DOI:
10.1039/C5RA13771C
(Paper)
RSC Adv., 2015,
5, 75547-75554
Synthesis and characterization of a novel fluorinated bismaleimide via a nucleophilic addition–elimination reaction and its polymeric networks†
Received
13th July 2015
, Accepted 28th August 2015
First published on 28th August 2015
Abstract
The commercially available octafluorocyclopentene (OFCP) as both a fluorinated building block and a linker has been successfully utilized to prepare a new fluorinated bismaleimide monomer OFCP-BMI via a nucleophilic addition–elimination reaction. The resulting OFCP-BMI is characterized by 1H, 13C, 19F nuclear magnetic resonance (NMR) spectroscopy, attenuated total reflectance Fourier transform infrared spectroscopy (ATR-FTIR), and high resolution mass spectrometry. The monomer OFCP-BMI reacts with a free radical initiator or self-cures to prepare its resins. No obvious glass transition temperature (Tg) below 260 °C was observed for the self-curing resin suggesting that a highly cross-linked polymeric network was formed. The self-curing resin exhibits excellent thermal stability with the on-set weight loss temperature (Ton-setd) of 445 °C and a 50% char yield at 800 °C under a nitrogen atmosphere. The resin obtained by a free radical polymerization process exhibits less thermal stability and a lower char yield than the self-curing resin due to its lower cross-linking density. The self-curing resin exhibits a low dielectric constant (Dk = 2.5).
Introduction
Bismaleimide (BMI) resins are an important class of thermosetting polymers for applications in advanced composites,1–3 electronics, and aerospace due to their relatively low cost and a combination of various excellent properties, such as, excellent processability, superior thermal stability, outstanding dielectric property, high modulus, low flammability, and high humidity resistance.4,5 The activated double bond of maleimide rings in BMI monomer can undergo several polymerization reactions, such as free radical polymerization,6 thermal self-curing, anionic polymerization,7,8 polymerization through Michael addition with diamines9,10 or di-thiols,11 polymerization through Diels–Alder reactions with bis-furan molecules or dienes,12,13 and photo-induced copolymerization with vinyl ether.14–16
Fluorinated polymers exhibit a series of unique features such as outstanding thermostability, chemical resistance, low surface energy, low refractive index, low dielectric constants, good water and oil repellence.17,18 Hence, they have been used in many areas: non-linear optical polymers,18–21 micro-porous organic polymers for gas separations,22,23 photonics,24 thermostable polymers for aerospace,25 proton exchange membranes (PEMs) for fuel cells,26 and hybrid composites.27,28 Introduction of fluorinated structures in polymeric networks often enhance performance of their properties.14 Several studies demonstrated the incorporation of fluorine containing building blocks into bismaleimide materials can effectively reduce their dielectric constants (Dk) and moisture adsorption and modify their surface properties.29,30 However, only limited fluorinated bismaleimides were prepared due to the availability and cost of the fluorinated building blocks for the synthesis of bismaleimides.31,32 It encourages us to look for new fluorinated building blocks and novel synthetic methods to effectively synthesize fluorinated bismaleimides with the prospects of commercialization.
The commercially available octafluorocyclopentene (OFCP) exhibits unique chemistry.33 OFCP is a toxic, non-flammable, low boiling point (27 °C), volatile, colourless liquid with a slight distinctive odour. The reaction of OFCP with phenols typically affords mono and/or di-substituted PFCP aryl ether derivatives with good to excellent isolated yields. Taking advantage of this unique chemistry, a new class of PFCP aryl ether polymers has been developed, which OFCP and mono-substituted PFCP aryl ether molecules have been used as monomers (AA type and AB type, Scheme 1, Method 1).33,34 However, due to the volatility of OFCP, it is not easy to control the stoichiometry of monomers so that the molecular weights of resulting PFCP aryl ether polymers are not high. Furthermore, the mono-substituted AB type monomers are not very stable. It is also difficult to incorporate functionalities into PFCP aryl ether polymers via Method 1. Usually functional biphenols, which are usually difficult to synthesize, are needed to yield functional polymers. Therefore, it would be beneficial for us to develop an effective method to prepare PFCP aryl ether polymers or functional polymers.
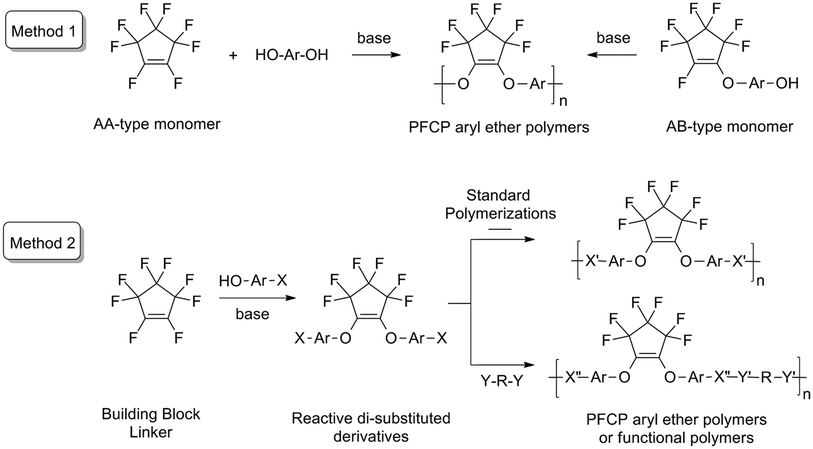 |
| Scheme 1 The two synthetic methods to PFCP aryl ether polymers. | |
In early 1990s, a variety of di-substituted PFCP aryl ether molecules were synthesized for the potential application as electrical insulating agents.35 Beyond these early works, further development in the preparation of new di-substituted PFCP aryl ether molecules was not reported in the recent literature. Using reactive di-substituted PFCP aryl ether derivatives as a monomer, an alternative synthetic method to PFCP aryl ether polymers or functional polymers was developed (Scheme 1, Method 2). In Method 2, the reactive di-substituted PFCP aryl ether derivatives (containing reactive X groups) can be prepared from the reaction of OFCP with mono-functional phenols via the facile nucleophilic addition–elimination process. The reactive di-substituted PFCP aryl ether derivatives can polymerize with themselves or other reactive or functional monomers to afford PFCP aryl ether polymers or functional polymers via standard polymerization processes taking advantage of the chemistry of reactive groups (X and Y) in the monomers. Using Method 2, various fluorinated polymeric materials can be prepared from OFCP, which provides us a great opportunity to develop next-generation fluorinated polymers.
In this study, the alternative synthetic strategy to PFCP aryl ether polymers (Scheme 1, Method 2) was successfully demonstrated by the preparation of a novel fluorinated bismaleimide monomer OFCP-BMI and its polymeric networks (Scheme 2). OFCP-BMI was readily synthesized via the reaction of OFCP with maleimide-functionalized phenol under a mild reaction condition (Scheme 2) and exhibits good solubility in various organic solvents. The resins R1 and R2 were prepared via the thermally activated polymerization without catalyst and free radical polymerization using AIBN as an initiator, respectively. The properties of the resulting resins were studied and discussed in the paper. The dielectric properties of the self-curing resin were also investigated.
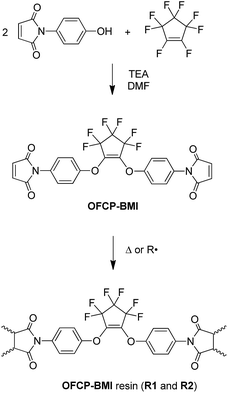 |
| Scheme 2 The synthesis of monomer OFCP-BMI and its resins R1 and R2. | |
Experimental section
Materials
N-(4-Hydroxyphenyl)maleimide was synthesized by following published procedure.36 Octafluorocyclopentene (97%) was purchased from SyQuest Laboratories and used as received. Deuterated solvents were purchased from Mallinckrodt Chemicals. All other chemicals and solvents were purchased from Alfa Aesar or Acros and used as received unless otherwise stated.
Instrumentation
1H, 13C and 19F NMR spectra were recorded on a Bruker 500 MHz and chemical shifts were measured in ppm (δ) with reference to internal tetramethylsilane (0 ppm), deuterated chloroform (77 ppm), and trichlorofluoromethane (0 ppm) for 1H, 13C and 19F NMR, respectively. Differential scanning calorimetry (DSC) analysis was performed on a Mettler Toledo DSC 1 system in nitrogen at a heating rate of 10 °C min−1. The glass transition temperature (Tg) was obtained from a second heating cycle using Star E version 10.0 software suite. Thermal gravimetric analysis (TGA) was performed on a Mettler-Toledo TGA/DSC 1 LF instrument in nitrogen at a heating rate of 10 °C min−1 up to 800 °C. High-resolution mass spectrometry (HR-MS) were obtained at the Mass Spectrometry Laboratory, Department of Chemistry and Biochemistry, University of Texas at Austin, 1 University Station A5300, Austin, TX 78712-0165. The dielectric constants of the polymer film were investigated at room temperature with a Casade Microtech Probe Station. The thickness of thin film and the diameter of top circle electrode were measured with a scanning electron microscope (SEM) (Zeiss: Supra 40). Single crystal X-ray diffraction data was collected at low temperature (Oxford Cryosystems cryostream, T = 100 K) on a sample using a Bruker Kappa D8 Quest diffractometer equipped with Incoatec microfocus Mo Kα radiation source and Photon 100 CMOS detector. Afterwards, the data was integrated, scaled, and evaluated for space group determination using Bruker SAINT, SADABS (multi-scan absorption correction), and XPREP, respectively. A starting model was generated using SHELXT (intrinsic phasing method)37 and further atomic site assignments, anisotropic refinement of non-hydrogen atomic positions, and addition of “riding” hydrogen atomic sites were completed with SHELXL2014.38 Gel permeation chromatography (GPC) data were collected in chloroform from a Waters 2690 Alliance System with photodiode array detection. GPC sample was eluted through Polymer Labs PLGel 5 mm Mixed-D and Mixed-E columns at 35 °C. Molecular weights were obtained using polystyrene as a standard (Polymer Labs Easical PS-2).
Synthesis of bismaleimide OFCP-BMI
To a 50 mL round-bottom flask equipped with a magnetic stirrer, N-(4-hydroxyphenyl) maleimide (3.1 g, 16.4 mmol), triethylamine (2.3 mL, 16.4 mmol), and DMF (15 mL) were introduced at 0 °C. Then octafluorocyclopentene (1 mL, 7.5 mmol) was then introduced with a syringe at once. The reaction solution was stirred in an ice bath for one hour, and then was stirred at 50 °C for about 2 h until all the mono-substituted intermediate disappeared. The solvent was then removed in vacuum, the crude product was dissolved in ethyl acetate (100 mL), sequentially washed with saturated Na2CO3 solution, DI water and brine, and then dry using MgSO4. The solvent was removed by rotary evaporator and the raw product was purified by column chromatography (hexane
:
ethyl acetate = 9
:
1) to afford a yellow solid (2.5 g, 61%). 1H NMR (500 MHz, acetone-d6) δ 7.23 (d, J = 8.9 Hz, 4H), 7.04 (s, 4H), 6.96 (d, J = 8.9 Hz, 4H); 19F NMR (471 MHz, acetone-d6) δ −115.18 (t, J = 3.9 Hz), −130.61 to −130.81 (m); 13C NMR (126 MHz, acetone-d6) δ 169.49 (s), 152.85 (s), 134.47 (s), 129.01 (s), 128.24 (s), 117.65 (s); ATR-FTIR (cm−1) ν 631, 692, 716, 824, 831, 841, 955, 976, 1001, 1016, 1065, 1119, 1146, 1171, 1194, 1215, 1279 (C–O), 1352, 1393, 1506, 1684, 1713; HR-MS (CI): calcd for C25H12N2O6F6, 550.0600; found, 550.0604.
Polymerizations of OFCP-BMI monomer
Thermally activated polymerization. OFCP-BMI monomer (35 mg) was put in a TGA ceramic pan and was heated at 290 °C for 3 h with a nitrogen flow (50 mL min−1). A transparent brown and clear insoluble solid was formed (34.7 mg, 99%). ATR-FTIR (cm−1) ν 833, 978, 1005, 1117, 1152, 1165, 1200, 1287, 1346, 1395, 1506, 1709.
Free radical polymerization of OFCP-BMI. OFCP-BMI monomer (274 mg, 0.5 mmol), toluene (2 mL), and 2,2-azobisisobutyronitrile (1.7 mg) were added into a single-neck round bottom flask. Reaction mixture was heated at 70 °C under N2 atmosphere for 3 days. The toluene was removed under vacuum. The resulting residue was dissolved in a minimal amount of dichloromethane and precipitated by methanol. The resulting solid material was filtered off, washed with MeOH and dried at 50 °C under reduced pressure affording a soluble yellow powder (130 mg, 47% yield). ATR-FTIR (cm−1) ν 831, 978, 1005, 1115, 1165, 1202, 1287, 1346, 1366, 1385, 1508, 1711.
Isothermal curing studies of OFCP-BMI monomer. Six 40 μL DSC aluminium pans containing OFCP-BMI monomer (5–6 mg) were put into a small air-circulating oven. The samples were heated at 200 °C (120 min), 220 °C (140 min), 240 °C (190 min), 260 °C (120 min), 280 °C (120 min) and 300 °C (120 min). At the end of each temperature stage, one pan was taken out of the oven and cooled to room temperature. ATR-FTIR spectra were measured for all samples.
Preparation of the thin film of self-curing resin for the measurement of Dk values. The parallel plate capacitors were fabricated on a gold-coated glass slide (20 mm × 20 mm) by spin-coating a solution of the appropriate monomer in THF (50 mg mL−1) at 1500 rpm for 60 s. The slide was placed into a plastic desiccator overnight under vacuum to completely remove the solvent and was heated at air atmosphere in a small oven at 200 °C/1 h, 220 °C/3 h, and 240 °C/1 h. Finally, gold was deposited via vacuum evaporation on the surface of the film as a top electrode. The thickness of the polymer film on a glass substrate was measured using scanning electron microscopy (SEM) technology (Fig. S7†).
Results and discussion
Synthesis and characteristics of bismaleimide monomer OFCP-BMI
After a systematic literature search, we find that there is still some room for the development of novel di-substituted PFCP aryl ether derivatives for the preparation of polymeric materials. In this study, a novel di-substituted PFCP aryl ether bismaleimide derivative OFCP-BMI was designed and readily synthesized via the reaction of maleimide-functionalized phenol with OFCP shown in Scheme 2. N-(4-Hydroxyphenyl) maleimide was readily prepared from 4-aminophenol by following a published one-pot procedure.36,39 The reaction of OFCP with N-(4-hydroxyphenyl)maleimide was carried out in the presence of triethylamine using DMF as a solvent at 0 °C, and both mono-substituted intermediate and di-substituted bismaleimide product were rapidly formed as observed by thin layer chromatography (TLC). At the mean time, the reaction solution changed to red color slowly. After stirring at 0 °C for about one hour, the reaction mixture was heated to 50 °C to accelerate the conversion of mono-substituted intermediate to monomer OFCP-BMI. After about 2 h, the mono-substituted intermediate was completely consumed as observed by TLC and the reaction was stopped. The yellowish monomer OFCP-BMI was obtained in a good isolated yield (61%) after isolation from a column chromatography procedure. OFCP-BMI exhibits good solubility in various solvents such as THF, acetone, 1,4-dioxane, DMF, DMSO, and NMP. It seems to be more soluble in THF and acetone than DMSO, which makes it more useful than normal aromatic bismaleimides (poor solubility in ordinary solvents) in advanced composite and other applications.40
Fig. 1 shows the NMR spectra of bismaleimide monomer OFCP-BMI in acetone-d6. The 1H NMR spectrum of monomer OFCP-BMI confirmed its chemical structure as follows: the maleimide protons appear as a singlet at 7.04 ppm, and the aromatic protons appear as two doublets at 7.23 ppm and 6.96 ppm, respectively. The 19F-NMR spectrum shows well-integrated two fluorine peaks corresponding to the PFCP ring substituted in a symmetrical fashion (Fig. 1). The 13C NMR spectrum shows a singlet at 152.9 ppm corresponding to the carbon atoms of C
C of maleimide ring (Fig. S1†). The chemical structure of monomer OFCP-BMI was further confirmed using ATR-FTIR spectrum. Fig. 2 shows the ATR-FTIR spectrum of OFCP-BMI monomer. The characteristic absorption peaks can be seen from the spectrum such as ν (cm−1): 1713 (C
O); 1684 (C
C for the PFCP ring); 1506 (para disubstitution of benzene ring), 1393(C–N–C), 1279 (C–O vinyl ether), 1146 (C–F), 831 (para disubstitution of benzene ring), 824 (maleimide –C
C–), 692 (C
C from maleimide ring), and 631 (C–F).
 |
| Fig. 1 1H NMR (top) and 19F NMR (bottom) spectra of monomer OFCP-BMI. | |
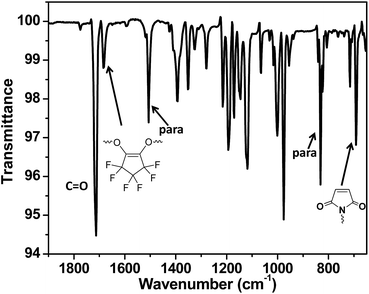 |
| Fig. 2 The ATR-FTIR spectrum of OFCP-BMI. | |
To further understand the solid-state nature of OFCP-BMI monomer, X-ray analysis was conducted. OFCP-BMI monomer was purified using column chromatography with hexane and ethyl acetate mixture as the eluent. The purified monomer OFCP-BMI was obtained as a yellow powder. Recrystallization of OFCP-BMI from THF with slow evaporation resulted in the afforded X-ray quality crystalline solid. The crystal structure for OFCP-BMI is shown in Fig. 3 and S2.† The crystal structure of OFCP-BMI shows very interesting characteristics. Two sp2 hybridized carbons in the fluorinated five member ring and two substituent oxygen atoms (O–C
C–O) almost locate at the same plane with a torsion angle ∼5° The two phenyl rings are nearly parallel to each other with a dihedral angle of ∼9° and strongly interact with each other by π–π stacking (the distance between two phenyl rings is 3.427 Å, see Fig. S2†). The maleimide rings are nearly orthogonal to each other with a dihedral angle of ∼86°. The lone pair electrons on one carbonyl oxygen of the maleimide ring seem strongly interact with π electrons on the other maleimide ring by p–π interaction (the distance between oxygen atom and maleimide ring is 3.061 Å, see Fig. S2†).
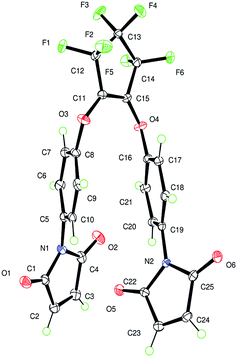 |
| Fig. 3 ORTEP representation of OFCP-BMI crystal structure. | |
As shown in Fig. S2,† the intermolecular interactions are also strong. The phenyl ring of one molecule strongly interacts with another phenyl ring of another molecule by π–π stacking (the distance between two phenyl rings is 3.814 Å). The lone pair electrons on one carbonyl oxygen of maleimide ring in one molecule seem also interact with π electrons on the maleimide ring of another molecule by p–π interaction (the distance between oxygen atom and maleimide ring is 3.122 Å). There are also close contacts between neighbouring fluorine atoms (the intermolecular distance between fluorine atoms is 2.770 Å).
The strong intramolecular and intermolecular interactions cause OPFC-BMI molecules to tightly pack with each other (Fig. S3†), which may be the reason that monomer OFCP-BMI exhibits a high melting point.
The thermal behaviour of monomer OFCP-BMI was investigated by differential scanning calorimetry (DSC). As showed in Fig. 4, the DSC curve of the first heating cycle was characterized by a sharp endothermic transition (Tend) in the temperature range 175–195 °C, presumably due to melting (Tm = 190 °C). The monomer also exhibited an exothermic transition associated with a polymerization reaction of residual double bonds of maleimide moieties. The onset temperature of the exothermic process is 250 °C and the curing exothermic process reaches its maximum at 290 °C. The DSC second heating curve did not show obvious glass transition under 350 °C, indicating a highly cross-linked polymer network was formed during the first heating cycle.
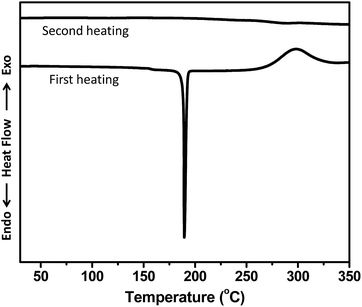 |
| Fig. 4 DSC thermograms of OFCP-BMI. | |
Thermal curing probed by ATR-FTIR
Fig. 5 shows the ATR-FTIR spectra of the resulting residue after a cumulative curing process by heating samples at each temperature for 120 min from 200 °C to 300 °C under air atmosphere, respectively. ATR-FTIR spectra indicated the curing process was obviously taking place beginning with 240 °C, which is close to the on-set temperature (250 °C) of the exothermic curing process observed from DSC studies. After heating to 260 °C, the characteristic absorption of the maleimide double bond at 690 cm−1 is almost disappeared. The characteristic absorption of double bond of PFCP aryl ether moiety at 1684 cm−1 seemed to be inert when the monomer was heated to 220 °C. After the self-curing process took place, the characteristic absorption peak of carbonyl groups became broader. As a result, it is hard to identify the characteristic absorption peak of double bonds of PFCP aryl ether moieties.
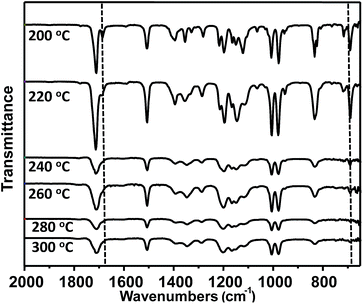 |
| Fig. 5 ATR-FTIR spectra of cured samples. | |
Polymerizations of bismaleimide monomer OFCP-BMI
Thermally mediated polymerization. The thermally induced polymerization was performed by heating OFCP-BMI monomer at 290 °C for 3 h. A transparent light brown, brittle, highly cross-linked resin R1 was obtained, which is insoluble in most of solvents. During the curing period, the weight loss was very small (less than 1%) according to TGA measurements, which indicated the yield of this self-curing process is over 99% (Table 1). ATR-FTIR spectrum for this self-curing resin shows on characteristic absorption peak the maleimide double bond at 690 cm−1 indicating the complete formation of polymeric network (Fig. S4†).
Table 1 The polymerization conditions and characterization of R1 and R2
Resin |
System |
Mn (g mol−1) |
PDI |
Conditions |
Yield |
Ton-setd |
Char yield |
R1 |
— |
— |
— |
290 °C (3 h) |
99% |
445 °C |
50% |
R2 |
AIBN/toluene |
1155 |
1.22 |
90 °C (24 h) |
47% |
150/365 °C |
45% |
Free radical polymerization using AIBN as an initiator. Free radical polymerizations of OFCP-BMI using AIBN as an initiator were carried out using toluene as a solvent, which afforded soluble light brown polymer powder R2 in a 47% yield (Table 1). This soluble oligomer can give us some information on the polymerization process. As shown in Fig. S4,† the resulting resin R2 shows the similar IR absorption pattern with the resin R1. Fig. S5† shows the 1H NMR spectrum of R2. The signal of the phenyl group appears as a broad hump from 6 to 8 ppm, and some sharp peaks appear on the top of this broad hump representing the phenyl group and vinyl hydrogen of end groups. The signal of the methine group appears from 3 to 5 ppm as a broad hump. Fig. S6† shows the 19F NMR spectrum of R2. The 19F spectrum of R2 in DMSO-d6 shows two broad peaks (one is at −113.14 ppm with three small shoulder peaks at −114.3 ppm, -115.0 ppm, and −115.6 ppm, and the other one is at −129.0 ppm with a small should peak at −129.6 ppm), which represent the two different environments of fluorine atoms.
Thermal properties of resulting polymers R1 and R2. The thermal properties of the self-curing resin R1 were investigated by TGA in air and nitrogen atmospheres, respectively. As showed in Fig. 6, the self-curing resin R1 exhibits excellent thermal stability and a one-step degradation process with an on-set degradation temperature at 430 °C (in air atmosphere) or 445 °C (in N2 atmosphere). The char yield of R1 at 800 °C is 50% at nitrogen atmosphere, which indicates it can be used as flame resistant materials.
 |
| Fig. 6 Thermo gravimetric curves of R1 and R2. | |
The thermal properties of resin R2 was investigated by TGA in nitrogen atmospheres. As showed in Fig. 6, the resin R2 exhibits a two-step degradation process. The first step degradation takes place at around 150 °C with about 4% weight loss, which may be due to some solvents in trapped in the polymeric network, and the second degradation (major degradation) takes place at around 365 °C. The resin R1 exhibits higher thermal stability than the resin R2 due to its much higher cross-linking density. Although R1 and R2 have a big difference in cross-linking density, they have a small difference (about 5%) in char yield at 800 °C under N2 atmosphere (Table 1).
Dielectric property of cured PFCP bismaleimide resin. Bismaleimide resins with the combination of excellent properties have been attracted a lot of attention in the electronic and aerospace industries. However, common bismaleimides have relatively high dielectric constants, which limit their application in aforementioned fields.41 The dielectric constants of typical bismaleimide resins are in the range of 3–5 at high frequency (1 MHz).42 The dielectric constant of neat 4,4′-bismaleimidodiphenyl methane resin is about 3.09 at 10 GHz.43 Copolymerization of BMI with cyanate ester can effectively achieve low dielectric constants composite materials.44 Another effective method for decreasing dielectric constant of polymeric materials is introducing fluorine atoms due to its high electronegativity and low electric polarity.45 The variation of the dielectric constant with frequency of the applied field is shown in Fig. 7. The dielectric constants were observed to vary from 5.7 to 2.5 for cured OFCP-BMI resin. The dielectric constant of cured OFCP-BMI resin is lower than the typical bismaleimide resin in the high frequency range, due to the introduction of fluorine content. The dielectric constant of cured OFCP-BMI resin is similar with the previous result on the fluorinated bismaleimide resins.46 However, the fluorinated building block we choose is much cheaper than previous reported fluorinated building blocks.
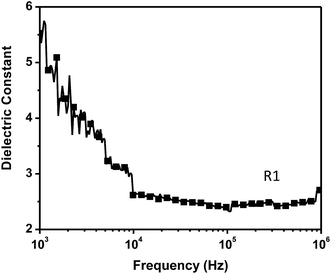 |
| Fig. 7 Dielectric constant versus frequency of cured bismaleimide resin at RT. | |
Conclusions
In conclusion, the new strategy (Scheme 1, Method 2) to PFCP aryl ether polymers using reactive di-substituted PFCP aryl ether molecules as monomers was successfully demonstrated by the preparation of new fluorinated bismaleimide resins. In this study, the reactive group X is the maleimide group. The new di-substituted PFCP aryl ether derivative OFCP-BMI was prepared via the nucleophilic addition–elimination reaction of OFCP with maleimide-functionalized phenol. The monomer OFCP-BMI is soluble in various common organic solvent such as THF, acetone, DMSO, and DMF. The curing profile of OFCP-BMI monomer was studied in air atmosphere and via DSC technique under nitrogen atmosphere. Two polymerization methods, thermal mediated polymerization and free radical polymerization using AIBN as an initiator, have been used to prepared polymeric networks R1 and R2, respectively. The self-curing OFCP-BMI resin is highly cross-linked, tough, and brittle, which is not easy to be recycled. The self-curing resin R1 exhibits high thermal stability with a Ton-setd around 445 °C and a char yield of 50% at 800 °C under nitrogen atmosphere. The self-curing OFCP-BMI resin exhibits improved dielectric properties as compared with typical non-fluorinated bismaleimide resin with a low dielectric constant (Dk = 2.5). Overall, the newly developed fluorinated bismaleimide OFCP-BMI is easy to scale up, exhibits many outstanding properties, and is suitable for advance composite applications and many other applications. By choosing appropriate X groups in Method 2, a large number of new fluorinated polymeric materials can be readily prepared from OFCP.
Acknowledgements
The authors thank Department of Chemistry and Alan G. MacDiarmid Nanotech Institute, the NMR facility of Chemistry Department (NSF, Grant CHE-1126177) and the University of Texas at Dallas for their support. We thank Dr Scott T. Iocano for his comments that greatly improved the manuscript.
Notes and references
- Q. Cheng, J. Bao, J. Park, Z. Liang, C. Zhang and B. Wang, Adv. Funct. Mater., 2009, 19, 3219–3225 CrossRef CAS PubMed
. - R. J. Morgan, R. J. Jurek, A. Yen and T. Donnellan, Polymer, 1993, 34, 835–842 CrossRef CAS
. - G. Liang and X. Hu, Polym. Int., 2004, 53, 670–674 CrossRef CAS
. - Y. L. Liu, Y. L. Liu, R. J. Jeng and Y.-S. Chiu, J. Polym. Sci., Part A: Polym. Chem., 2001, 39, 1716–1725 CrossRef CAS PubMed
. - Z.-Y. Wang, J.-C. Ho and W.-J. Shu, J. Appl. Polym. Sci., 2012, 123, 2977–2984 CrossRef CAS PubMed
. - R. C. P. Cubbon, Polymer, 1965, 6, 419–426 CrossRef CAS
. - T. Hagiwara, T. Shimizu, T. Someno, T. Yamagishi, H. Hamana and T. Narita, Macromolecules, 1988, 21, 3324–3327 CrossRef CAS
. - M. Takeishi, S. Arimori, N. Iwasaki, K. Sekiya and R. Sato, Polym. J., 1992, 24, 365–373 CrossRef CAS
. - X. Zhang, Y.-H. Jin, H.-X. Diao, F.-S. Du, Z.-C. Li and F.-M. Li, Macromolecules, 2003, 36, 3115–3127 CrossRef CAS
. - C. S. Wu, Y. L. Liu and Y.-S. Chiu, Polymer, 2002, 43, 1773–1779 CrossRef CAS
. - H.-J. Yen and G.-S. Liou, J. Mater. Chem., 2010, 20, 4080–4084 RSC
. - C. Goussé, A. Gandini and P. Hodge, Macromolecules, 1998, 31, 314–321 CrossRef
. - Q. Tian, Y. C. Yuan, M. Z. Rong and M. Q. Zhang, J. Mater. Chem., 2009, 19, 1289–1296 RSC
. - A. Soules, C. Pozos Vázquez, B. Améduri, C. Joly-Duhamel, M. Essahli and B. Boutevin, J. Polym. Sci., Part A: Polym. Chem., 2008, 46, 3214–3228 CrossRef CAS PubMed
. - C. E. Hoyle, S. C. Clark, S. Jonsson and M. Shimose, Polymer, 1997, 38, 5695–5697 CrossRef CAS
. - P. Kohli, A. B. Scranton and G. J. Blanchard, Macromolecules, 1998, 31, 5681–5689 CrossRef CAS
. - B. Ameduri, in Encyclopedia of Polymer Science and Technology, John Wiley & Sons, Inc., 2002, DOI:10.1002/0471440264.pst575
. - S. T. Iacono, S. M. Budy, J. Y. Jin and D. W. Smith, J. Polym. Sci., Part A: Polym. Chem., 2007, 45, 5705–5721 CrossRef CAS PubMed
. - J. Wu, J.-H. Liou, C. Y. Shu, Y. Patel, R. Menon, C. Santucci, S. T. Iacono, D. W. Smith Jr and B. M. Novak, React. Funct. Polym., 2015, 93, 38–46 CrossRef CAS PubMed
. - J. Wu, S. T. Iacono, G. T. McCandless, D. W. Smith and B. M. Novak, Chem. Commun., 2015, 51, 9220–9222 RSC
. - D. K. Dei, B. R. Lund, J. Wu, D. Simon, T. Ware, W. E. Voit, D. MacFarlane, S. M. Liff and D. W. Smith, ACS Macro Lett., 2012, 1, 35–39 Search PubMed
. - D.-P. Liu, Q. Chen, Y.-C. Zhao, L.-M. Zhang, A.-D. Qi and B.-H. Han, ACS Macro Lett., 2013, 2, 522–526 CrossRef CAS
. - Y. Zhao, K. X. Yao, B. Teng, T. Zhang and Y. Han, Energy Environ. Sci., 2013, 6, 3684–3692 CAS
. - M.-C. Oh, K.-J. Kim, W.-S. Chu, J.-W. Kim, J.-K. Seo, Y.-O. Noh and H.-J. Lee, Polymers, 2011, 3, 975–997 CrossRef CAS PubMed
. - N. Venkat, T. D. Dang, Z. Bai, V. K. McNier, J. N. DeCerbo, B.-H. Tsao and J. T. Stricker, Mater. Sci. Eng., B, 2010, 168, 16–21 CrossRef CAS PubMed
. - R. Souzy, B. Ameduri, B. Boutevin, G. Gebel and P. Capron, Solid State Ionics, 2005, 176, 2839–2848 CrossRef CAS PubMed
. - C. Bi, H. Zhang, S. Xiao, Y. Zhang, Z. Mai and X. Li, J. Membr. Sci., 2011, 376, 170–178 CrossRef CAS PubMed
. - J. Park, J.-M. Oh, S. E. Creager and D. W. Smith Jr, Chem. Commun., 2012, 48, 8225–8227 RSC
. - G. C. Tesoro and S. M. Pendharkar, Polym. Adv. Technol., 1994, 5, 521–528 CrossRef CAS PubMed
. - A. Nagai and A. Takahashi, Polym. J., 1994, 26, 357–362 CrossRef CAS
. - A. C. Misra and G. Tesoro, Polymer, 1992, 33, 1083–1089 CrossRef CAS
. - G. C. Tesoro and S. M. Pendharkar, Polym. Adv. Technol., 1994, 5, 521–528 CrossRef CAS PubMed
. - J. M. Cracowski, B. Sharma, D. K. Brown, K. Christensen, B. R. Lund and D. W. Smith, Macromolecules, 2012, 45, 766–771 CrossRef CAS
. - R. Campos, A. A. Mansur, C. H. Cook, B. Batchelor, S. T. Iacono and D. W. Smith Jr, J. Fluorine Chem., 2014, 166, 60–68 CrossRef CAS PubMed
. - M. Negele, D. Bielefeldt, T. Himmler, A. Marhold, Preparation of 1,2-bis(aryloxy)perfluorocycloalkenes and analogs as electrical insulators, DE3817626A1, 1989 Search PubMed
. - I. A. Mohammed and A. Mustapha, Molecules, 2010, 15, 7498–7508 CrossRef CAS PubMed
. - G. M. Sheldrick, Acta Crystallogr., Sect. A: Found. Adv., 2015, 71, 3–8 CrossRef PubMed
. - G. M. Sheldrick, Acta Crystallogr., Sect. C: Struct. Chem., 2015, 71, 3–8 CrossRef PubMed
. - Y. Wang, J. Zhao, Y. Yuan, S. Liu, Z. Feng and Y. Zhao, Polym. Degrad. Stab., 2014, 99, 27–34 CrossRef CAS PubMed
. - Y. L. Liu, Y. L. Liu, R. J. Jeng and Y.-S. Chiu, J. Polym. Sci., Part A: Polym. Chem., 2001, 39, 1716–1725 CrossRef CAS PubMed
. - J. Fan, X. Hu and C. Y. Yue, J. Polym. Sci., Part B: Polym. Phys., 2003, 41, 1123–1134 CrossRef CAS PubMed
. - C.-S. Wang, T.-S. Leu and K.-R. Hsu, Polymer, 1998, 39, 2921–2927 CrossRef CAS
. - G. Liang, Z. Zhang, J. Yang and X. Wang, Polym. Bull., 2007, 59, 269–278 CrossRef CAS
. - H.-J. Hwang, C.-H. Li and C.-S. Wang, Polymer, 2006, 47, 1291–1299 CrossRef CAS PubMed
. - C. H. Lin, S. L. Chang, H. H. Lee, H. C. Chang, K. Y. Hwang, A. P. Tu and W. C. Su, J. Polym. Sci., Part A: Polym. Chem., 2008, 46, 4970–4983 CrossRef CAS PubMed
. - Z.-Y. Wang, J.-C. Ho and W.-J. Shu, J. Appl. Polym. Sci., 2012, 123, 2977–2984 CrossRef CAS PubMed
.
Footnote |
† Electronic supplementary information (ESI) available: 1H, 13C, and 19F NMR, ATR-FTIR, X-ray crystallographic information, and SEM image. CCDC 1054069. For ESI and crystallographic data in CIF or other electronic format see DOI: 10.1039/c5ra13771c |
|
This journal is © The Royal Society of Chemistry 2015 |
Click here to see how this site uses Cookies. View our privacy policy here.