DOI:
10.1039/C5RA07606D
(Paper)
RSC Adv., 2015,
5, 58821-58831
The effect of bis allyl benzoxazine on the thermal, mechanical and dielectric properties of bismaleimide–cyanate blend polymers
Received
26th April 2015
, Accepted 25th June 2015
First published on 26th June 2015
Abstract
Thermosetting terpolymers composed of bis allyl benzoxazine (Bz-allyl), cyanate ester (BADCy) and 4,4′-bismaleimidodiphenyl methane (BMI) were prepared via co-curing reactions. The curing kinetics of Bz-allyl/BMI/BADCy were investigated via non-isothermal DSC at different heating rates using the Flynn–Wall–Ozawa method. The dielectric, thermal and mechanical properties of Bz-allyl/BMI/BADCy terpolymers were systemically investigated in detail through mechanical measurement, scanning electron microscopy (SEM), dynamic mechanical analysis (DMA) and thermo-gravimetric analysis (TGA). The results show that a suitable addition of Bz-allyl can enhance the impact strength and flexural strength as well as reduce the dielectric constant and the dielectric loss of BMI/BADCy. The dynamic mechanical analysis reveals that the cross-link density of the blend is higher than BMI/BADCy. The higher crosslinking density of the terpolymer led to good thermal stability of the terpolymer. Scanning electron microscopy analysis shows the distinct characteristics of ductile fracture of the blends. All of these changes in properties are closely correlated to the copolymerization between Bz-allyl and BMI/BADCy, which could form an interpenetrating polymer network in the system.
Introduction
Polybenzoxazines have attracted significant interest as a class of high performance phenolic resin. Compared with traditional phenolic resin, polybenzoxazine possesses several superior properties,1,2 such as near-zero shrinkage during polymerization, high thermal stability, flame retardancy, good mechanical strength, low dielectric constant, can be cured without any added initiator or catalyst and good reactivity and compatibility with other polymers.3,4 In addition, with the molecular design flexibility, polybenzoxazine precursors can be prepared, which makes the tailoring of well-defined molecular structures for specific applications possible.5 The major advantages of the typical polybenzoxazines are associated with the existence of inter- and intramolecular hydrogen bonds in the network structure.6–8 Therefore, benzoxazine resin is widely used in the modification of thermosetting resin.
Polycyanurates derived from the thermal curing of cyanate ester possess high thermal properties, low dielectric constants and good mechanical properties after being fully cured to form a triazine network.9–13 However, the thermal characteristics are inferior to those of conventional bismaleimide systems. Blends of cyanate ester and bismaleimide have been realized to derive systems bearing the good physicochemical attributes of the two components, i.e. the heat resistance of bismaleimide and the mechanical properties of polycyanurates.14–18 Some blend systems were investigated by the addition polymerization of bisphenol A dicyanate (BADCy) and 4,4′-bismaleimido diphenylmethane,19 and also BADCy and 2,2′-bis[4-(4-maleimido phenoxy)propane].20,21 Commercial blend formulations of bismaleimide–cyanate known as B-T resins are available, which are extensively used as materials for engineering materials in aircrafts, reinforced plastics, and injection-molding powders, as well as materials in electric motor coil windings, etc.22 However, according to research on the bismaleimide–cyanate system,23,24 the copolymerization of bismaleimide with cyanate resin will result in an interpenetrating network (IPN) structure with high thermal and mechanical properties. Some novel allyl-functionalised aryl cyanate esters have been developed to be incorporated into cyanate ester/bismaleimide blends.25–29 The addition of a small amount of allyl-functionalised aryl cyanate esters may increase the Tg of the overall network by linking the polycyanurate network to the poly-bismaleimide network. So, bis allyl benzoxazine, which has allyl groups, is selected to modify bismaleimide–cyanate ester, and the composite is expected to possess better performance.
In this work, bis allyl benzoxazine (Bz-allyl) is utilised to modify BMI/BADCy resins, aiming to regulate their dielectric, heat resistance, and mechanical properties. The effects of Bz-allyl on the moisture resistance, mechanical, dielectric and thermal properties of Bz-allyl/BADCy/BMI composites were investigated to develop high performance materials.
Experimental
Materials
Bisphenol A cyanate ester (BADCy) resins were purchased from Shangyu Shengda Biochemical Co. Ltd (Shangyu, China). 4,4′-Bismaleimidodiphenyl methane (BMI) was received from Hubei Fengguang Chemicals, China. Bis allyl benzoxazine (Bz-allyl) was synthesized from diallyl bisphenol A, aniline and paraformaldehyde according to procedures previously described.5 The chemical structures of BADCy, BMI and Bz-allyl are shown in Fig. 1.
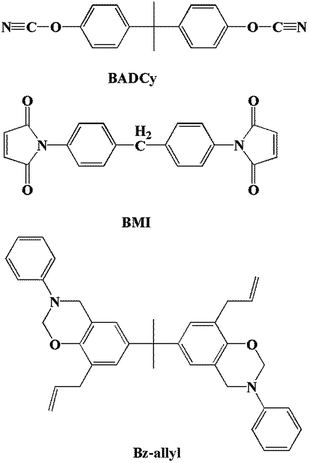 |
| Fig. 1 The chemical structures of BADCy, BMI and Bz-allyl. | |
Preparation of Bz-allyl/BMI blends
Appropriate quantities of BADCy and BMI were placed in a three-necked flask with a mechanical stirrer and a thermometer. The mass ratios of BADCy and BMI were 4
:
3. The mixture was heated to 160 °C and maintained at that temperature with stirring until a homogeneous liquid was obtained. The liquid was maintained at that temperature for an additional 0.5 h, which is the BMI/BADCy prepolymer. After that, the mixture was cooled to 140 °C, the Bz-allyl was added, and then the ternary mixture was maintained with stirring for 20 min and a brown-red transparent liquid was obtained. Bz-allyl was blended with BMI/BADCy in different mass ratios of 3%, 6%, 9% and 12%. The blends were denoted as BBz3, BBz6, BBz9 and BBz12, respectively.
Preparation of cured resins
Firstly, the blends were poured into the preheated mold with a silicon coating on the inner surface. Then, the blends were degassed in a vacuum oven (120 °C for 0.5 h under vacuum). Finally, the blends were cured following the procedures of 150 °C for 2 h + 180 °C for 3 h + 200 °C for 2 h, and post-cured at 240 °C for 2 h.
Characterization
The impact strength was measured according to GB/T2567-2008 using a testing machine (ZBC-50A Plastic Pendulum Impact Testing Machine, Shenzhen Sans Measurement Technology Co. Ltd, Shenzhen, China). The sample dimensions were (50 ± 0.02) × (7 ± 0.02) × (4 ± 0.02) mm3. Flexural strength and modulus were measured according to GB/T2567-2008 using a testing machine (CMT-6303 Electronic Tensile Testing Machine, Shenzhen Sans Measurement Technology Co. Ltd, Shenzhen, China). The sample dimensions were (80 ± 0.02) × (10 ± 0.02) × (4 ± 0.02) mm3. Five samples were tested for each composition, and the results are presented as an average for tested samples.
DSC measurements were performed with a Q1000DSC thermoanalyzer system (USA) ranging from room temperature to 380 °C under a N2 atmosphere. A dry nitrogen flow of 40 ml min−1 was used as the purge gas. Samples of about 10 mg were enclosed in aluminium DSC capsules. Dynamic mechanical analysis (DMA) was performed with a Switzerland Mettler-Toledo DMA with a sample size of 45 mm × 6 mm × 3 mm. DMA tests were carried out from 25 to 380 °C with a heating rate of 3 °C min−1 at 1 Hz. TGA tests were performed by using a Netzsch STA 449C thermogravimetric analyzer (Germany) at a heating rate of 10 °C min−1 under a N2 atmosphere from 20 to 800 °C.
The dielectric constant ε and loss factor tan
δ were measured using a high-frequency QBG-3 Gauger and a S914 dielectric loss test set (Shanghai AE Electron Equipment Co. Ltd, Shanghai, China) at a frequency between 10 and 60 MHz. The sample dimensions were (25 ± 0.02) × (25 ± 0.02) × (3 ± 0.02) mm3.
Scanning electron microscopy (SEM) was performed on a TESCAN VEGA3 LMH instrument. The SEM accelerating voltage was 20 kV. The water absorption of a sample was determined by swelling in distilled water for 20 h at 100 °C. The sample dimensions were (10 ± 0.02) × (10 ± 0.02) × (3 ± 0.02) mm3. FT-IR spectra were recorded on KBr pellets from 400 to 4000 cm−1 with a resolution of 4 cm−1 on a Nicolet IS10 IR spectrometer (USA).
Results and discussion
Curing behavior of BBz9 resins
To explain the high thermal properties of Bz-allyl/BADCy/BMI, it is necessary to study the curing behavior of BBz9 in order to establish the curing kinetic parameters and offer a better knowledge of the curing process and an improvement of the quality of high performance materials. The curing behaviors of BBz9 were studied using differential scanning calorimetry.
The non-isothermal DSC thermograms at the heating rates of 5 °C min−1, 10 °C min−1, 15 °C min−1, 20 °C min−1 and 25 °C min−1 are shown in Fig. 2. Obviously, the heating rate exerts a great influence on the curing process. With increasing the heating rate, the exothermic curing peaks were shifted to a higher temperature. Information about the nature of the curing reaction such as onset temperature (Ti), peak temperature (Tp), and endset temperature (Te) at different heating rates could be derived, which are listed in Table 1.
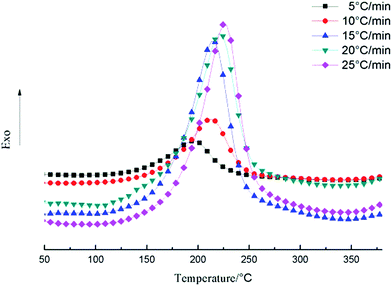 |
| Fig. 2 DSC curves of BBz9 at different heating rates. | |
Table 1 Exothermic peak temperatures of BBz9 at different heating rates
Heating rate (°C min−1) |
Onset temperature (Ti) (°C) |
Peak temperature (Tp) (°C) |
Endset temperature (Te) (°C) |
5 |
112 |
195 |
251 |
10 |
115 |
212 |
268 |
15 |
116 |
214 |
283 |
20 |
120 |
220 |
290 |
25 |
128 |
226 |
291 |
Various models had been proposed for analyzing the non-isothermal curing behavior of polymers.30–32 According to the relationship between the peak temperature and heating rate (β), the apparent activation energies (Ea) of the curing reaction of the terpolymer are 101.9 and 104.7 kJ mol−1 calculated using the Kissinger and Ozawa methods, respectively33,34 (Fig. 3). The Ea values from the Kissinger and Ozawa methods are quite close to each other and their differences may be caused by the different assumptions.
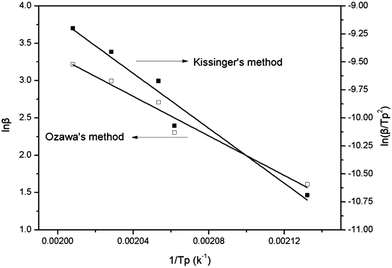 |
| Fig. 3 Representations of the Kissinger and Ozawa methods for calculating activation energy from non-isothermal data for the copolymer. | |
The reaction activation energy also could be calculated using the Flynn–Wall–Ozawa (FWO)35 (eqn (1)) multiheating rate method at different values of conversion.
|
log β = log[AEa/RG(α)] − 0.4567Ea/RT − 2.315
| (1) |
where
β is the rate of temperature increase,
α is the degree of curing reaction,
A is a constant,
Ea is the reaction activation energy,
R is the gas constant, and
G(
α) is the integral form.
For a constant conversion α, the plot of log
β versus 1/T obtained from DSC thermograms using various heating rates should render a straight line, where the slope allows the determination of the apparent activation energy. According to the Flynn–Wall–Ozawa method, linear relationships of log
β versus 1/T at various degrees of conversion are established in Fig. 4. Fig. 5 presents activation energy as a function of conversion. It can be seen that the activation energy values tended to increase with the degree of conversion.
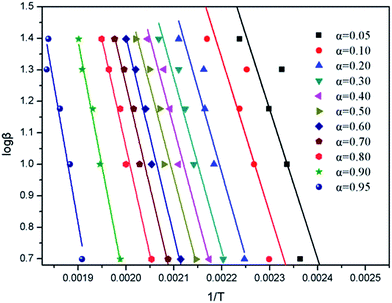 |
| Fig. 4 Flynn–Wall–Ozawa plots at various degrees of curing of BBz9. | |
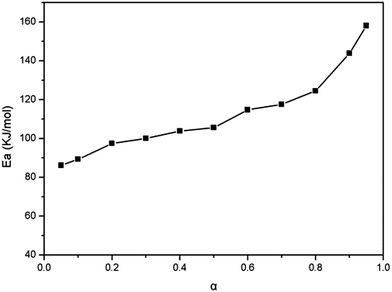 |
| Fig. 5 Variation of Ea versus conversion. | |
Mechanical properties of the Bz-allyl/BMI/BADCy resin system
The impact strengths of the BMI/BADCy resin and the blends with the different content of Bz-allyl are shown in Fig. 6. The three-point bend test was used to determine the flexural strength and modulus of the Bz-allyl/BMI/BADCy blends. The effects of composition on flexural strength and flexural modulus of the Bz-allyl/BMI/BADCy blends are depicted in Fig. 7. It is observed from Fig. 6 that the BBz9 system has the maximum impact strength (17.9 kJ m−2), which is increased by 50% compared with that of the BMI/BADCy resin. The BBz9 system has a maximum flexural strength (128 MPa) and a flexural modulus (4089 MPa), which are increased by 41% and 13%, respectively, compared with those of BMI/BADCy. Therefore, it can be concluded that the addition of Bz-allyl can efficiently improve the mechanical properties of the BMI/BADCy resin. The enhancement of impact and flexural strength may be attributed to the network structure of high crosslinking density and the flexible ether bonds in Bz-allyl/BMI/BADCy.
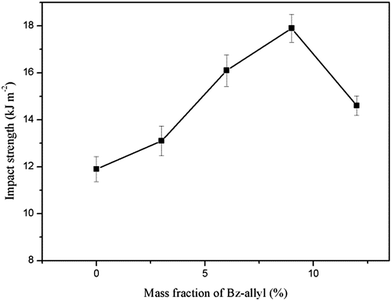 |
| Fig. 6 The impact strength of the Bz-allyl/BMI/BADCy systems. | |
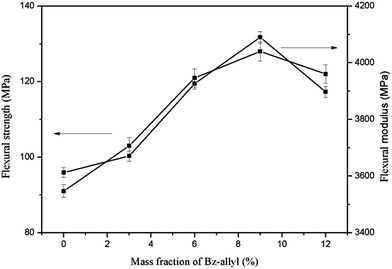 |
| Fig. 7 The flexural strength and flexural modulus of the Bz-allyl/BMI/BADCy systems. | |
To investigate the possible curing reactions of BBz9, FT-IR measurements were carried out. FT-IR spectra of BBz9 before and after curing are shown in Fig. 8. As a result, the absorption at 947 cm−1 assigned to the oxazine ring in the benzoxazine ring structure disappeared after curing. The absorptions at 2271 and 2236 cm−1 assigned to the cyanate ester group also disappeared. The new absorptions at 1564 and 1371 cm−1 assigned to the triazine group appeared. The appearance of an absorption band at 1213 cm−1 revealed the generation of ether bonds, which may be due to the reaction between BMI and PBz-allyl as shown in Scheme 2d.
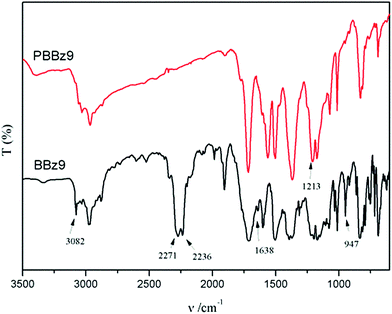 |
| Fig. 8 IR spectra of BBz9 before and after curing. | |
In the process of BMI and BADCy curing, the thermal reaction of BMI and BADCy can occur as shown in Scheme 1a and b, respectively. However, the curing mechanism of the Bz-allyl/BMI/BADCy blends is different from that of BMI/BADCy. Ene, Diels–Alder, homopolymerization, and alternating copolymerization reactions are involved in the modification of BMI/BADCy with Bz-allyl.36 The ene reaction occurs at a lower temperature compared with that of the other reactions as shown in Scheme 2c. The cure reactions of the blends of BADCy with BMI can occur as shown in Scheme 1.37 Additionally, parts of Bz-allyl undergo ring-opening polymerization to form polybenzoxazine and generate the phenolic hydroxyl group as shown in Scheme 2a, which can react with the BMI/BADCy as shown in Scheme 2d and e. With increasing temperature, Bz-allyl can react completely, but the allyl group seldom reacts (Scheme 2b) because of the stability of the radical due to resonance.38 On the one hand, the polymerization of the Bz-allyl/BMI/BADCy blends forms ether bonds, which results in good flexibility of the polymer. On the other hand, it may be attributed to the network structure of high crosslinking density formed in the Bz-allyl/BMI/BADCy blends. Moreover, the hydrogen bond is a physical interaction which will increase the impact strength and flexural strength of BMI/BADCy blends. Therefore, it can be concluded that the addition of Bz-allyl can efficiently improve the mechanical properties of BMI/BADCy resins.
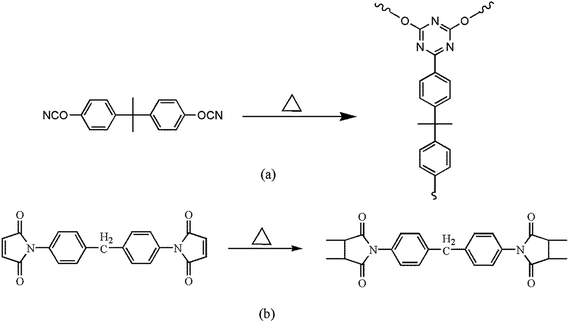 |
| Scheme 1 The curing procedure for BADCy and BMI (a) and (b), respectively. | |
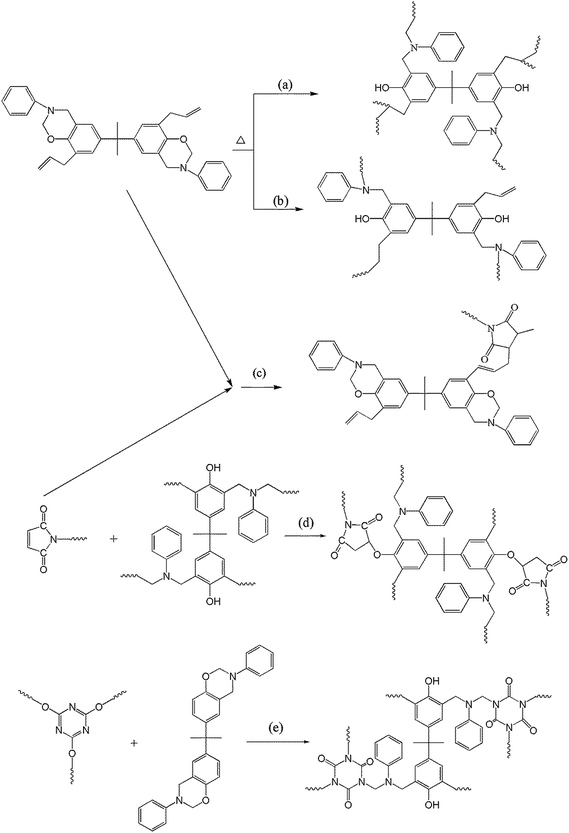 |
| Scheme 2 The curing procedure for Bz-allyl/BMI/BADCy. | |
Fracture surfaces of the Bz-allyl/BMI/BADCy resin system
To research the effect of Bz-allyl on the mechanical properties of BMI/BADCy resins, SEM images of the fracture surfaces of samples after impact tests are taken and shown in Fig. 9.
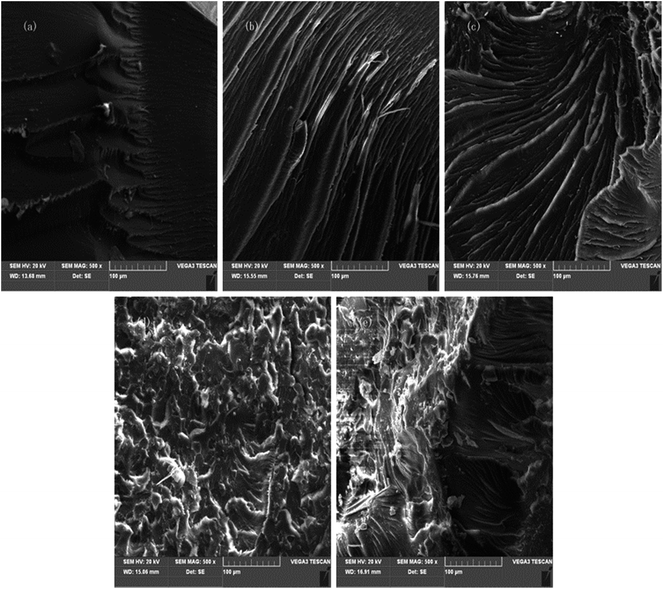 |
| Fig. 9 SEM images of fracture surfaces of Bz-allyl/BMI/BADCy systems: (a) BMI/BADCy, (b) BBz3, (c) BBz6, (d) BBz9 and (e) BBz12. | |
Fig. 9a–e presents SEM images of the fracture surfaces of BMI/BADCy, BBz3, BBz6, BBz9 and BBz12, respectively. It can be observed that the BMI/BADCy resin has a smooth and riverlike fracture surface (Fig. 9a), exhibiting a typical brittle feature. While with the addition of Bz-allyl into the BMI/BADCy resin, the fracture surface becomes rougher and is accompanied with more ductile sunken areas, which is consistent with the improved impact strength of the composites. For the BMI/BADCy resin, BBz3 and BBz6, as shown in Fig. 9a–c, with the content of the Bz-allyl increased, it can be observed that the riverlike fracture surfaces become more concentrated. The aspect ratios of cracks on the river region of the blend (Fig. 9c) are smaller than those of the BMI/BADCy resin (Fig. 9a), and there are more ductile sunken areas than for the BMI/BADCy resin. In the case of the BBz9 system, as shown in Fig. 9d, the fracture surface is much rougher than those of the BMI/BADCy resin, BBz3 and BBz6 systems, and there exists a large amount of ductile sunken areas, which can absorb the energy of fracture and hinder the crack propagation, exhibiting a typical rough feature. In addition, BBz12, as shown in Fig. 9e, has large ductile sunken areas in the matrix, and the surface scaly roughness is more obvious. In summary, the Bz-allyl/BMI/BADCy blend has a rough surface, more dimples and ductile sunken areas, and is of high ductility and thus can absorb more energy during the impact process. The possible reason is that the co-curing of Bz-allyl and BMI/BADCy has largely enhanced the toughness of the resin. Therefore, the impact strength of the composite with high Bz-allyl content is increased. The features of the fracture surfaces of Bz-allyl/BMI/BADCy systems accord well with the mechanical properties.
Dynamic mechanical analysis of the Bz-allyl/BMI/BADCy resin system
The DMA curves of the temperature dependence of tan
δ and storage modulus of the cured blends of BMI/BADCy, BBz3, BBz6, BBz9 and BBz12 are shown in Fig. 10 and 11. From the dynamic mechanical spectra, it is observed that the cured BMI/BADCy (Fig. 11) exhibits well-defined dynamic mechanical damping peaks centered at 245 and 308 °C, which are ascribed to the glass transitions of polycyanurate and polybismaleimide, respectively.21 The corresponding temperatures for BBz3 are 246 and 371 °C from Fig. 11. In contrast, only one dynamic mechanical damping peak at about 262 °C is observed for cured BBz9 (Fig. 11). Two glass transitions undoubtedly confirmed that the cured BMI/BADCy and cured BBz3 included two kinds of network. However, one single glass transition indicates that BBz6, BBz9 and BBz12 are a homogeneous network. There is only a single Tg so we speculate that there are further reactions between Bz-allyl and BMI/BADCy.
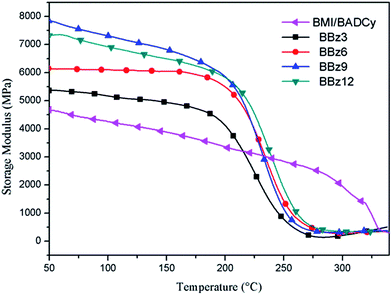 |
| Fig. 10 The storage modulus of Bz-allyl/BMI/BADCy systems. | |
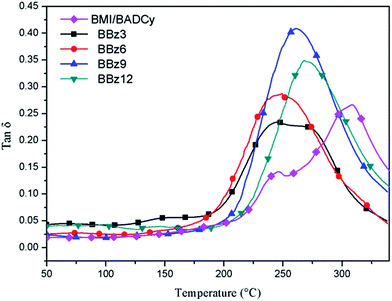 |
| Fig. 11 The tan δ of Bz-allyl/BMI/BADCy systems. | |
As can be seen, all of the composites exhibit a slightly higher storage modulus than the BMI/BADCy resin in the glassy state. This result may be attributed to the higher modulus of PBz-allyl. So the initial modulus of the Bz-allyl/BMI/BADCy blends was increased by the increase of Bz-allyl content. The Tg of the Bz-allyl/BMI/BADCy blends is summarized in Table 2. With the increase of Bz-allyl content, the Tgs of the cured blends shifted to the higher temperature. Based on the rubber elasticity theory and the following eqn (2),39 we calculated the crosslinking densities of the different crosslinked systems and the data are shown in Table 2.
where
ρ is the crosslinking density;
E is storage modulus in the rubbery region (
Tg + 40 °C);
φ is the front factor, which is unity for ideal rubbers;
R is the gas constant and
T is the absolute temperature.
28 It should be noted that this equation is applicable to polymer networks that have a rubbery plateau region. So it is strictly valid only for lightly crosslinked materials and therefore is used only to qualitatively compare the level of crosslinking in the cast resins.
40 It indicates that the addition of Bz-allyl into BMI/BADCy resin leads to increased
Tg because of the increased crosslinking degree of the Bz-allyl/BMI/BADCy blends. The crosslinking density increased with the increased proportion of Bz-allyl which may be attributed to the reaction between Bz-allyl and BMI/BADCy. However, the Bz-allyl/BMI/BADCy terpolymers still have high
Tg. Therefore, a conclusion can be drawn that the Bz-allyl/BMI/BADCy terpolymers show good heat resistance.
Table 2 Thermal properties of BOZ/BMI/BADCy thermosets
Sample |
Td5 (°C) |
Td10 (°C) |
Char yield (%) |
Tg (°C) |
Crosslinking density (103 mol m−3) |
BMI/BADCy |
441 |
446 |
35.8 |
245, 308 |
1.83 |
BBz3 |
427 |
433 |
46.6 |
246, 271 |
1.92 |
BBz6 |
405 |
415 |
45.2 |
248 |
2.15 |
BBz9 |
387 |
406 |
43.1 |
262 |
2.28 |
BBz12 |
379 |
401 |
43.3 |
267 |
2.32 |
Dielectric properties of Bz-allyl/BMI blends
The dielectric constant and dielectric loss factor of the BMI/BADCy resin and other modified systems with different content of Bz-allyl are shown in Fig. 12 and 13, respectively. It can be seen that the dielectric constant values and the dielectric loss factors of Bz-allyl/BMI/BADCy blends are smaller than BMI/BADCy in the frequency band from 10 to 60 MHz. This indicates that the Bz-allyl/BMI/BADCy terpolymers have good dielectric stability.
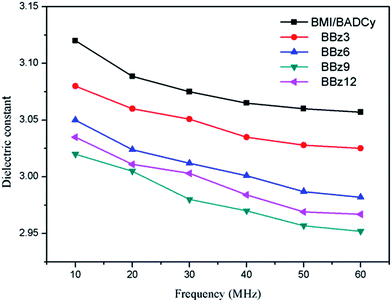 |
| Fig. 12 The dielectric constants of Bz-allyl/BMI/BADCy systems. | |
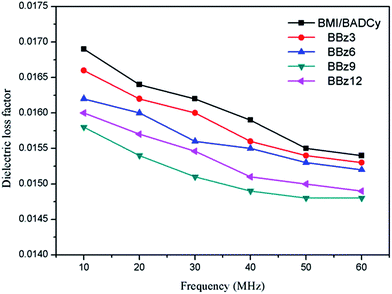 |
| Fig. 13 The dielectric loss factors of Bz-allyl/BMI/BADCy systems. | |
It is known to all that polybenzoxazine possesses a lower electrical capacitance than other thermosetting materials but also is less sensitive to changes in frequency.41 As Fig. 12 and 13 show, it can be seen that the dielectric constant values and the dielectric loss factors of the Bz-allyl/BMI/BADCy resin systems decreased with the content of Bz-allyl firstly. Then the dielectric constant values and the dielectric loss factors slightly increased with the further addition of Bz-allyl into the BMI/BADCy resin system. When the Bz-allyl content in the cured Bz-allyl/BMI/BADCy resins was 9 wt%, the dielectric constant values and the dielectric loss factors of the blends reached the minimum values of 3.02 and 0.0158 at 10 MHz, respectively. On the one hand, it was due to the triazine rings and diphenyl ether with low dielectric constant and dielectric loss factor. On the other hand, the dielectric constant could be reduced by the decrease in the dipole polarization in more highly crosslinked systems. The dielectric loss factor is also related to the crosslinking density based on its effect on the lagging of dipole polarization. But at high frequency there is no time for the polarization of dipoles. So the dielectric loss factor is insensitive to the crosslinking density at high frequency, which led to no obvious difference in the measured values of the dielectric loss factor. Wang et al. reported that the high cross-linking density and high viscosity during the final curing stage of cyanate esters hindered the mobility of residual cyanate groups.42 However, according to Wang’s conclusion, when Bz-allyl increased, the network structure of high crosslinking density formed by Bz-allyl/BMI/BADCy hindered the curing of BMI/BADCy, which caused the increase of the dielectric constant and the dielectric loss factor. Therefore, both the dielectric constant and loss factor of Bz-allyl/BMI/BADCy blends decrease compared with BMI/BADCy. It can be concluded that a suitable content of Bz-allyl in Bz-allyl/BMI/BADCy blends creates good dielectric properties and they retain good dielectric stability.
Thermal properties of the Bz-allyl/BMI/BADCy resin system
The derivative curves of the TGA thermograms for the terpolymers reveal a great deal of information about the network structure of polybenzoxazine.43,44 In this work, we attempted to increase its crosslinking density by adding Bz-allyl. The TGA thermograms and their derivative curves are shown in Fig. 14 and 15. Table 2 summarizes the results.
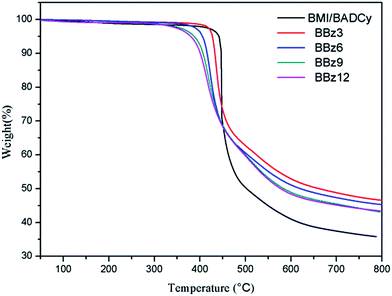 |
| Fig. 14 TGA curves of Bz-allyl/BMI/BADCy systems. | |
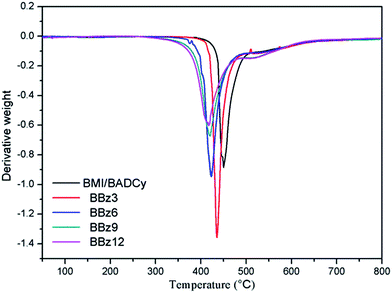 |
| Fig. 15 DTG curves of Bz-allyl/BMI/BADCy systems. | |
As can be seen from Fig. 15, different from an observable weight loss peak at around 420 °C on the differential curve of the Bz-allyl/BMI/BADCy systems, there was no obvious weight loss of Bz-allyl/BMI/BADCy blends under 350 °C. In Table 2, the Td5 of BMI/BADCy is 441 °C, while for BBz9 it is 387 °C. The char yield at 800 °C of BMI/BADCy is 35.8%, while for BBz9 it is 43.1%. The better thermal stability of BMI/BADCy compared to BBz9 may be attributed to the excellent thermal stability of BMI/BADCy, and more triazine rings in BMI/BADCy. The addition of Bz-allyl does not significantly sacrifice the thermal resistance of BMI/BADCy. However, the thermal stability of the BBz systems may be attributed to the increased cross-linking degree of the Bz-allyl/BMI/CE blends which results from the reaction between Bz-allyl which undergoes ring-opening polymerization to form polybenzoxazine, allyl groups react with BMI/CE resins and generate the phenolic hydroxyl group which increases the interactions between molecules.
Water absorption of the Bz-allyl/BMI/BADCy resin system
It is well recognized that BMI/BADCy is considered as a matrix for high-temperature aerospace structural applications and is expected to experience extreme environmental conditions for a prolonged period of time. Outstanding moisture resistance is a very important property of a material, especially those requiring stable high performance,45 because in general absorbed water will decline almost all properties of the original material including thermal, glass transition temperature, mechanical and dielectric properties, etc.38 Therefore, less water absorption is one important target of developing new BMI/BADCy composites with high performance. The water absorption of the Bz-allyl/BMI/BADCy systems is presented in Fig. 16. At the initial reaction stage, the water absorption values of the Bz-allyl/BMI/BADCy systems increase fast with increasing immersion time. With the prolongation of the immersion time, water absorption values increase slowly. Finally the water absorption values become more consistent. It also can be seen that the water absorption decreases from 2.52 to 2.28 wt% with the small addition (3 wt%) of Bz-allyl into BMI/BADCy resin, and which continually decreases with the continuous increase of Bz-allyl content in BMI/BADCy systems. In the case of the BBz9 system, its water absorption is only 1.77 wt%, which is much lower than those of the BMI/BADCy resin. However, the addition of Bz-allyl tends to increase the water resistance property. Apicella et al.46 proposed that there may be three ways for epoxy resins to absorb water: (1) formation of a polymer–diluent solution; (2) adsorption at hydrophilic sites; (3) adsorption on the surface of free volume elements. The improvement of the water resistant property of Bz-allyl/BMI/BADCy systems may be due to the more perfect network structure. Compared with BMI/BADCy, Bz-allyl has phenolic hydroxyl groups, which catalyze the reaction between BMI with BADCy; Bz-allyl also has allyl groups, which react with BMI/BADCy and form a perfect network structure after curing. Therefore, the Bz-allyl/BMI/BADCy systems have less free volume elements, which results in the lower water absorption values of the Bz-allyl/BMI/BADCy systems.
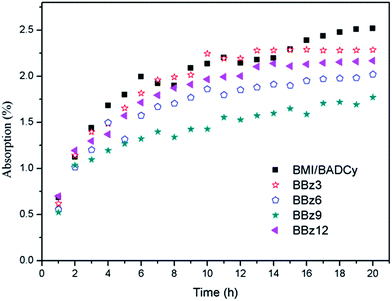 |
| Fig. 16 Water absorption of Bz-allyl/BMI/BADCy systems. | |
Conclusion
A kind of high-performance polymer composite has been fabricated using Bz-allyl/BMI/BADCy resin, in which the BMI/BADCy resin was modified with Bz-allyl in order to improve its dielectric, thermal and mechanical properties and the cross-linking degree after curing. The curing kinetics of Bz-ally/BMI/BADCy was investigated via non-isothermal DSC at different heating rates using the Flynn–Wall–Ozawa method. The incorporation of Bz-allyl can catalyze the reaction of BMI/BADCy resin and react with BMI/BADCy resin. BBz9 shows the maximum impact strength and flexural strength, which are 50% and 41% higher than those of BMI/BADCy resin, respectively. The Tg value for BBz9 is 262 °C. The Bz-allyl/BMI/BADCy blend with a suitable addition of Bz-allyl still shows good thermal resistance and moisture resistance. The appropriate addition of Bz-allyl does not significantly sacrifice the thermal resistance of the BMI/BADCy resin. Also, Bz-allyl can improve the dielectric properties of BMI/BADCy resin. The dielectric constant and loss of BBz9 are 2.95–3.02 and 0.0148–0.0158, respectively, over a relatively wide frequency band from 10 to 60 MHz. In addition, the SEM analysis shows that the Bz-allyl/BMI/BADCy systems have a distinct characteristic of ductile fracture. The outstanding integrated properties of the Bz-allyl/BMI/BADCy blend can be attributed to the network structure of high crosslinking density formed in Bz-allyl/BMI/BADCy blends.
Acknowledgements
This work was financially supported by the National Science Foundation of China (No. 51407134), the China Postdoctoral Science Foundation (No. 2014M562412) and the graduate starting seed fund of Northwestern Polytechnical University (No. Z2014175).
References
- C. P. R. Nair, Prog. Polym. Sci., 2004, 29, 401–498 CrossRef CAS PubMed.
- T. Takeichi and T. Agag, High Perform. Polym., 2006, 18, 777–797 CrossRef CAS PubMed.
- N. N. Ghosh, B. Kiskan and Y. Yagci, Prog. Polym. Sci., 2007, 32, 1344–1391 CrossRef CAS PubMed.
- H. D. Kim and H. Ishida, J. Appl. Polym. Sci., 2001, 79, 1207–1219 CrossRef CAS.
- X. Ning and H. Ishida, J. Polym. Sci., Part A: Polym. Chem., 1994, 32, 1121–1129 CrossRef CAS PubMed.
- H. D. Kim and H. Ishida, Macromolecules, 2003, 36, 8320–8329 CrossRef CAS.
- C. Sawaryn, K. Landfester and A. Taden, Macromolecules, 2011, 44, 7668–7674 CrossRef CAS.
- Y. C. Su, S. W. Kuo, D. R. Yei, H. Xu and F. C. Chang, Polymer, 2003, 44, 2187–2191 CrossRef CAS.
- T. Fang and D. A. Shimp, Prog. Polym. Sci., 1995, 20, 61–118 CrossRef CAS.
- B. Zhang, Z. Wang and X. Zhang, Polymer, 2009, 50, 817–824 CrossRef CAS PubMed.
- A. Chaplin, I. Hamerton, B. J. Howlin and J. M. Barton, Macromolecules, 1994, 27, 4927–4935 CrossRef CAS.
- B. Y. Ryu and T. Emrick, Macromolecules, 2011, 44, 5693–5700 CrossRef CAS.
- A. J. Guenthner, K. R. Lamison, V. Vij, J. T. Reams, G. R. Yandek and J. M. Mabry, Macromolecules, 2011, 45, 211–220 CrossRef.
- E. M. Maya, A. W. Snow and L. J. Buckley, Macromolecules, 2001, 35, 460–466 CrossRef.
- I. Hamerton and J. N. Hay, Polym. Int., 1998, 47, 465–473 CrossRef CAS.
- C. H. Lin, C. N. Hsiao, C. H. Li and C. S. Wang, J. Polym. Sci., Part A: Polym. Chem., 2004, 42, 3986–3995 CrossRef CAS PubMed.
- A. W. Snow and L. J. Buckley, Macromolecules, 1997, 30, 394–405 CrossRef CAS.
- A. J. Guenthner, G. R. Yandek, M. E. Wright, B. J. Petteys, R. Quintana, D. Connor, R. D. Gilardi and D. Marchant, Macromolecules, 2006, 39, 6046–6053 CrossRef CAS.
- R. H. Lin, A. C. Lee, W. H. Lu and C. W. Lin, J. Appl. Polym. Sci., 2004, 94, 345–354 CrossRef CAS PubMed.
- C. P. R. Nair and T. Francis, J. Appl. Polym. Sci., 1999, 74, 3365–3375 CrossRef CAS.
- C. P. R. Nair, T. Francis, T. M. Vijayan and K. Krishnan, J. Appl. Polym. Sci., 1999, 74, 2737–2746 CrossRef CAS.
- A. O. Owusu, G. C. Martin and G. T. Gotro, Polym. Eng. Sci., 1991, 31, 1604–1609 Search PubMed.
- J. M. Barton, I. Hamerton and J. R. Jones, Polym. Int., 1993, 31, 95–106 CrossRef CAS PubMed.
- J. M. Barton, I. Hamerton and J. R. Jones, Polym. Int., 1992, 29, 145–156 CrossRef CAS PubMed.
- I. Hamerton, J. M. Barton, A. Chaplin, B. J. Howlin and S. J. Shaw, Polymer, 2001, 42, 2307–2319 CrossRef CAS.
- I. Hamerton, J. R. Jones and J. M. Barton, Polym. Mater.: Sci. Eng., 1994, 71, 807–808 CAS.
- A. Chaplin, I. Hamerton, B. J. Howlin and J. M. Sarton, Macromolecules, 1994, 27, 4927–4935 CrossRef CAS.
- Y. Q. Wang, K. C. Kou, L. H. Zhuo, H. Chen, Y. Zhang and G. L. Wu, J. Polym. Res., 2015, 22, 1–8 CrossRef PubMed.
- A. Chaplin, I. Hamerton, B. J. Howlin and J. M. Sarton, Polym. Mater.: Sci. Eng., 1994, 71, 811–812 CAS.
- J. T. Wan, B. G. Li, H. Fan, Z. Y. Bu and C. J. Xu, Thermochim. Acta, 2010, 511, 51–58 CrossRef CAS PubMed.
- W. S. Choi, A. M. Shanmugharaj and S. H. Ryu, Thermochim. Acta, 2010, 506, 77–81 CrossRef CAS PubMed.
- G. L. Wu, K. C. Kou, L. H. Zhuo, Y. Q. Wang and J. Q. Zhang, Thermochim. Acta, 2013, 559, 86–91 CrossRef CAS PubMed.
- S. Vyazovkin and N. Sbirrazzuoli, Macromolecules, 1996, 29, 1867–1873 CrossRef CAS.
- S. Vyazovkin and C. A. Weght, Thermochim. Acta, 1999, 340, 53–68 CrossRef.
- R. A. Rafiq and P. S. Anthony, Thermochim. Acta, 1978, 26, 672–688 Search PubMed.
- Z. Wang, J. C. Zhao, Q. C. Ran, R. Q. Zhu and Y. Gu, React. Funct. Polym., 2013, 73, 668–673 CrossRef CAS PubMed.
- X. Y. Liu, Y. F. Yu and S. J. Li, Polymer, 2006, 47, 3767–3773 CrossRef CAS PubMed.
- A. J. Gu and G. Z. Liang, Polym.-Plast. Technol. Eng., 1997, 36, 681–694 CrossRef CAS PubMed.
- P. Musto, M. Abbate, G. Ragosta and G. Scarinzi, Polymer, 2007, 48, 3703–3716 CrossRef CAS PubMed.
- H. Ishida and D. J. Allen, Polymer, 1996, 37, 4487–4495 CrossRef CAS.
- H. Ishida and D. J. Allen, J. Polym. Sci., Part B: Polym. Phys., 1996, 34, 1019–1030 CrossRef CAS.
- J. Y. Shieh, S. P. Yang, M. F. Wu and C. S. Wang, J. Polym. Sci., Part A: Polym. Chem., 2004, 42, 2589–2600 CrossRef CAS PubMed.
- K. Hemvichian and H. Ishida, Polymer, 2002, 43, 4391–4402 CrossRef CAS.
- K. Hemvichian, A. Laobuthee, S. Chirachanchai and H. Ishida, Polym. Degrad. Stab., 2002, 76, 1–15 CrossRef CAS.
- K. Yang, M. J. Xu and B. Li, Polym. Degrad. Stab., 2013, 98, 1397–1406 CrossRef CAS PubMed.
- A. Apicella, R. Tessieri and C. De Cataldis, J. Membr. Sci., 1984, 18, 211–225 CrossRef CAS.
|
This journal is © The Royal Society of Chemistry 2015 |
Click here to see how this site uses Cookies. View our privacy policy here.