DOI:
10.1039/C5RA17724C
(Paper)
RSC Adv., 2015,
5, 96829-96839
Glycine-assisted evolution of palygorskite via a one-step hydrothermal process to give an efficient adsorbent for capturing Pb(II) ions†
Received
1st September 2015
, Accepted 26th October 2015
First published on 28th October 2015
Abstract
As the materials of “green 21st century material worlds”, natural silicates have received unprecedented attention by virtue of their abundance, low-cost, stability, and non-toxic and eco-friendly nature compared to other synthetic materials. With the aim to develop a new hybrid silicate adsorbent with improved adsorption properties, the naturally abundant palygorskite (PAL) was functionalized with glycine (GLY) via a simple one-step hydrothermal process and used for capturing Pb(II) ions from aqueous solution. The main reaction parameters, e.g., the pH values of the reaction medium, solid-to-liquid ratio, reaction time and dosage of GLY, were systematically optimized, and the as-prepared adsorbent was characterized using X-ray diffraction (XRD), field-emission scanning electron microscopy (FESEM), transmittance electronic microscopy (TEM) and Fourier transform infrared spectroscopy (FTIR) techniques. The results reveal that the PAL crystal was converted to a hybrid silicate material with the assistance of GLY, and simultaneously the functional groups were introduced during the hydrothermal reaction, which caused an evident enhancement in the adsorption capacity of PAL for Pb(II) ions from 55.76 mg g−1 to 123.24 mg g−1. Almost 99.60% of Pb(II) could be captured and removed from a 40 mg L−1 Pb(II) solution using the as-prepared GLY-PAL silicate adsorbent, which is obviously higher than the 83.85% achieved by raw PAL. The intensified complexation of the functional groups on the silicate with Pb(II), the electrostatic attraction and the pore adsorption are responsible for the enhancement in the adsorption capability.
1. Introduction
Palygorskite (PAL, also called attapulgite) is a naturally available hydrated magnesium-rich aluminum silicate clay mineral with a nanorod-like crystal morphology and a nano-channel of 0.37 nm × 0.64 nm along the c-axis of the rod.1 The octahedral cations in the crystalline framework of PAL can be partially replaced by di- or tri-valent cations owing to the isomorphic phenomenon occurring in the formation process of minerals, and so PAL carries permanent negative charges.2 Due to the larger specific surface area and moderate cation exchange capacity, PAL has found widespread applications in many areas, e.g., as a carrier of catalysts,3–5 hybrid pigment,6 slow-release carrier of drugs,7 and adsorbent.8 As a promising adsorbent, PAL has the advantages of being inexpensive, abundant, non-toxic and eco-friendly, and has been widely used to remove toxic heavy metal ions,9,10 organic compounds11,12 or various dyes13–15 from wastewater.
As is known, the adsorption properties of PAL are highly dependent on its multi-porous structure, rich surface charges and surface groups. However, the adsorption capacity of natural PAL is usually limited due to the finite amount of adsorption sites in its structure or on its surface. So, to improve the adsorption properties of natural PAL by building more adsorption sites through a simple but effective method has become a current research hotspot and is also a challenging subject. Thus far, many chemical methods (i.e., acid treatment16,17 and surface organification modifications18,19) and physical methods (i.e., heat treatment,20,21 extrusion,22 and grinding23) have been frequently adopted to improve the adsorption performance of PAL, because these methods may alter the surface charge, number of surface groups and pore structure of PAL. It has also been reported that the introduction of various organic functional groups (i.e., –NH2, –CONH2, –COOH, and –SO32−) onto the surface of PAL are contributory to enhancing the adsorption capacity of PAL for toxic heavy metal ions and dyes.17,24–26 It was demonstrated that the introduced functional groups may intensify the affinity of PAL with heavy metal ions, and thus improve its ability to capture these ions. This has been proved to be a feasible approach to improve the adsorption properties. However, the process of grafting organic functional groups onto the surface of PAL is usually complex, and the grafting efficiency is low. Moreover, the finite adsorption sites on raw PAL itself make it difficult to enhance the adsorption properties to a higher level by only introducing some functional groups. Thus, increasing the adsorption sites by altering the structure of PAL itself and simultaneously introducing functional groups via a one-step reaction process is highly desired as a new feasible approach to enhance the adsorption properties.
Among numerous methods for improving the crystal structure of PAL, the hydrothermal process was intensively studied as a new approach to enhance the performance of materials. Li et al.27 confirm that a montmorillonite supported carbon nanosphere adsorbent (Mt-spC) prepared via a hydrothermal process may evidently enhance the removal efficiency of Cr(VI) from 40% to 80% due to the generation of new adsorption sites. Our previous work also confirmed that the hydrothermal process may evidently enhance the adsorption of methylene blue by PAL by 67.7% in contrast to raw PAL.28 This indicates that the combination of a hydrothermal process with organic molecules containing functional groups will be an feasible approach to fabricate a new adsorbent with improved adsorption properties.
Based on the above background and our previous work,8,28 in this paper, we prepared a hybrid silicate adsorbent via a one-step hydrothermal process of PAL in the presence of non-toxic glycine (GLY) as both the accelerant of the structure evolution of PAL and the “donor” of the functional groups. The reaction parameters were optimized and the PAL-derived adsorbent was characterized using XRD, FESEM, TEM and FTIR techniques. The adsorption properties of the adsorbent towards toxic Pb(II) ions were evaluated, and the adsorption mechanism was also investigated.
2. Experimental
2.1 Materials
Natural PAL clay is taken from Huangnishan Mine located in Xuyi county of Jiangsu Province in China, which was pre-treated by rolling once before use. The chemical composition of the raw PAL clay is SiO2 52.37%, Al2O3 11.67%, Fe2O3 7.91%, MgO 6.92%, CaO 1.91%, K2O 1.49% and Na2O 0.27%, as determined using a MiniPal 4 X-ray fluorescence spectrometer (PANalytical Co., Netherlands). Glycine (NH2CH2COOH, A.R. grade) is purchased from Sinopharm Chemical Reagent Co., Ltd. (Shanghai, China). Pb(CH3COO)2·3H2O (A.R. grade) was provided by Beijing Hongxing Chemical Reagent Factory (Beijng, China). All other reagents are of analytical grade and all solutions are prepared with deionized water.
2.2 Hydrothermal treatment of PAL
The natural PAL was pretreated with 5% HCl aqueous solution at the solid/liquid ratio of 1/10 under stirring for 4 h to remove the associated carbonates, and then the resultant aqueous suspension was passed through a 200-mesh sieve to remove the large grains of quartz. The resultant suspension was centrifuged at 4500 rpm for 10 min to separate the solid from the solution, and the solid product was fully washed with deionized water until the pH value of the supernatant was about 7. Finally, the solid product was oven-dried at 105 °C for 4 h, and then smashed and passed through a 200-mesh screen for further use.
The PAL powder obtained using the above procedure was dispersed in 60 mL of various aqueous solutions of GLY with different concentrations (0.1, 0.5 and 1.0 mol L−1) at different pH values (2, 4, 6, 8, 10, and 12) and different solid/liquid ratios (1/200, 1/100, 1/50, and 1/25). Then, the resultant dispersion was transferred to a 100 mL sealed Teflon reactor, and the reaction was conducted at 180 °C for different lengths of time (12 h, 24 h, 48 h, 60 h, 72 h). After, the reactor was naturally cooled to room temperature, and the solid product was separated by centrifugation at 4500 rpm and fully washed until the pH and conductivity of the supernatant remained constant. Finally, the solid was dried to a constant mass at 60 °C under vacuum, smashed and passed through a 200-mesh sieve for further use.
During the reaction process, the pH value of the solution was adjusted using 1 mol L−1 HCl or 2 mol L−1 NaOH solution. The optimized reaction parameters for preparing the best sample are: GLY dosage, 0.5 mol L−1; pH value, 12; solid/liquid ratio, 1/100 (m/v); reaction time, 60 h. The raw PAL and functionalized PAL are coded as RPAL and GLY(x)-PAL (x represents the concentration of GLY), respectively, and the optimal sample prepared at the optimal condition (GLY dosage, 0.5 mol L−1; pH, 12; solid/liquid ratio, 1/100 (m/v); reaction time, 60 h) was coded as GLY-PAL.
2.3 Batch adsorption experiment
0.0250 g of the adsorbent fully contacted 25 mL of an aqueous solution of Pb(II) ions with the initial concentration of 200 mg L−1 by shaking the solid/liquid mixture on a THZ-98A orbital shaker at a speed of 150 rpm and temperature of 30 °C for 30 min to attain adsorption equilibrium. Afterwards, the solution was separated from the solid adsorbent by centrifugation, and the solution was collected and used to analyze the residual Pb(II) in the solution after adsorption. The concentration of Pb(II) was determined using a Specord 200UV/vis spectrophotometer at the maximum absorbance wavelength of 532 nm using xylenol orange (XO) as the color agent. Then, the amount of Pb(II) ions adsorbed per unit mass of adsorbent could be calculated according to the difference in the Pb(II) concentration in the solution before and after adsorption, using the following equation (eqn (1)): |
 | (1) |
where qe (mg g−1) is the adsorption capacity of the adsorbent for the Pb(II) ions; ci and cf (mg L−1) are the concentrations of the Pb(II) ions in the solution before and after adsorption, respectively; v (L) is the volume of Pb(II) solution used; and w (g) is the mass of the adsorbent used. The RPAL and optimal GLY-PAL samples were used to evaluate the adsorption isotherms and kinetics. Three parallel measurements were carried out and the averages are reported in this paper.
2.4 Characterization
Powder X-ray diffraction (XRD) patterns are collected using an X-ray diffractometer with a Cu anode (PAN analytical Co. X’pert PRO), running at 40 kV and 30 mA. The morphologies of the samples are observed using a field-emission scanning electron microscope (FESEM, JEOL JSM-6701F SEM, Japan). TEM images were taken using a JEM-1200 EX/S transmission electron microscope (TEM, JEOL, Tokyo, Japan). FTIR spectra are measured using a Thermo Nicolet NEXUS TM spectrophotometer over the range of 4000–400 cm−1 using KBr pellets. In order to accurately compare the change in the FTIR absorbance bands of the samples before and after the hydrothermal reaction, the mass of the samples was accurately controlled, with the mass ratio of sample to KBr set at 1
:
150. The mixture of the sample and KBr was fully ground and made into a tablet. The FTIR spectra were normalized by defining the transmittance of the maximum peak as 10%, and defining the transmittance of the baseline as 100%. Zeta potentials are measured using a ZEN3600 Zeta voltmeter (Malvern, Britain). The content of elements C, H and N was detected using an Elementar Analysensysteme GmbH (varioEL cube V1.2.1 2009-1-27, CHN Mode, Ser. No. 19092013).
3. Results and discussion
3.1 FTIR spectra
FTIR spectroscopy is an effective method to analyze the change in chemical groups. Fig. 1 shows the FTIR spectra of GLY and PAL before and after the hydrothermal reaction. As shown in Fig. 1, the characteristic absorbance bands of pure GLY in the crystalline state appear at 1629 cm−1 (δasNH2), 1592 cm−1 (νasCOO−) and 1439 cm−1 (νsCOO−).29–32 The characteristic absorbance bands of PAL appear at 3616 cm−1 (stretching vibration of O–H in (Al)2O–H), 3551 cm−1 (stretching vibration of O–H in Al3+Fe3+(Mg2+)OH) and 1196 cm−1 (asymmetric stretching vibration of the Si–O–Si bond linked two inverse tetrahedron sheet in the ribbon-layer structure of PAL).33,34 After the hydrothermal reaction, the characteristic absorbance bands of PAL show some changes. The bands of RPAL at 3551 cm−1 and 1196 cm−1 disappeared after the reaction when the concentration of the GLY solution is above 0.5 mol L−1, but the two bands only weakened when the concentration of GLY is 0.1 mol L−1. The band at about 980 cm−1 (the perpendicular Si–O asymmetric stretching)35 almost disappeared. These results indicate that some Si–O–Si or Si–O–M bonds may also be broken and the ribbon-layer structure of PAL was destroyed to form a new silicate through the re-arrangement of the PAL crystal.36 The band of RPAL at 3420 cm−1 (the stretching vibration of O–H) shifts to 3441 cm−1 (for GLY(0.5)-PAL) and 3446 cm−1 (for GLY(1)-PAL) after the reaction, due to the interaction between Si–OH and NH2.37 This indicates that the GLY molecules may generate interactions with the bound water molecules coordinated to the (Al, Fe)-octahedra at the edge of the channel. The band at 1654 cm−1, ascribed to the H–O–H bending vibration of the adsorbed, zeolitic and crystalline water molecules, slightly shifts to 1647, 1639 and 1638 cm−1 after the reaction, which may be considered as an effect of the asymmetric vibration of NH2. The above analysis suggests that the hydrothermal reaction has a severe influence on the microscopic structure of the tetrahedral and octahedral sheets.
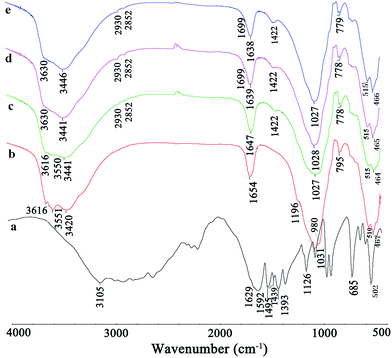 |
| Fig. 1 FTIR spectra of the (a) GLY, (b) RPAL, (c) GLY(0.1)-PAL, (d) GLY(0.5)-PAL and (e) GLY(1)-PAL samples. | |
The new absorbance bands at about 1699 cm−1 (ascribed to the C
O stretching vibration of the –COOH groups),36 at about 1422 cm−1 (the symmetric stretching vibration of –COO−),38,39 and at 2930 and 2852 cm−1 (the C–H asymmetric and symmetric stretching vibration of the –CH2 groups) were observed in the FTIR spectra of the hydrothermally treated PAL. In addition, the asymmetric vibrations of NH2 (1629 cm−1) are not observed owing to the overlap of the H–O–H bending vibration. These results confirm that GLY participates in the hydrothermal reaction and interacts with PAL as the donor of the functional groups (i.e., –NH2 and –COOH groups). The existence of GLY in the GLY-PAL samples was also confirmed by the elemental analysis result. The nitrogen content in RPAL is only 0.06%, but it increases to 0.4% after forming GLY-PAL (Table S1†). Meanwhile, the element mapping of nitrogen also confirmed the existence of nitrogen in GLY-PAL (Fig. S1 and S2†), which is consistent with the FTIR result. According to the result of the element analysis, it can be calculated that the loading amount of GLY in GLY-PAL is 2.15%.
3.2 XRD analysis
Fig. 2 shows the XRD patterns of PAL before and after the hydrothermal reaction. The diffraction peaks at 2θ = 8.31° (110 crystalline plane), 13.6° (200 crystalline plane), 16.4° (130 crystalline plane), 19.8° (040 crystalline plane) and 27.5° (400 crystalline plane) are attributed to the characteristic reflection of the PAL crystal (Fig. 2a).40 Several changes in the reflection peaks could be observed in the XRD patterns of the PAL samples after the hydrothermal reaction: (i) the intensity of the (110) reflection peak of PAL gradually decreased and the position of the peak slightly shifts to a relatively larger angle (2θ = 8.33°); while the (200) and (130) reflections almost disappeared with an increase in the dosage of GLY, indicating that the crystal structure of PAL was partially evolved into an amorphous silicate, and the continuous reverse tetrahedron sheets and discontinuous octahedron sheets in the PAL crystal were deformed;41 (ii) the (040) diffraction peak at 2θ = 19.8° has no obvious changes, indicating that the octahedral structure of PAL was only deformed and has not been severely damaged, which was also confirmed by the FTIR analysis (Fig. 1); (iii) the intensity of the reflection peak of PAL at 2θ = 35.0°, corresponding to the tetrahedra, had almost no change, but the peak becomes sharper after the hydrothermal reaction, which is due to the re-arrangement of the silicon–oxygen tetrahedral chains. Furthermore, the reflection of dolomite at 2θ = 30.9° diminished after the hydrothermal reaction, indicating that the associated dolomite may participate in the hydrothermal reaction.8
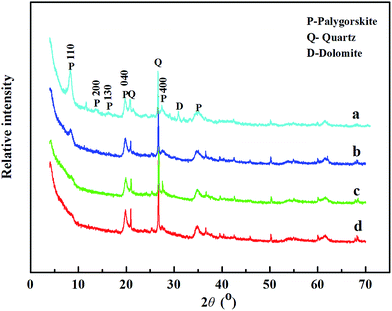 |
| Fig. 2 XRD patterns of the (a) RPAL, (b) GLY(0.1)-PAL, (c) GLY(0.5)-PAL and (d) GLY(1)-PAL samples. | |
3.3 FESEM and TEM morphologies
Fig. 3 shows the FESEM and TEM images of PAL before and after the hydrothermal reaction. As shown in Fig. 3a and a′, lots of bulk crystal bundles and some associated minerals (i.e., quartz and dolomite) could be observed in the FESEM and TEM images of RPAL. After the hydrothermal reaction in 0.1 mol L−1 GLY solution, the dispersity of the PAL rods slightly improved, but the number of PAL rods was reduced and the amount of sheet-like or particle-like matter increased after the hydrothermal process (Fig. 3b and b′). As the concentration of the GLY solution was increased to 0.5 mol L−1, the number of rods was further decreased and more sheet-like matter was produced and associated with the shortened PAL rods to form a rod/sheet mixture (Fig. 3c and c′). With a further increase in the concentration of GLY to 1 mol L−1, the PAL rods become even shorter, and almost thoroughly transform as amorphous silicate (Fig. 3d and d′), as is also confirmed by the XRD result (Fig. 2). The structure of the PAL crystal was almost destroyed. These results indicated that the crystal structure and morphology of PAL evolved during the reaction to form more broken Si–O− bonds as expected by us.42,43 The increase in the GLY dosage may promote the evolution of the PAL crystals. Fig. S5† shows the TEM image of BPAL (prepared at hydrothermal conditions without adding GLY); it was observed that the PAL rods have no obvious change, which is different from the situation in the presence of GLY. This confirms that GLY may contribute to the evolution of the PAL crystals in addition to acting as the donor of functional groups.
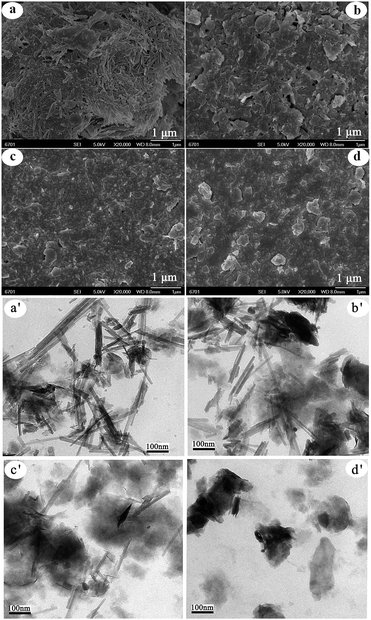 |
| Fig. 3 FESEM (a–d) and TEM (a′–d′) images of the RPAL and GLY-PAL samples: (a and a′) RPAL, (b and b′) GLY(0.1)-PAL, (c and c′) GLY(0.5)-PAL, and (d and d′) GLY(1)-PAL. | |
3.4 Effect of reaction parameters on adsorption capacities
The effect of the reaction parameters, including the pH values, solid/liquid ratio, dosage of GLY and reaction time on the adsorption capacity of the as-prepared adsorbent for Pb(II) ions was investigated, as shown in Fig. 4. As can be seen from Fig. 4a, the pH values of the reaction medium evidently affected the adsorption capacity of the as-prepared adsorbent for Pb(II) ions. Upon increasing the pH value of the reaction medium from 2 to 12, the adsorption capacity sharply increased, and reaches the optimal value at pH 12. The enhanced adsorption of Pb(II) ions by PAL after modification is mainly ascribed to the re-arrangement of the PAL crystal and the change of the surface groups (i.e., –H2N–CH2COO− and Si–O− groups). Fig. S6† shows the TEM image of PAL after the hydrothermal treatment at pH 7 and 9 in the solution of 0.5 mol L−1 GLY. It can be seen that although the GLY dosage is higher, the rod crystals of PAL have not obviously evolved at relatively lower pH values, and lots of rods can still be clearly observed in the TEM image of the product. Compared with that seen in Fig. 3c′, the hydrothermal reaction at pH 12 in the presence of GLY can break more Si–O–Si bonds than Si–O− groups to form a silicate adsorbent rich in Si–O− groups.43 The Si–O− groups, as the important adsorption sites, have a relatively stronger ability to complex to Pb(II) ions, and this is favorable to increase the adsorption capability of the adsorbent to Pb(II). In addition, the GLY may interact with the surface groups of the PAL adsorbent to introduce the functional groups (i.e., –COO−, –NH2). Because the isoelectric point of GLY is 5.97, the GLY molecules may exist in the solution in different forms at different pH values. Under acidic conditions (pH < 5.97), the GLY molecules exist in the protonated form (+H3N–CH2COOH), while the surface of PAL is still negatively charged. The electrostatic interaction between +H3N–CH2COOH and the surface of PAL and the hydrogen-bonding interaction between +H3N–CH2COOH and the Si–OH groups on the surface of PAL results in the weaker bonding of GLY with PAL. Also, the Si–O–Si bond is difficult to be broken at lower pH values (Fig. S6†), and no sufficient Si–O− groups were formed under acidic conditions, which obviously does not contribute to improve the adsorption capacity. Thus, the weaker structure evolution of PAL and the smaller contribution of GLY to the adsorption process lead to the relatively lower adsorption capacity of the PAL-derived product at lower pH values. When the pH > 5.97, the GLY molecules are mainly present in the form of H2N–CH2COO−. Just like the complex interaction of small dye molecules with PAL (i.e., Maya Blue44), the GLY molecules may also coordinate with the metal ions (i.e., Mg2+, Al3+ and Fe3+) in the framework of the PAL-derived product to form a M–H2N–CH2COO− or M–HN–CH2COO− linkage. Under alkaline conditions, the Si–O–M–O–Si bonds are more easily broken, so more O–M–(vacant site) coordination cavities are uncovered, which may accommodate the GLY molecules to form a coordination formulation, which is favorable for the loading of GLY on clay.45–47 Also, the hydrogen-bonding interaction between Si–OH and the GLY molecules are contributory to the immobilization of GLY on the hydrothermally treated PAL product.39,48 The elemental analysis of C, H, and N elements shows that the N content in GLY-PAL is 0.4%, which confirms the existence of GLY molecules in the GLY-PAL product. The breakage of Si–O–Si bonds and the loading of GLY molecules help to improve the adsorption capability of the as-prepared adsorbent for Pb(II) ions. After optimization, pH 12 was selected as the optimal reaction condition.
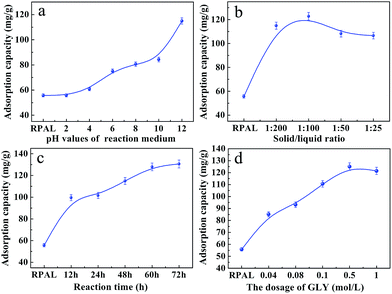 |
| Fig. 4 The effect of (a) the pH values of the reaction medium, (b) the solid–liquid ratio, (c) the reaction time and (d) the GLY dosage on the adsorption capacity for Pb(II). | |
The solid/liquid ratio was optimized at pH 12 and a GLY dosage of 0.5 mol L−1. Fig. 4b shows the effect of the solid/liquid ratio in the reaction system on the adsorption capacities of the silicate adsorbent for Pb(II). As can be seen, by increasing the solid/liquid ratio from 1/200 to 1/25, the adsorption capacities firstly increased, reached the maximum at the solid/liquid ratio of 1/100, and then decreased. Unlike the greater effect of pH value on the adsorption capacity, the solid/liquid ratio has a relatively smaller influence on the adsorption capability. Because the dosage of GLY is fixed, the increase of the solid/liquid ratio means that the amount of GLY corresponding to a unit mass of PAL is less, so the adsorption capacity of the resultant adsorbent decreases. At the solid/liquid ratio of 1/200, the amount of GLY corresponding to a unit mass of PAL is higher, which may seriously damage the crystal structure of PAL, and thus decrease the adsorption capability.
Fig. 4c shows the effect of the reaction time on the adsorption capacities of PAL for Pb(II). It is obvious that prolonging the reaction time has a positive effect on improving the adsorption of the resultant adsorbent for Pb(II). This may be attributed to the evolution of the crystal structure and the full reaction of GLY with PAL made possible by prolonging the reaction time. A reaction time of 72 h corresponds to the optimum adsorption capability. As an overall consideration, we selected 60 h as the final reaction time.
The effect of the GLY dosage on the adsorption capacity was optimized at the optimal pH value and solid/liquid ratio and with a reaction time of 60 h. As shown in Fig. 4d, the adsorption capacity of the PAL adsorbent for Pb(II) gradually increases with the increase in the dosage of GLY, reaching the maximum at a dosage of 0.5 mol L−1 and then slightly decreasing with a further increase in the dosage of GLY, indicating that a moderate concentration is the key to obtaining the optimal adsorbent. So, 0.5 mol L−1 is selected as the optimum concentration. As shown in the XRD, SEM and TEM analyses, the dosage of GLY may greatly affect the evolution degree of the PAL crystal. At lower dosages of GLY (0.1 mol L−1), the rod crystal structure only slightly evolved, and only a small portion of the Si–O–Si (or M) bonds were broken to form the Si–O− groups that help to improve the adsorption. By increasing the dosage of GLY to 0.5 mol L−1, many more Si–O–Si(M) bonds were broken and the PAL crystal evolved into an amorphous silicate adsorbent rich in Si–O− groups, as also confirmed by the increase of the negative zeta potential, which enhances the adsorption capability. At a higher dosage of GLY (1 mol L−1), the crystal structure of PAL was almost thoroughly destroyed, which is not favorable for adsorption. As shown in Fig. S7,† GLY-PAL shows a relatively higher adsorption capacity than the blank sample (BPAL), which confirms the positive effect of GLY in improving the adsorption. Thus, the optimal GLY dosage is selected as 0.5 mol L−1.
3.5 Effect of external pH on adsorption capacities
It is known that the pH value during the adsorption process may affect the adsorption behavior of an adsorbent,49 because it could alter the surface charge and existence state of the adsorption sites of an adsorbent. It was reported that the Pb(II) species exist in the forms Pb2+, Pb(OH)+, Pb(OH)20 and Pb(OH)3− in solutions at different pH values.25 At pH < 6.5, the predominant lead species are Pb2+ ions and the removal of Pb(II) is mainly ascribed to the adsorption of Pb2+. At pH > 6.5, Pb(II) will be precipitated. The effect of the pH value on the adsorption of Pb(II) was investigated over a pH range from 1 to 6.5 (Fig. 5a). As can be seen, the adsorption capacity (qe) evidently increased after increasing the pH value from 1 to 6.5, and a dramatic increase of adsorption capacity occurred with pH 4 as the turning point, indicating that the removal of Pb(II) by the optimal GLY-PAL sample is highly dependent on the external pH value. In contrast, the adsorption capacities of BPAL (prepared at pH 12, solid/liquid ratio of 1/100, reaction time of 60 h, without GLY) and RPAL also increased with an increase in the pH value, which further indicated that the external pH values have an obvious effect on adsorption. At each pH value, the adsorption capability of GLY-PAL is always higher than that of RPAL and BPAL, which confirms that GLY is essential to improve the adsorption capability of PAL.
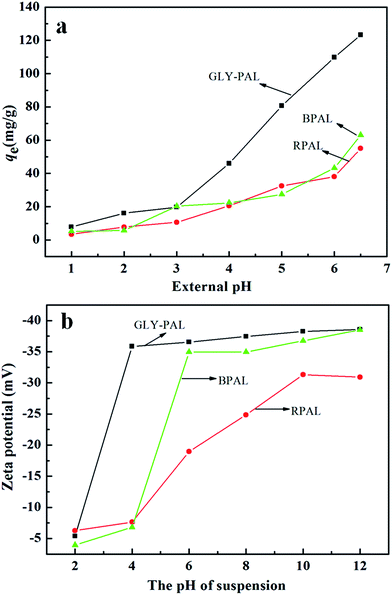 |
| Fig. 5 The effect of the external pH on (a) the adsorption capacity, and (b) the zeta potential. | |
In fact, the change in the external pH value mainly affects the surface charges and existence form of the surface groups. To explore the relationship between the external pH value and the adsorption capacities, the zeta potentials of the optimal GLY-PAL sample were determined over a range of pH values from 2 to 12. As is shown in Fig. 5b, the zeta potential is negative over the whole studied pH range, indicating that RPAL, BPAL and GLY-PAL all have an electronegative surface. The negative zeta potential of GLY-PAL is lower (−5.39 mV) at pH 2, but it sharply increased to −35.85 mV at pH 4. With a further increase in the pH value, the zeta potential increases further, which is in accordance with the increasing tendency of the adsorption capacity (pH 4 is the turning point). The increased negative zeta potential is due to the breakage of the Si–O–Si(M) bonds, the formation of more Si (or Al)–O− bonds and the loading of GLY containing functional groups onto the GLY-PAL sample. That is to say, the increase in the zeta potential improved the electrostatic interactions in GLY-PAL and the complexing capability of Si–O− to Pb(II). Similarly, the zeta potentials become more negative for BPAL and RPAL with increasing pH values, as shown in Fig. 5b, but the increasing tendency of the adsorption capability is not as apparent as that of GLY-PAL. This further indicates that the functional groups of GLY, besides the Si–O− groups and the electrostatic attraction, may contribute to the adsorption process.37 At a relatively higher pH, the Si–O and –COOH (or –NH2) groups tend to exist in the form Si–O− and –COO− (–NH2), respectively, which have a relatively stronger complexing capabilities towards Pb(II), which is helpful to enhance the adsorption capability.
3.6 Effect of contact time on adsorption and adsorption kinetics
The optimal GLY-PAL sample was selected to investigate the adsorption kinetics. As shown in Fig. 6a, the adsorption capacity increases with the contact time. About 54.3% of Pb(II) was removed within the initial 5 min, and then the increasing trend becomes flat until the adsorption equilibrium was achieved at a contact time of 30 min. The decrease in the adsorption rate with longer contact times is mainly because the adsorption sites on the surface of the adsorbents were rapidly saturated with increased contact times, which leads to a decrease in the concentration gradient (the main driving force for the diffusion of adsorbate) between the solution and the solid/liquid interface.50 For RPAL, a contact time of 60 min is needed to achieve the adsorption equilibrium, while 30 min is enough for the GLY-PAL adsorbent, indicating that the GLY-PAL adsorbent has a relatively rapid adsorption rate compared to raw PAL. Furthermore, in order to study the adsorption kinetics of the GLY-PAL adsorbent for Pb(II), the most commonly used kinetic models, including the pseudo-first-order (eqn (2))51 and pseudo-second-order (eqn (3))52 kinetic models were used to fit and analyze the kinetic adsorption process. |
ln(qe − qt) = ln q1e − k1t
| (2) |
|
 | (3) |
where the parameters k1 (min−1) and k2 (g (mg−1 min−1)) are the rate constants calculated from the pseudo-first-order and pseudo-second-order kinetic models, respectively. qt and qe (mg g−1) are the amounts of Pb(II) ions adsorbed per unit mass of adsorbent at time t and at equilibrium, respectively. As can be seen from Fig. 6b, the linear correlation coefficients obtained by fitting with the pseudo-first-order kinetic model for RPAL and GLY-PAL are very low, whereas the linear correlation coefficient obtained from the fitting of the pseudo-second-order kinetic model is very high (>0.9999) and the plots are also well fitted as straight lines over the entire range of the contact time. Also, the theoretical adsorption capacity (q2e) calculated using the pseudo-second-order kinetic model is almost equivalent to the experimental value, but the q1e obtained from the pseudo-first-order kinetic model is far away from the experimental value (see Table 1). Therefore, the adsorption of Pb(II) onto the RPAL and GLY-PAL adsorbents conformed to the pseudo-second-order kinetic model.
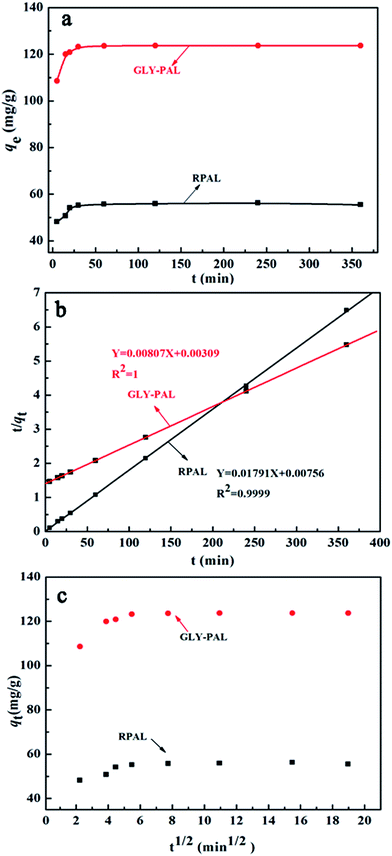 |
| Fig. 6 (a) Adsorption kinetic curves of the RPAL and GLY-PAL adsorbents for Pb(II), (b) fitting curves of t/qt versus t, and (c) plot of the Weber–Morris equation for the adsorption of Pb(II). | |
Table 1 Adsorption kinetic parameters for the adsorption of Pb(II) onto RPAL and GLY-PAL
Samples |
qexp (mg g−1) |
Pseudo-first-order kinetic model |
Pseudo-second-order kinetic model |
Inter-particle kinetic model |
q1e (mg g−1) |
k1 (min−1) |
R2 |
q2e (mg g−1) |
k2 (g mg−1 min−1) |
R2 |
kint (mg g−1 min−1/2) |
R2 |
RPAL |
55.76 |
2.17 |
0.0045 |
0.2285 |
55.87 |
0.042 |
0.9999 |
0.3273 |
0.4469 |
GLY-PAL |
123.24 |
2.66 |
0.0227 |
0.6510 |
123.46 |
0.021 |
1 |
0.5106 |
0.3469 |
As discussed previously, the pseudo-second-order kinetic model represents an associated chemisorption and surface diffusion process. The good fitting of the experimental data with the pseudo-second-order kinetic model indicates that the adsorption of Pb(II) onto the GLY-PAL adsorbent is mainly dominated by chemisorption.52,53 The intra-particle diffusion/transportation process was often used to describe a rate controlling step of the metal ions in many adsorption processes. The possibility of intra-particle diffusion was checked using the Weber–Morris intra-particle diffusion model, which is presented in eqn (4):54
where
kint is the intra-particle diffusion rate constant (mg g
−1 min
−1/2), and
qt is the amount of Pb(
II) adsorbed onto the adsorbent at any time
t. A plot of
qt versus t1/2 for Pb(
II) is displayed in
Fig. 6c, and the corresponding kinetic parameters calculated from the intra-particle diffusion model are listed in
Table 1. The two distinct stages were evidently observed in
Fig. 6c, which is not linear over the whole time range. The first portion corresponds to the external surface adsorption of Pb(
II) onto the surface of GLY-PAL, which is due to boundary layer diffusion effects. And the second portion indicates a gradual equilibrium adsorption due to intra-particle diffusion.
55 The larger slope suggests that Pb(
II) quickly migrates from the bulk solution to the surface of the adsorbent in the first portion. The gentle slope for the second portion indicates that intra-particle diffusion plays a predominant role in the adsorption process. The contribution of the intra-particle diffusion effect is lower than that of the surface diffusion, but it is assumed to be the rate-controlling step.
56
3.7 Effect of the initial concentration on adsorption and adsorption isotherms
The effect of different initial concentrations (10.0–200 mg L−1) of Pb(II) on the adsorption properties of RPAL and GLY-PAL was investigated and is shown in Fig. 7. The adsorption capacities of RPAL and GLY-PAL for Pb(II) initially rapidly increased as the initial concentration was increased, and then the increasing tendency became slow. This is because the concentration gradient increases with an increasing initial concentration, which provides the vital driving force to promote the interaction between the Pb(II) and adsorbents, and then enhances the adsorption capability of the adsorbent to Pb(II).
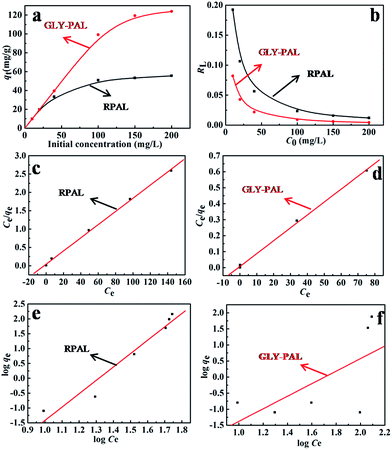 |
| Fig. 7 Effect of initial concentration on the adsorption for Pb(II) (a); the relationship between the initial concentration and RL (b); the fitting curves with the Langmuir isotherm model (c and d) and the Freundlich isotherm model (e and f). | |
In order to further study the adsorption behaviors and explore the adsorption mechanism, the adsorption isotherm was fitted with the classical Langmuir and Freundlich isotherm models, which could demonstrate how the adsorbate interacts with the adsorbent in the process of reaching the adsorption equilibrium. In these two models, the Langmuir isotherm model considers that only monolayer adsorption occurs on a surface, assuming that all the adsorption sites are the same on the adsorbent. The linear form of the Langmuir equation is presented as follows:57
|
 | (5) |
where
ce (mg L
−1) is the concentration of Pb(
II) in the solution after the adsorption equilibrium,
qm is the saturated adsorption capacity (mg g
−1) theoretically calculated by fitting the curves with the Langmuir model, and
qe (mg g
−1) is the adsorption capacity of Pb(
II) in the equilibrium state.
b (L mg
−1) is a constant which is bound up with the energy of adsorption.
qm and
b can be calculated from the linear plots of
ce/
qe versus ce.
A dimensionless equilibrium factor RL for the Langmuir isotherm can predict the characteristic of a sorption system.58 The parameter is defined as:
|
 | (6) |
where
b is the constant mentioned above in the Langmuir isotherm equation.
c0 is the initial concentration of Pb(
II) solution. The value of
RL can be calculated using the above equation and the plot of
RL vs. c0 is given in
Fig. 7b. The fact that 0 <
RL < 1 suggests a favorable adsorption process.
The Freundlich isotherm is used to describe the distribution of adsorbate and sorbent species in the adsorption system. The equation of the Freundlich isotherm model is expressed as:57
|
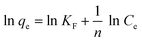 | (7) |
where
KF ((mg g
−1) (L mg
−1)) is the Freundlich isotherm constant with respect to the binding energy and is defined as the distribution coefficient. The constant
n usually refers to the adsorption intensity. The
KF and
n values can be calculated from the slope and intercept of the linear plots of ln
qe versus ln
Ce, respectively.
The experimental data was fitted by the Langmuir and Freundlich isotherm models, and the results are shown in Fig. 7(c and d) and (e and f), respectively. The relevant parameters were calculated by the fitting the results with the Langmuir isotherm and Freundlich isotherm models (Fig. 7(c and d) and (e and f)), and the results are listed in Table 2. As can be seen, the curves obtained by fitting the experimental data with the Langmuir isotherm model shows better linear correlation coefficients above 0.9986, and the experimental adsorption capacities (qe) are almost equal to the theoretically calculated values. Comparatively, the linear correlation coefficient obtained from the Freundlich model is smaller than that obtained from the Langmuir model. This confirms that the Langmuir model is more suitable to describe the adsorption process, and the adsorption of Pb(II) onto GLY-PAL tends to be a monolayer coverage process. Moreover, the value of RL is in the range of 0–0.2 for RPAL and is smaller than 0.1 for GLY-PAL (Fig. 7b), which indicates that the adsorption process of Pb(II) onto GLY-PAL is spontaneous and favorable. In our work, the adsorption capacity of GLY-PAL for Pb(II) is 123.24 mg g−1, but it is only 55.76 mg g−1 when raw PAL is used when the initial concentration of the Pb(II) solution is 200 mg L−1. At each initial concentration, the adsorption capacity of GLY-PAL is much higher than that of RPAL. Compared with the other adsorbents reported previously (as listed in Table 2), the GLY-PAL adsorbent shows a relatively better adsorption capability, and has potential to be used as an efficient adsorbent for capturing Pb(II) Table 3.
Table 2 Adsorption isotherm parameters for the adsorption of Pb(II) onto RPAL and GLY-PAL
Samples |
Langmuir model |
Freundlich model |
qm (mg g−1) |
b (L mg−1) |
R2 |
n |
KF |
R2 |
RPAL |
55.56 |
0.42 |
0.9986 |
4.74 |
21.11 |
0.9495 |
GLY-PAL |
123.46 |
1.12 |
0.9988 |
4.65 |
48.53 |
0.4203 |
Table 3 Comparison of the adsorption capacities of Pb(II) ions onto various adsorbents
Adsorbent materials |
qm (mg g−1) |
References |
GLY-PAL |
123.24 |
This work |
Palygorskite |
62.11 |
59 |
Natural Jordanian clay |
66.2 |
60 |
Kaolinite clay |
19.27 |
61 |
Clay/poly(methoxyethyl)acrylamide–tripolyphosphate-impregnated |
81.02 |
62 |
Sodium tetraborate-modified kaolinite clay |
56.18 |
63 |
MX-80 bentonite |
68.52 |
64 |
The removal efficiency of GLY-PAL for Pb(II) is satisfactory. At a lower initial concentration (<20 mg L−1), the percentage removal of Pb(II) by RPAL and GLY-PAL can reach a climax of 98.80% and 99.60%, respectively. However, with a further increase in the initial concentration to 40 mg L−1, the percentage removal of Pb(II) by RPAL is only 83.85%, but it can reach 99.60% for GLY-PAL. Even when the initial concentration is 200 mg L−1, the percentage removal of Pb(II) by GLY-PAL can reach 61.9%; while the removal ratio of RPAL is only 27.7%. This reveals that GLY-PAL can be used for the highly efficient removal of Pb(II) from aqueous solution. In comparison with the efficient adsorption of Pb(II) by the GLY-PAL adsorbent, the adsorption capabilities of the adsorbent for Cu(II) (27.56 mg g−1) and Zn(II) (27.63 mg g−1) are relatively lower (Fig. S8†), which indicate that the GLY-PAL adsorbent has a relatively stronger combination ability with Pb(II) compared to that with Cu(II) and Zn(II).
3.8 Adsorption mechanisms
As for the adsorption process of metal ions onto the adsorbent, the electrostatic attraction, pore adsorption, ion-exchange and chemical complexation are generally considered as the main driving forces to immobilize the metal ions on the solid phase.65
The FTIR spectra of GLY-PAL and GLY-PAL-Pb (GLY-PAL after the adsorption of Pb(II)) show that the C
O stretching vibration of the –COOH and –COO− groups at 1699 cm−1 slightly shift to 1705 cm−1 after the adsorption of Pb(II). Meanwhile, the band at 1422 cm−1 shifts to 1437 cm−1 (Fig. 8). The band at about 1639 cm−1 (H–O–H bending mode) shifts to 1660 cm−1 after the adsorption. Moreover, the absorbance peak at about 3440 cm−1 seemingly becomes sharper after the adsorption of Pb(II), which is attributed to the stretching vibration of O–H (weak-broad) and N–H (variable). This further suggests that oxygen atoms in the hydroxyl groups could also participate in the adsorption of Pb(II).39 All of the above indicate that lots of –COOH (–COO−) and Si–O− groups can complex with Pb(II) during the adsorption process.
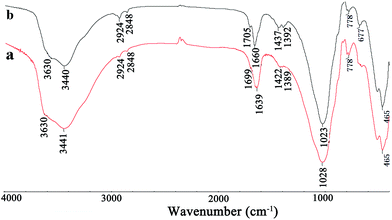 |
| Fig. 8 FTIR spectra of (a) GLY-PAL and (b) GLY-PAL-Pb. | |
As shown in Table S2 (see ESI†), the BET specific surface area of GLY-PAL decreased after adsorption because the part of the pores that is used for the adsorption was blocked after adsorbing Pb(II), which confirms that pore adsorption plays a role in the adsorption of Pb(II). Fig. S1, S2, S9 and S10 (see ESI†) show the EDS spectra and elemental mapping of the GLY-PAL and GLY-PAL-Pb samples. It is obvious that no Pb element was observed in the EDS spectrum and element mapping of GLY-PAL, but a clear and uniform distribution of Pb element was observed in the elemental mapping of GLY-PAL-Pb, with a strong peak of Pb in the EDS spectrum. This confirms that Pb(II) was adsorbed onto the GLY-PAL adsorbents.
In addition, the zeta potentials of GLY-PAL at different pH values (Fig. 5) confirm that the change in the adsorption capacity is consistent with the change in the negative zeta potential, which implies that the electrostatic attraction is contributory to the adsorption, but it is not the main factor. In order to confirm the action of GLY on improving the adsorption, a control experiment without adding GLY was conducted and the result is shown in Fig. S7 (see ESI†). As can be seen, the adsorption capacity is only 63.06 mg g−1 for the BPAL sample, which is slightly higher than that of RPAL, indicating that the GLY-assisted structural evolution via the hydrothermal process and the loading of GLY may contribute to the improvement of the adsorption capacity. For one thing, GLY molecules promote the evolution of the PAL crystal (as confirmed by XRD, TEM and SEM analyses); for another, the existence of GLY in GLY-PAL may facilitate the adsorption of Pb(II). Combined with the above detailed discussion, the dominating adsorption mechanisms for the Pb(II) ions can be proposed: (i) the pores existing in GLY-PAL give rise to pore adsorption; (ii) the electrostatic attraction leads to the Pb(II) in the solution being more easily transferred to the surface of the GLY-PAL adsorbent; the Si(or M)–O− groups on the surface of GLY-PAL (Surf-O−) could also chelate with Pb(II) and hold it on the surface of GLY-PAL according to the equation: 2Surf-O− + Pb2+ → 2Surf-O−⋯Pb2+;66 (iii) the breakage of the Si–O–M–O–Si framework in the PAL crystal under higher pH conditions may generate many vacant coordination sites around the Al, Mg or Fe ions, which may coordinate with NH2–CH2–COO−/NH–CH2–COO− (under alkaline conditions) to form M–NH2–CH2–COO− or M–OOC–CH2–NH2 linkages, and the functional groups were introduced. The existence of N in GLY-PAL can be directly confirmed by the element mapping of the nitrogen element as shown in Fig. S2.† The introduced –NH2 or –COO− groups are one of the efficient functional groups used to remove heavy metal ions.67 Therefore, Pb(II) ions may be bound with the functional groups on GLY-PAL through a coordination interaction,68,69 as shown in eqn (8).
|
2GLY-PAL–COO− (or –NH2) + Pb(II) → 2GLY-PAL–COO−⋯Pb(II) (or 2GLY-PAL–NH2⋯Pb(II))
| (8) |
4. Conclusions
Inspired by the “from nature, for nature and into nature” idea, the earth-abundant, inexpensive and eco-friendly natural PAL clay was used as the raw material to fabricate a new silicate adsorbent with the assistance of GLY via a facile one-step hydrothermal process. The influence of the primary parameters (pH values, reaction time, solid-to-liquid ratio and the GLY dosage) on the structure and adsorption properties of PAL was discussed in detail. The optimal reaction parameters were obtained as: pH, 12; solid/liquid ratio, 1/100; reaction time, 60 h; and GLY dosage, 0.5 mol L−1. It was revealed that the inert Si–O–Si and Si–O–M bonds can be easily broken under alkaline conditions to form Si–O− groups, and the PAL crystal was evolved into a new amorphous silicate with an evident increase in the negative zeta potential and an enhancement of the surface complexation capability. Without adding GLY, the hydrothermal process has little contribution to the structure evolution and the improvement of the adsorption capacity, but the introduction of GLY can promote the structure evolution of PAL and bring functional groups onto the adsorbent. The Si–O–M–O–Si framework could be broken under alkaline conditions, which provides cavities O–M–(vacant site) that may accommodate the coordination of GLY molecules and then immobilize them. The functional groups were introduced onto the GLY-PAL adsorbent. By comparison with raw PAL, the as-prepared GLY-PAL adsorbent displays superior adsorption performance, with a maximum adsorption capacity of 123.24 mg g−1 (compared to only 55.76 mg g−1 for RPAL) and a higher percentage removal of 99.6%. The adsorption process for Pb(II) mainly involves pore adsorption, electrostatic attraction and chelation. As a whole, the low-cost adsorbent derived from natural PAL clay exhibits outstanding adsorption properties for Pb(II), eco-friendly advantages, and can be used as a promising adsorbent candidate for future environmental applications.
Acknowledgements
The authors would like to thank National Natural Science Foundation of China (NO. 21377135 and 51403221) and “863” Project of the Ministry of Science and Technology, P. R. China (No. 2013AA032003) for the financial support of this research.
References
- W. F. Bradley, Am. Mineral., 1940, 25, 405 CAS.
- E. Galán, Clay Miner., 1996, 31, 443 Search PubMed.
- W. B. Wang, F. F. Wang, Y. R. Kang and A. Q. Wang, RSC Adv., 2013, 3, 11515 RSC.
- D. Papoulis, S. Komarneni, D. Panagiotaras, P. Tsolis-Katagas, D. Panagiotaras, H. G. Kacandes, P. Zhang, S. Yin, T. Sato and H. Katsuki, Appl. Clay Sci., 2013, 83, 191 CrossRef.
- R. Liu, X. Z. Niu, X. N. Xia, Z. B. Zeng, G. Z. Zhang and Y. B. Lu, RSC Adv., 2015, 5, 62394 RSC.
- A. Doménech-Carbó, F. M. Valle-Algarra, M. T. Doménech-Carbó, L. Osete-Cortina and M. E. Domine, RSC Adv., 2013, 3, 20099 RSC.
- Y. Kong, H. Ge, J. Xiong, S. Zuo, Y. Wei, C. Yao and L. Deng, Appl. Clay Sci., 2014, 99, 119 CrossRef CAS.
- H. L. Lu, H. Xu, Y. Chen, J. L. Zhang and J. X. Zhuang, RSC Adv., 2014, 4, 5873 RSC.
- W. B. Wang, G. Y. Tian, Z. F. Zhang and A. Q. Wang, Chem. Eng. J., 2015, 265, 228 CrossRef CAS.
- S. S. Gupta and K. G. Bhattacharyya, RSC Adv., 2014, 4, 28537 RSC.
- N. Frini-Srasra and E. Srasra, Surf. Eng. Appl. Electrochem., 2009, 45, 306 CrossRef.
- X. G. Wang, S. Y. Lü, C. M. Gao, X. B. Xu, X. J. Zhang, X. Bai, M. Z. Liu and L. Wu, Chem. Eng. J., 2014, 252, 404 CrossRef CAS.
- W. B. Wang, F. F. Wang, Y. R. Kang and A. Q. Wang, Water, Air, Soil Pollut., 2015, 226, 83 CrossRef.
- A. S. Bhatt, P. L. Sakaria, M. Vasudevan, R. R. Pawar, N. Sudheesh, H. C. Bajaj and H. M. Mody, RSC Adv., 2012, 2, 8663 RSC.
- R. Giustetto and O. Wahyudi, Microporous Mesoporous Mater., 2011, 142, 221 CrossRef CAS.
- H. Chen, Y. G. Zhao and A. Q. Wang, J. Hazard. Mater., 2007, 149, 346 CrossRef CAS PubMed.
- N. Frini-Srasra and E. Srasra, Desalination, 2010, 250, 26 CrossRef CAS.
- R. L. Frost and E. Mendelovici, J. Colloid Interface Sci., 2006, 294, 47 CrossRef CAS PubMed.
- L. X. Zhang, Q. Z. Jin, J. H. Huang, Y. F. Liu, L. Shan and X. G. Wang, Appl. Surf. Sci., 2010, 256, 5911 CrossRef CAS.
- T. H. Chen, J. Wang, C. S. Qin, S. C. Peng, Y. X. Song and Y. Guo, J. Chin. Ceram. Soc., 2006, 34, 1406 CAS.
- D. H. Su, C. H. Wang, S. M. Cai, C. D. Mu, D. F. Li and W. Lin, Appl. Clay Sci., 2012, 41, 62 Search PubMed.
- J. X. Xu, W. B. Wang and A. Q. Wang, Appl. Clay Sci., 2014, 95, 365 CrossRef CAS.
- Y. Liu, W. B. Wang and A. Q. Wang, Powder Technol., 2012, 225, 124 CrossRef CAS.
- P. Deepika, K. S. Avijeet and J. S. Michael, Colloid Polym. Sci., 2013, 291, 1795 Search PubMed.
- G. Z. Kyzas and N. K. Lazaridis, J. Colloid Interface Sci., 2009, 331, 32 CrossRef CAS PubMed.
- E. K. Yetimoglu, M. V. Kahraman and O. Ercan, React. Funct. Polym., 2007, 67, 451 CrossRef CAS.
- T. Li, J. F. Shen, S. T. Huang, N. Li and M. X. Ye, Appl. Clay Sci., 2014, 93–94, 48 CrossRef CAS.
- Z. F. Zhang, W. B. Wang and A. Q. Wang, Appl. Surf. Sci., 2015, 329, 306 CrossRef CAS.
- M. Meng, L. Stievano and J. F. Lambert, Langmuir, 2004, 20, 914 CrossRef CAS PubMed.
- A. Ihs, B. Liedberg, K. Uvdal, C. TÖrnkvist, P. BodÖ and I. LundstrÖm, J. Colloid Interface Sci., 1990, 140, 192 CrossRef CAS.
- I. Laulicht, S. Pinchas, D. Samuel and I. Wasserman, J. Phys. Chem., 1966, 70, 2719 CrossRef CAS PubMed.
- K. Uvdal, P. BodÖ, A. His, B. Liedberg and W. R. Salaneck, J. Colloid Interface Sci., 1990, 140, 207 CrossRef CAS.
- W. C. Yan, D. Liu, D. Y. Tan, P. Yuan and M. Chen, Spectrochim. Acta, Part A, 2012, 97, 1052 CrossRef CAS PubMed.
- R. L. Frost, O. B. Locos, H. Ruan and J. T. Kloprogge, Vib. Spectrosc., 2001, 27, 1 CrossRef CAS.
- D. A. McKeown, J. E. Post and E. S. Etz, Clays Clay Miner., 2002, 50, 667 CrossRef CAS.
- Q. F. Liu, X. Yao, H. F. Cheng and R. L. Frost, Spectrochim. Acta, Part A, 2012, 96, 784 CrossRef CAS PubMed.
- X. H. Wang and A. Q. Wang, Desalin. Water Treat., 2012, 48, 38 CrossRef CAS.
- J. Z. Guo, B. Li, L. Liu and K. Lv, Chemosphere, 2014, 111, 225 CrossRef CAS PubMed.
- M. Elena Ramos and F. Javier Huertas, Appl. Clay Sci., 2013, 80–81, 10 CrossRef.
- J. E. Chisholm, Can. Mineral., 1990, 28, 329 CAS.
- R. L. Frost and Z. Ding, Thermochim. Acta, 2003, 397, 119 CrossRef CAS.
- N. Güven and L. L. Carney, Clays Clay Miner., 1979, 27, 253 Search PubMed.
- D. C. Golden and J. B. Dixon, Clays Clay Miner., 1990, 38, 401 CAS.
- R. Giustetto, K. Seenivasan, D. Pellerej, G. Ricchiardi and S. Bordiga, Microporous Mesoporous Mater., 2012, 155, 167 CrossRef CAS.
- S. Kalra, C. K. Pant, H. D. Pathak and M. S. Mehta, Indian J. Biochem. Biophys., 2000, 37, 341 CAS.
- S. Kalra, C. K. Pant, H. D. Pathak and M. S. Mehata, Colloids Surf., A, 2003, 212, 43 CrossRef CAS.
- W. Bodenheimer and L. Heller, Clay Miner., 1967, 7, 167 CAS.
- S. Shoval and S. Yariv, Clays Clay Miner., 1979, 27, 19 CAS.
- M. Sathishkumar, A. R. Binupriya, D. Kavitha, R. Selvakumar, R. Jayabalan, J. G. Choi and S. E. Yun, Chem. Eng. J., 2009, 147, 265 CrossRef CAS.
- M. Ravanan, M. Ghaedi, A. Ansari, F. Taghizadeh and D. Elhamifar, Spectrochim. Acta, Part A, 2014, 123, 467 CrossRef CAS PubMed.
- Y. S. Ho, Scientometrics, 2004, 59, 171 CrossRef CAS.
- Y. S. Ho and G. McKay, Water Res., 2000, 34, 735 CrossRef CAS.
- X. Jing, F. Liu, X. Yang, P. Ling, L. Li, C. Long and A. Li, J. Hazard. Mater., 2009, 167, 589 CrossRef CAS PubMed.
- W. J. Weber and J. C. Morris, J. Sanit. Eng. Div., Am. Soc. Civ. Eng., 1963, 89, 31 Search PubMed.
- G. Crank, The mathematics of diffusion, Clarendon Press, London, 1933 Search PubMed.
- Y. S. Ho and G. Mckay, Process Biochem., 2003, 38, 1047 CrossRef CAS.
- M. Mohapatra, S. Khatun and S. Anand, Chem. Eng. J., 2009, 155, 184 CrossRef CAS.
- E. Malkoc, J. Hazard. Mater., 2006, 137, 899 CrossRef CAS PubMed.
- J. H. Potgieter, S. S. Potgieter-Vermaak and P. D. Kalibantonga, Miner. Eng., 2006, 19, 463 CrossRef CAS.
- Y. S. Al-Degs, M. I. El-Barghouthi, A. A. Issa, M. A. Khraisheh and G. M. Walker, Water Res., 2006, 40, 2645 CrossRef CAS PubMed.
- K. O. Adebowale, I. E. Unuabonah and B. I. Olu-Owolabi, J. Hazard. Mater., 2006, 134, 130 CrossRef CAS PubMed.
- M. Sölener, S. Tunali, A. S. Özcan, A. Özcan and T. Gedikbey, Desalination, 2008, 223, 308 CrossRef.
- E. I. Unuabonah, K. O. Adebowale, B. I. Olu-Owolabi, L. Z. Yang and L. X. Kong, Hydrometallurgy, 2008, 93, 1 CrossRef CAS.
- D. Xu, X. L. Tan, C. L. Chen and X. K. Wang, Appl. Clay Sci., 2008, 41, 37 CrossRef CAS.
- D. L. Sparks, in Environmental Soil Chemistry, ed. D. L. Sparks, USA, 2003 Search PubMed.
- H. Chen and A. Q. Wang, J. Colloid Interface Sci., 2007, 307, 309 CrossRef CAS PubMed.
- H. Wang, J. Kang, H. Liu and J. Qu, J. Environ. Sci., 2009, 21, 1473 CrossRef CAS.
- E. Sabah and M. S. Celik, J. Colloid Interface Sci., 2002, 251, 33 CrossRef CAS PubMed.
- W. X. Kuang, G. A. Facey, C. Detellier, B. Casal, J. M. Serratosa and E. Ruiz-Hitzky, Chem. Mater., 2003, 15, 4956 CrossRef CAS.
Footnote |
† Electronic supplementary information (ESI) available. See DOI: 10.1039/c5ra17724c |
|
This journal is © The Royal Society of Chemistry 2015 |
Click here to see how this site uses Cookies. View our privacy policy here.