prostaglandins; a class of compounds that has several important functions, such as the promotion of inflammation, pain and fever.7 Nap is extensively used in the treatment of many diseases like rheumatoid arthritis, degenerative joint disease, ankylosing spondylitis, acute gout and primary dismenorrea.8 The pharmaceutical activity of S-naproxen (S-Nap) is 28 times stronger than that of R-naproxen (R-Nap), while R-Nap gives rise to some undesirable side-effects.9 Thence, the chiral analysis of naproxen enantiomers in the presence of each other is important.
Various methods have been used for chiral analysis, including spectrofluorometry10 and resonance Rayleigh scattering (RRS)11 after solid phase extraction, high performance liquid chromatography (HPLC),12–14 gas chromatography,15 capillary electrophoresis (CE),16 chiral ligand exchange chromatography (CLEC),17 fluorescence detection,18,19 mass spectrometry,20 NMR spectroscopy,21 near infrared spectroscopy,22 room temperature phosphorescence,23 molecularly imprinted polymer chiral stationary phases24 and electrochemical methods.25
Recently, electrochemical techniques were used for the chiral analysis of different enantiomers. Electrochemical methods have a lot of advantages such as low cost, high sensitivity and simplicity. Several electrochemical methods have been reported for the analysis of enantiomer compositions. Huang et al. fabricated a chiral sensor with a nanostructured composite for the enantioselective recognition of lysine enantiomers.26 Chen et al. reported a gold electrode modified with Cu(II) and L-cysteine for the chiral recognition of tryptophan enantiomers.27 Nie et al. constructed a chiral electrochemical sensor via a cysteic acid modified glassy carbon electrode to discriminate tyrosine enantiomers.28 Guo et al. designed a chiral sensor for the enantioselective recognition of Nap enantiomers using BSA biofunctionalized reduced graphene oxide nanosheets.29 Chen et al. fabricated a chiral electrochemical sensor through chemically linking L-methotrexate onto a gold electrode surface for the enantioselective recognition of penicillamine enantiomers.30 Zhang et al. constructed a chiral electrochemical sensor by adsorbing human serum albumin onto a methylene blue–multi-wall carbon nanotube nanohybrid modified glassy carbon electrode for the discrimination of tryptophan enantiomers.31
The present sensors with high sensitivity, simplicity, low cost, low-power requirements, and high compatibility have been a preferable approach for the chiral recognition of Nap enantiomers.
One of the principal goals of chiral recognition is to construct an effectual chiral selective system, which should have recognition sites for certain chiral enantiomers.
In this work, a chiral surface was synthesized from a gold electrode modified with cysteine self-assembled monolayers (SAMs) over gold nanoparticles. The self-assembly procedure is a modification method for the surface of electrodes, which have been used for the improvement of sensors and biosensors, because it leads to the spontaneous chemisorption of the cysteine thiol groups onto the gold surface.
Gold nanoparticles have potential applications in the construction of electrochemical sensors and biosensors32 because of their small dimensional size, good stability, biocompatibility, good conductivity and excellent catalytic activity.33 Furthermore, gold nanoparticle modifications can greatly increase the immobilized amount of S-functionalized compounds and enhance the Au–S bond and stability of the SAMs.34 Also, electrochemical deposition of gold nanoparticles (AuNPs) is a fast and convenient method of preparation.35,36
The gold nanoparticle synthesis was performed using an electrodeposition method at a constant potential, then L-cysteine was self-assembled onto the modified electrode. Cysteine, a small thiol-containing amino acid, contains carboxyl, amino and thiol functionalities37 with a chiral centre. Since the interaction between the two enantiomers of Nap and the chiral interface is different, the Nap enantiomers can be recognized. Scanning electron microscopy (SEM), cyclic voltammetry (CV) and electrochemical impedance spectroscopy (EIS) were used to analyze the characteristics of the interface. The results showed that the modified electrode could be used to discriminate Nap enantiomers, and that the chiral surface has a good response towards the quantitative analysis of enantiomer compositions in pharmaceutical samples. Also, the modified electrode can be used to determine the concentration ratio of Nap enantiomers in racemic mixtures.
Experimental
Reagents and materials
L-Cysteine (L-Cys), R- and S-Nap, L- and D-tryptophan (Trp), L- and D-alalanine (Ala), L- and D-histidine (His), L- and D-proline (Pro), KAu(CN)4, K3[Fe(CN)6], K4[Fe(CN)6] and other chemicals were supplied by Aldrich Chem. Co. (Milwaukee, WI, USA). Phosphate buffer was prepared using KH2PO4 and K2HPO4 which contained 0.1 M KCl (PBS, pH = 6). All other reagents were commercially available and of analytical grade. Double distilled water was used throughout the experiments.
Apparatus
Cyclic voltammetry (CV) and electrochemical impedance spectroscopy (EIS) measurements were performed with a potentiostat/galvanostat (Autolab PGSTAT302 N) and they were controlled by a computer using Nova version 1.7 software. A conventional three electrode system was employed with bare or modified gold electrodes (Au, Φ = 4 mm) as the working electrodes, a platinum wire auxiliary electrode as the counter electrode and an Ag/AgCl electrode as the reference electrode. Cyclic voltammetry and electrochemical impedance spectroscopy were measured in 2.5 mM [Fe(CN)6]4−/3− solution. A Mira FEG-SEM instrument by TESCAN was used to obtain the scanning electron microscopy (SEM) images of the different surfaces. All measurements were performed at room temperature.
Preparation of the working electrode
The gold electrode was polished with 1.0, 0.3 and 0.05 μM alumina slurries, and sonicated in double distilled water, ethanol, and double distilled water each for 5 min. Then the electrode was transferred to the electrochemical cell for cleaning by several potential cycles between −0.25 and +1.6 V vs. Ag/AgCl at 1 V s−1 in 0.5 M H2SO4 for 10 cycles and then 10 cycles in fresh 0.5 M H2SO4 at 0.1 V s−1 with the same potential range, then it was subsequently rinsed with double distilled water. At last, the pre-treated electrode was immersed in a solution containing 6 mmol L−1 KAu(CN)4 and 0.1 mol L−1 KNO3. The potential applied between the working electrode and Ag/AgCl electrode as the reference electrode was held constant at −400 mV (bulk electrolysis, BE) for 400 s.26 This electrode is denoted as Au/AuNPs.
A self-assembled monolayer of cysteine was formed on a bare Au electrode and the gold nanoparticle modified electrode (Au/AuNPs) by soaking the electrodes in 5 mmol L−1 L-cysteine/0.1 mol L−1 PBS/pH 2.58 for 15 min at room temperature.23 These electrodes are denoted as Au–Cys and Au/AuNPs–Cys, respectively. The modified electrodes were washed with doubly distilled water to remove the physically adsorbed species and dried carefully without touching the surface. Scheme 1 shows the stepwise preparation of the modified electrodes.
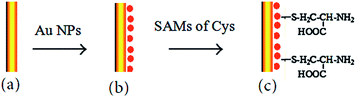 |
| Scheme 1 Schematic model of the chiral surface fabrication process (a) bare Au, (b) Au/AuNPs, (c) Au/AuNPs–Cys. | |
Results and discussion
SEM measurements
Scanning electron microscopy (SEM) was used to investigate the surface morphology characteristics of the modified electrodes. Fig. 1 displays the SEM images of the stepwise preparation of the modified electrodes. The SEM images of Au/AuNPs and Au/AuNPs–Cys electrodes are shown in Fig. 1A and B, respectively. As seen in Fig. 1A, Au nanoparticles are randomly formed electrochemically and increase the surface area. The shape and size of the nanoparticles are not homogenous. The diameters of the synthesized nanoparticles were around 18–51 nm. Also, in Fig. 1B cysteine molecules are assembled on the electrode surface.
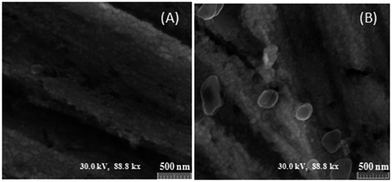 |
| Fig. 1 SEM images of the modified electrodes: (A) Au/AuNPs; (B) Au/AuNPs–Cys. | |
These results confirm the existence of AuNPs on the modified electrode surface and also the self-assembly of cysteine with the sulfide groups.
Electrochemical characterization of the modified electrodes
The cyclic voltammetry of ferricyanide is a valuable and convenient tool for monitoring the barrier of the modified electrode. It was used to study the interface properties of the modified electrodes. The cyclic voltammograms (CVs) of the different modified electrodes in 2.5 mM [Fe(CN)6]4−/3− solution at a scan rate of 100 mV s−1 are shown in Fig. 2A. As can be seen, the reversible one-electron redox behavior of ferricyanide ions was observed on the bare gold electrode at the scan rate of 100 mV s−1. After deposition of the Au nanoparticles on the surface of the gold electrode (Au/AuNPs electrode) the peak current increased. The increase in peak current is due to the increase in the electrode surface area following electrodeposition of the AuNPs. After self-assembly of the monolayer of cysteine on the gold nanoparticle modified electrode (curve c) the redox peak current decreased a little, while ΔEp increased (ΔEp = 110 mV). This indicates that the self-assembled cysteine monolayer partly blocked the electron-transfer from [Fe(CN)6]4−/3− at the gold electrode surface so the redox current decreased and the oxidation and reduction potentials increased.
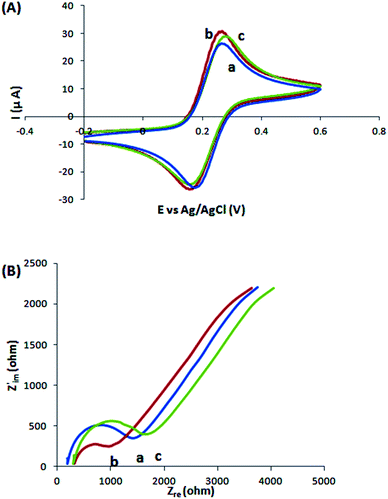 |
| Fig. 2 (A) CVs of the: (a) Au, (b) Au/AuNPs, (c) Au/AuNPs–Cys electrodes. Scan potential: −0.20 to 0.6 V vs. Ag/AgCl. Scan rate: 100 mV s−1. (B) EIS of the different electrodes, in 0.1 M KCl containing 2.5 mM [Fe(CN)6]4−/3− solution at +0.25 V from 0.1 Hz to 10.0 kHz. | |
The results of the EIS for the bare Au electrode, Au/AuNPs electrode and Au/AuNPs–Cys electrode in the presence of 2.5 mM [Fe(CN)6]4−/3− in 0.1 M KCl are shown in Fig. 2B. It can be seen that the bare Au electrode displays a semicircular part in the high frequency region. The charge transfer resistance (Rct) was estimated to be 930 Ω. After being modified with Au nanoparticles, the semicircle diameter from EIS decreased and Rct decreased to 566 Ω, indicating that the Au nanoparticles were deposited on the Au electrode surface. After self-assembly of the monolayer of cysteine, Rct was found to be 1131 Ω.
The CVs of the Au/AuNPs–Cys electrode in [Fe (CN)6]4−/3− solution at different scan rates were studied. The peak potential and current depended on the scan rate. A linear relationship between the oxidation peak current (Ip) and the square root of the scan rate (ν1/2) in the range 60–400 mV s−1 confirms a diffusion controlled electrochemical process.
Furthermore, the stability of the modified electrodes was studied. After 50 cycles of CV measurements in [Fe(CN)6]4−/3− at 100 mV s−1, the relative standard deviation (RSD) of the peak current was 0.65% (n = 5), indicating the good stability of this interface.
Also the reversibility of the sensor was investigated. After immersion of the chiral sensor in a 100 mg L−1 R- or S-Nap solution for 20 min and then immersion in [Fe(CN)6]4−/3− solution, the peak current reached 99.2% of the blank peak current value (before immersion in the enantiomer solution) after 5 cycles of CV measurements, and the peak current reached 99.7% of the blank value after 10 cycles of CV. Thus the results indicated a good reversibility.
Enantioselective recognition of Nap enantiomers
The CV response. Under the optimum experimental conditions, the enantioselective recognition of the Nap enantiomers was carried out by the fabricated chiral biosensor. Fig. 3 shows the cyclic voltammograms for the Au/AuNPs–Cys modified electrode in a solution of 2.5 mM [Fe(CN)6]4−/3− at a scan rate of 100 mV s−1 before (curve a) and after immersion in 100 mg L−1 R-Nap (curve b) or S-Nap (curve c) solutions for 20 min. As shown in Fig. 3, the redox peak current decreased and a larger decrease was obtained for curve c than curve b (the current changed from 29.6 μA to 27.7 μA and 19.3 μA for R-Nap and S-Nap, respectively) which indicates the presence of an interaction between L-Cys and R- or S-Nap, but the interaction of R-Nap was weaker than that for S-Nap. This is because a higher amount of R-Nap than S-Nap became adsorbed onto the surface of the chiral electrode, making a larger blocking layer for the electron transfer at the surface of the electrode. The change in current response for S-Nap, ΔIS (ΔIS = I − IS, where I and IS are the peak current before and after submerging the electrode in S-Nap solution, respectively), was found to be 10.3 μA, while the change in current response for the R-Nap solution, ΔIR (ΔIR = I − IR, where IR denotes the peak current after submerging the electrode in R-Nap solution) was found to be 1.9 μA. The difference between the current response changes for R-Nap (ΔIR) and S-Nap (ΔIS), ΔIRS (ΔIRS = ΔIR − ΔIS), was calculated as 8.4 μA. Such a large difference makes the developed chiral biosensor effective in the enantioselective recognition of Nap enantiomers. The results indicated that S-Nap binds more strongly than R-Nap.
 |
| Fig. 3 CVs for the modified electrode in 0.1 M KCl containing 2.5 mM [Fe(CN)6]4−/3− solution (a) before and (b) after incubation with 100 mg L−1 R-Nap or (c) S-Nap. Scan potential: −0.20 to 0.6 V vs. Ag/AgCl. Scan rate: 100 mV s−1, pH = 6, time of incubation = 20 min. | |
EIS measurements. EIS is usually used to probe the interfacial properties of modified electrodes.38 In EIS, the total impedance is determined by several parameters: (1) electrolyte resistance, Rs; (2) the lipid bilayer capacitance, Cdl; (3) charge transfer resistance, Rct, and (4) the Warburg element, Zw. A modified Randles equivalent circuit was used to fit the measured results (inset of Fig. 4). Rs and Zw represent the bulk properties of the electrolyte solution and diffusion of the applied redox probe, respectively, which are not affected by chemical transformations occurring at the electrode interface. Cdl depends on the dielectric permittivity introduced into the doubly-charged layer molecules, and for less polar molecules the capacitance should be smaller. Rct depends on the insulating features at the electrode/electrolyte interface. The semicircle diameter in the impedance spectrum equals the interfacial electron transfer resistance (Rct).27 Fig. 4 illustrates the electron-transfer resistance of the modified electrodes before and after immersion in 100 mg L−1 R- or S-Nap solution for 20 min. After interaction of the modified electrode (Rct = 1.13 kΩ, curve a) with R-Nap or S-Nap, the Rct increased. Interaction between the electrode and the enantiomers of Nap, results in diminishing electron transfer from the ferrocyanide/ferricyanide. Also, because of the stronger interaction of S-Nap (Rct = 1.971 kΩ, curve c) compared to R-Nap (Rct = 1.481 kΩ, curve b) the electron-transfer resistance is larger. These results are consistent with those obtained from CV.
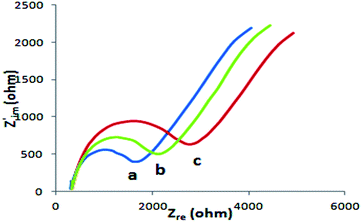 |
| Fig. 4 The EIS results for the modified electrode in 2.5 mM [Fe(CN)6]4−/3− solutions containing 0.1 M KCl (a) before and (b) after incubation in 100 mg L−1 R-Nap or (c) S-Nap. Scan potential: −0.20 to 0.6 V vs. Ag/AgCl. Scan rate: 100 mV s−1, pH = 6, time of incubation = 20 min. | |
Optimization of the experimental conditions
Influence of the pH. The pH of the solution plays an important role in the enantiospecificity of the Nap enantiomers. The effect of pH on the electrochemical response of the chiral biosensor was studied over the pH range 3 to 9 (Fig. 5A). It can be seen that the maximum difference in peak current between the two enantiomers appeared at pH 6.0. Therefore, all experiments were performed at pH 6.0.
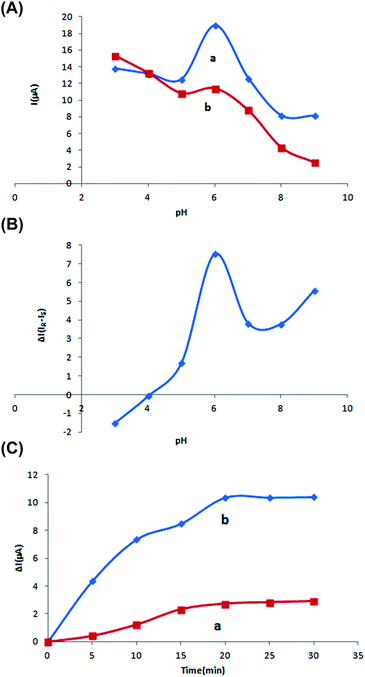 |
| Fig. 5 (A) The effect of pH on the peak current change of the chiral biosensor at pH: 3.0, 4.0, 5.0, 6.0, 7.0, 8.0, 9.0; (B) the dependence of ΔIp on pH and (C) the dependence of the peak current change on time incubated for (a) R-Nap and (b) S-Nap. Scan potential: −0.20 to 0.6 V vs. Ag/AgCl. Scan rate: 100 mV s−1. | |
Influence of the interaction time. Furthermore, the influence of the interaction time was studied from 5 to 30 min. As Fig. 5C shows, by increasing the interaction time of the S-Nap or R-Nap enantiomers the peak current change increases for both the enantiomers, and then tends to level off with a further increase in reaction time. So, an interaction time of 20 min was selected as the optimum time.
Enantioselectivity coefficient of the chiral biosensor
The selectivity of the chiral biosensor was quantitatively evaluated using the enantioselectivity coefficient (α), which is defined using the following equation:12
where α is the enantioselectivity coefficient, a quantitative measure of a sensor’s ability to discriminate the S-isomer from the interfering R-isomer, and ΔIS and ΔIR are the differences in the anodic peak current after immersion of the modified electrodes, corresponding to S- and R-naproxen, respectively.
The enantioselectivity coefficient for the chiral sensor was calculated as 5.4.
Four different chiral amino acids (tryptophan, alanine, histidine and proline) were also examined under the same conditions to investigate the selectivity of the chiral sensor. Table 1 shows the average differences in the anodic peak current and the enantioselectivity coefficient for the chiral sensor corresponding to the different amino acid enantiomers. The results show that the sensor has good selectivity and specificity for Nap enantiomers.
Table 1 Enantioselectivity coefficient (α) of the chiral sensor corresponding to the amino acids enantiomers
Chiral analyte |
ΔIS (mA) |
ΔIR (mA) |
α |
Nap |
10.3 |
1.9 |
5.4 |
Trp |
9.2 |
3.4 |
2.7 |
Pro |
10.5 |
7.8 |
1.3 |
His |
6.6 |
5.6 |
1.2 |
Ala |
6.4 |
6.1 |
— |
To the best of our knowledge, only one report has been published on the introduction of an enantioselective electrochemical sensor for Nap.29 The proposed sensor was compared with the reported one. As Table 2 shows, the obtained enantioselectivity coefficient for the proposed sensor is more than 2.5 times greater than that for the reported one.
Table 2 Comparison of the enantioselective electrochemical sensors for naproxen enantiomers
Type of electrode |
Detection method |
Linear range (μmol L−1) |
LOD (μmol L−1) |
α |
Ref. |
TBO@rGO/GCE |
CV |
500 to 5000 |
0.33 |
2.29 |
29 |
Au/AuNPs–L-Cys E |
CV |
2 to 20 |
0.67 |
5.4 |
This work |
Influence of naproxen concentration. Cyclic voltammetry measurements were performed using different concentrations of S-Nap and R-Nap. As shown in Fig. 6, the relationship between the anodic peak current change and the concentration of the Nap enantiomers was linear. By increasing the concentration of S-Nap in the range 2.0 × 10−6 mol L−1 to 2.0 × 10−5 mol L−1, the peak current increased according to the linear equation, Ip (ΔIS) (μA) = 0.5627C (μM) + 0.5463 (R = 0.9701). The [Fe(CN)6]4−/3− solution peak current changed after immersion of the electrode in R-Nap solution for 20 min. Also, the peak current change increased linearly by increasing the S-Nap concentration and the linear equation was Ip (ΔIR) (μA) = 0.0702C (μM) + 0.0496 (R = 0.9836). The obtained detection limit was 6.7 × 10−7 mol L−1 (S/N = 3). As seen, an increase in the concentration of both enantiomers caused an increase in the current change at the modified electrode, but S-Nap caused a larger current change than R-Nap. Therefore, the chiral biosensor could enantioselectively recognize the Nap enantiomers.
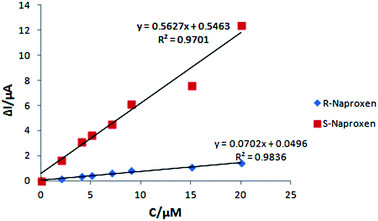 |
| Fig. 6 The relative change of oxidation current peak for different concentrations of naproxen enantiomers adsorbed on modified electrodes: (a) S-naproxen and (b) R-naproxen. | |
Application of the enantioselective electrode
Herein, the application of a chiral biosensor was studied. Different volume ratios of S- and R-Nap mixed solutions were prepared. The peak current change gradually increased by increasing the ratio of S-Nap enantiomer to the R-Nap enantiomer for both high and low concentrations (Fig. 7). As seen, the modified electrode surface displayed higher chiral sensitivity towards S-Nap rather than its counter isomer in the mixed solutions. Also, the calibration curves showed good linearity, the chiral biosensor can be used to detect one enantiomer of naproxen in the presence of the other isomer.
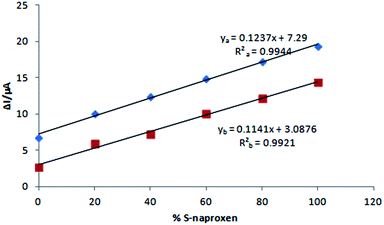 |
| Fig. 7 The relative change of the modified electrode oxidation current peak with an enantiomeric composition of R- and S-naproxen at concentrations (a) 100 mg L−1 and (b) 50 mg L−1. | |
Conclusion
The present study demonstrates an excellent sensor based on L-Cys-SAMs desposited on an AuNPs/Au electrode for recognizing one of the enantiomers of Nap in the presence of the other optical isomer. The modified electrode could be used successfully for the enantioselective sensing of S-Nap in the presence of R-Nap. The results showed that a larger decrease in the electrochemical signal was obtained from S-Nap than from R-Nap. The chiral recognition was based on the different interactions of the Nap enantiomers with the chiral modified electrode surface. The electrochemical response of a series of different concentrations of R- and S-Nap was measured, and a linear relationship was observed between the peak current and the concentration of Nap enantiomers. Of course, the slope of the calibration curve for S-Nap was larger than that for R-Nap. Furthermore, the signals for Nap mixtures with different enantiomeric compositions were investigated.
Acknowledgements
The authors acknowledge the Bu-Ali Sina University Research Council and the Centre of Excellence in Development of Environmentally Friendly Methods for Chemical Synthesis (CEDEFMCS) for providing support to this work.
References
- M. Zhang and B. C. Ye, Anal. Chem., 2011, 83, 1504–1509 CrossRef CAS PubMed.
- M. Trojanowicz and M. Kaniewskaa, Electroanalysis, 2009, 21, 229–238 CrossRef CAS PubMed.
- T. Q. Yan and C. Orihuela, J. Chromatogr. A, 2007, 1156, 220–227 CrossRef CAS PubMed.
- G. Absalan and Y. Alipour, J. Pharm. Biomed. Anal., 2013, 83, 96–100 CrossRef CAS PubMed.
- A. Afkhami, M. Abbasi-Tarighat and M. Bahram, Talanta, 2008, 75, 91–98 CrossRef CAS PubMed.
- Y. Sun, Z. Zhang, Z. Xi and Z. Shi, Talanta, 2009, 79, 676–680 CrossRef CAS PubMed.
- T. Madrakian, M. Ahmadi, A. Afkhami and M. Soleimani, J. Anal., 2013, 138, 4542–4549 RSC.
- P. A. Todd and S. P. Clissold, Drugs, 1990, 40, 91–137 CrossRef CAS PubMed.
- F. Dimiza, A. N. Papadopoulos, V. Tangoulis, V. Psycharis, C. P. Raptopoulou, D. P. Kessissoglou and G. Psomas, J. Inorg. Biochem., 2012, 107, 54–64 CrossRef CAS PubMed.
- M. Ahmadi, T. Madrakian and A. Afkhami, RSC Adv., 2015, 5, 5450–5457 RSC.
- M. Ahmadi, T. Madrakian and A. Afkhami, Sens. Actuators, B, 2015, 210, 439–445 CrossRef CAS PubMed.
- L. J. Zhang, M. F. Song, Q. Tian and S. G. Min, Sep. Purif. Technol., 2007, 55, 388–391 CrossRef CAS PubMed.
- Z. X. Zheng, J. M. Lin and F. Qu, J. Chromatogr. A, 2003, 1007, 189–196 CrossRef CAS.
- X. N. Lu, Y. Chen, L. Guo and Y. F. Yang, J. Chromatogr. A, 2002, 945, 249–255 CrossRef CAS.
- W. Liu and J. J. Gan, J. Agric. Food Chem., 2004, 52, 755–761 CrossRef CAS PubMed.
- J. Elek, D. Mangelings, T. Ivanyi, I. Lazar and Y. V. Heyden, J. Pharm. Biomed. Anal., 2005, 38, 601–608 CrossRef CAS PubMed.
- L. Qi, G. I. Yang, H. Z. Zhang and J. Qiao, Talanta, 2010, 81, 1554–1559 CrossRef CAS PubMed.
- L. Chi, J. Z. Zhao and T. D. James, J. Org. Chem., 2008, 73, 4684–4687 CrossRef CAS PubMed.
- Y. L. Wei, S. F. Wang, S. M. Shuang and C. Dong, Talanta, 2010, 81, 1800–1810 CrossRef CAS PubMed.
- W. A. Tao, F. C. Gozzo and R. G. Cooks, Anal. Chem., 2001, 73, 1692–1698 CrossRef CAS.
- C. Roussel, M. Roman, F. Andreoli, A. Del Rio, R. Faure and N. Vanthuyne, Chirality, 2006, 18, 762–771 CrossRef CAS PubMed.
- C. D. Tran, D. Oliveira and S. Yu, Anal. Chem., 2006, 78, 1349–1356 CrossRef CAS PubMed.
- Y. Wei, Y. Ren, J. Li, S. Shuang and C. Dong, Analyst, 2011, 136, 299–303 RSC.
- J.-D. Lei and T.-W. Tan, Biochem. Eng. J., 2002, 11, 175–179 CrossRef CAS.
- H. S. Guo, J. M. Kim, S. M. Chang and W. S. Kim, Biosens. Bioelectron., 2009, 24, 2931–2934 CrossRef CAS PubMed.
- Y. Huang, D. Guo, Q. Zhang, L. Guo, Y. Chen and Y. Fu, RSC Adv., 2014, 4, 33457–33461 RSC.
- Q. Chen, J. Zhou, Q. Han, Y. Wang and Y. Fu, Colloids Surf., B, 2012, 92, 130–135 CrossRef CAS PubMed.
- R. Nie, X. Bo, H. Wang, L. Zeng and L. Guo, Electrochem. Commun., 2013, 27, 112–115 CrossRef CAS PubMed.
- L. Guo, Y. Huang, Q. Zhang, C. Chen, D. Guo, Y. Chen and Y. Fu, J. Electrochem. Soc., 2014, 161, B70–B74 CrossRef CAS PubMed.
- Q. Chen, J. Zhou, Q. Han, Y. Wang and Y. Fu, J. Solid State Electrochem., 2012, 16, 2481–2485 CrossRef CAS PubMed.
- Q. Zhang, L. Guo, Y. Huang, Y. Wang, Q. Han and Y. Fu, Anal. Methods, 2013, 5, 4397–4401 RSC.
- N. F. Atta, A. Galal, F. M. Abu-Attia and S. M. Azab, J. Electrochem. Soc., 2010, 157, F116–F123 CrossRef CAS PubMed.
- R. N. Goyal, V. K. Gupta, M. Oyama and N. Bachheti, Talanta, 2007, 72, 976–983 CrossRef CAS PubMed.
- T. Quczak, Electrochim. Acta, 2009, 54, 5863–5870 CrossRef PubMed.
- Y. Zhang, S. Asahina, S. Yoshihara and T. Shirakashi, Electrochim. Acta, 2003, 48, 741–747 CrossRef CAS.
- M. S. El-Deab, T. Sotomura and T. Ohsaka, J. Electrochem. Soc., 2005, 152, C730–C737 CrossRef PubMed.
- G. Hager and A. G. Brolo, J. Electroanal. Chem., 2003, 550, 291–301 CrossRef.
- N. F. Atta, A. Galal and E. H. El-Ads, Analyst, 2012, 137, 2658–2668 RSC.
|
This journal is © The Royal Society of Chemistry 2015 |
Click here to see how this site uses Cookies. View our privacy policy here.