DOI:
10.1039/C4SC02269F
(Edge Article)
Chem. Sci., 2015,
6, 666-674
Laser-induced pinpoint hydrogen evolution from benzene and water using metal free single-walled carbon nanotubes with high quantum yields†
Received
30th July 2014
, Accepted 9th September 2014
First published on 9th September 2014
Introduction
Carbon nanomaterials have been widely studied for their potential applications as electrode materials for efficient energy conversion and storage.1–5 Metal-free nanostructured elemental carbons and carbon-based composites have proven to be attractive alternatives to conventional metal-based catalysts for several important chemical reactions such as dehydrogenation reactions of aromatic compounds,5–7 oxygenation8 and Friedel–Crafts reactions.9,10 However, these reactions were carried out under severe conditions to activate substrate molecules.
In particular, single-walled carbon nanotubes (SWCNTs) have been of great interest to researchers because of their unique structures and physical properties.11–17 SWCNTs have been proposed as advanced metal-catalyst supports for electrochemical catalysis.18–20 However, there are no reports that SWCNTs alone are used as photocatalysts under ambient conditions due to their poor photochemical and excited properties.
Catalytic hydrogen (H2) evolution systems have been extensively studied because hydrogen is a clean energy source for the future, which should reduce dependence on fossil fuels and emissions of greenhouse gases in the long term.21,22 In many cases, noble metals such as platinum and semiconductors have been used as photocatalysts.23–35 However, there are no reports on photocatalytic metal-free H2 evolution systems using pure carbon alone as a photocatalyst.36
We report herein efficient H2 evolution from benzene and benzene derivatives using metal free SWCNTs alone as a photocatalyst under visible laser light irradiation (532 nm) at room temperature and atmospheric pressure with a high quantum yield of 130%. Efficient laser-induced hydrogen evolution was also observed from water with SWCNTs. The reaction mechanisms of laser-induced H2 evolution from benzene and water with SWCNTs are clarified based on the oxidized products, deuterium kinetic isotope effects and the dependence of the rate of H2 evolution on the laser intensity. This is the first example of laser-induced H2 evolution with high quantum yields, paving a new way for pinpoint H2 production using a laser pulse, which may find various applications.
Results and discussion
Laser-induced photocatalytic hydrogen evolution from benzene with SWCNTs
Laser pulse irradiation (λ = 532 nm; 500 mW; 10 Hz) of a deaerated benzene solution (2.5 mL) containing dispersed metal-free SWCNTs (0.15 mg) resulted in efficient hydrogen evolution. The amount of hydrogen evolved by laser irradiation for 2 h reached 100 μmol (2.2 mL). The quantum yield of hydrogen evolution was determined from the initial rate to be 34%. When benzene was replaced by deuterated benzene (C6D6), the deuterated hydrogen molecules such as D2 and HD were also evolved efficiently (see Fig. S1 in the ESI†). The initial rate of hydrogen evolution was 21 μmol h−1 with a 50
:
1 ratio for D2 and HD, respectively. The KIE (kinetic isotope effect) value was determined from the slopes in Fig. 1 to be 2.4. These results indicate that the hydrogen source is benzene. The products derived from the dehydrogenation of benzene were determined by GC-MS and HPLC analyses to be biphenyl, terphenyls, and terphenylene (see Fig. S2 and S3 in ESI†). The stoichiometry required to produce H2 and biphenyl from benzene is given by eqn (1). |  | (1) |
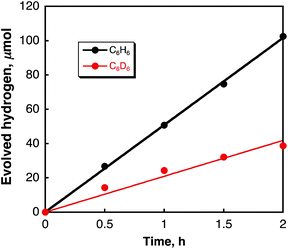 |
| Fig. 1 Time courses of hydrogen evolution in deaerated C6H6 (black circles) and C6D6 (red circles) containing SWCNTs (0.060 mg mL−1) under laser irradiation at 532 nm (50 mJ per pulse). | |
There is no evidence for the functionalisation of SWCNTs by benzene under photoirradiation, which was observed by TG analyses (Fig. 2) because no weight loss from the decomposition of functionalized molecules to SWCNTs was observed at low temperature. Thus, hydrogen was evolved via the condensation reaction of benzene in the photocatalytic reaction.
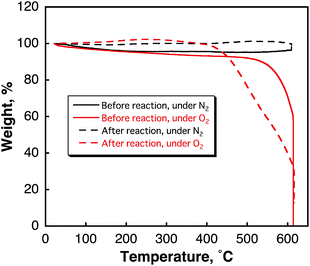 |
| Fig. 2 TG curves of SWCNTs before and after laser light irradiation (H2 evolution) in benzene observed under deaerated and aerated conditions. | |
Hydrogen was also evolved in various aromatic solvents with electron withdrawing and donating substituent(s) (Fig. S4 in ESI†). The amounts of H2 evolved are listed in Table 1. The highest catalytic activity was obtained in benzene. The catalytic turnover number (TON) is roughly estimated as (2.0 ± 0.5) × 106 per SWCNT for 2 h irradiation, calculated from the tube diameter and average length of the SWCNTs with a zig–zag structure used in this study.37 When benzene was replaced by benzene-d6, the TON decreased to (8.0 ± 2.0) × 105 per SWCNT. This value agrees with the KIE of 2.4 determined from the initial rate of H2 evolution as shown in Fig. 1.
Table 1 Amount of H2 evolved in various solvents after laser irradiation for 2 h
Solvent |
H2 evolveda μmol |
Conditions: SWCNTs (0.15 mg) dispersed in deaerated solvent (2.5 mL). Excited at 532 nm (50 mJ per pulse).
|
Benzene |
100 |
Mesitylene |
71 |
Toluene |
66 |
p-Xylene |
60 |
Chlorobenzene |
54 |
Benzonitrile |
39 |
1,2-Dimethoxybenzene |
12 |
Transmission electron microscopy (TEM) measurements were performed to evaluate the transformation of SWCNTs before and after hydrogen evolution. The TEM images before photoirradiation (Fig. 3a and b) clearly exhibit tubular morphologies. There are no inorganic impurities in the commercially available and highly purified SWCNTs used in this study (see Experimental section). After hydrogen evolution, the tubular structure was partially changed to an agnail structure and small clusters. However, a tubular component still remains (Fig. 3c and d).
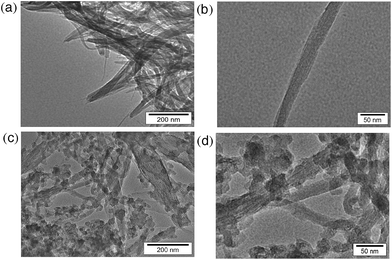 |
| Fig. 3 TEM images of SWCNTs (a and b) before and (c and d) after laser photoirradiation (50 mJ per pulse, 10 Hz) for 2 h in deaerated benzene at 298 K. | |
The dependence of the rate of H2 evolution on the laser intensity was examined using different laser power intensities at 532 nm (0–82 mJ per pulse). The initial rates of H2 evolution are proportional to the fourth power of the laser intensity as shown in Fig. 4. This suggests that a bimolecular reaction of two-photon absorbed species may be involved in the photocatalytic hydrogen evolution.
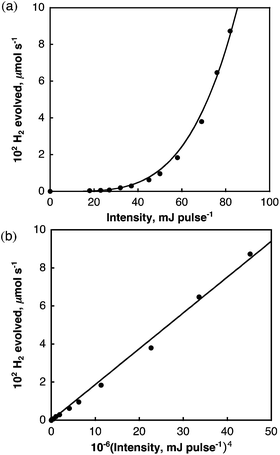 |
| Fig. 4 (a) Plot of initial rate of H2 evolved in deaerated benzene vs. laser power intensity at 532 nm; (b) plot of initial rate of H2 evolved vs. the fourth power of the laser intensity. | |
On the basis of the above-mentioned results, the plausible photocatalytic mechanism for hydrogen evolution in benzene is shown in Scheme 1. A SWCNT was excited by two photons to form a doubly excited SWCNT (SWCNT**). The SWCNT** can reduce benzene to produce the benzene radical anion (C6H6˙−) and the one-electron oxidized SWCNT (SWCNT˙+) with the rate constant ket1. Dimerisation of C6H6˙− occurs, accompanied by hydrogen evolution to produce the biphenyl dianion [(C6H5)22−] with the rate constant kH. Such hydrogen evolution via the radical coupling of C6H6˙− has previously been reported for the reduction of benzene with a cesium nano carbon catalyst.38 (C6H5)22− can reduce benzene to produce C6H6˙− and the biphenyl radical anion [(C6H5)2˙−] in benzene.39 Back electron transfer from (C6H5)2˙− to SWCNT˙+ results in the formation of biphenyl [(C6H5)2], accompanied by the regeneration of the SWCNT. On the other hand, C6H6˙− can reduce the SWCNT to produce SWCNT˙− and C6H6 with the rate constant ket2. The charge recombination from SWCNT˙− to SWCNT˙+ also regenerates the SWCNT. The overall stoichiometry of the photocatalytic cycle in Scheme 1 agrees with eqn (1).
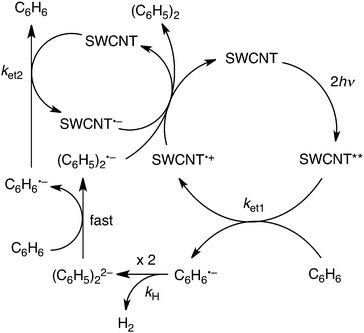 |
| Scheme 1 | |
According to Scheme 1, the rate of hydrogen evolution is given by eqn (2). The rate of formation and decay of C6H6˙− is given by eqn (3).
| d[H2]/dt = kH[C6H6˙−]2 | (2) |
| d[C6H6˙−]/dt = ket1[SWCNT**] − kH[C6H6˙−]2 − ket2[SWCNT][C6H6˙−] | (3) |
Assuming that
ket2[SWCNT][C
6H
6˙
−] ≫
kH[C
6H
6˙
−]
2, the steady-state concentration of C
6H
6˙
− is given by
eqn (4).
| [C6H6˙−] = ket1[SWCNT**]/(ket2[SWCNT]) | (4) |
From
eqn (2) and
(4), the rate of hydrogen evolution is rewritten by
eqn (5).
| d[H2]/dt = kH [ket1[SWCNT**]/(ket2[SWCNT])]2 | (5) |
Because the concentration of SWCNT** is proportional to the square of the laser intensity, the rate of hydrogen evolution is proportional to the fourth power of the laser intensity as observed in
Fig. 4b. The observed deuterium kinetic isotope effect on the photocatalytic H
2 evolution in
Fig. 1 suggests that the C–H bond cleavage of C
6H
6˙
− (
kH) is involved as the rate-determining step in the radical coupling for H
2 evolution in
Scheme 1.
In Scheme 1, C6H6˙− produced by the electron transfer from C6H6 to SWCNT** dimerizes to afford H2 and (C6H5)22−, followed by rapid electron transfer from C6H6 to (C6H5)22− to yield C6H6˙− and (C6H5)2˙−. The overall reaction of C6H6˙− with C6H6 to yield H2 and (C6H5)2˙− is shown in Scheme 2, where (C6H5)2˙− can react further with C6H6 to produce the terphenyl radical anion [(C6H5(C6H4)C6H5)˙−] and H2. The same type of reaction continues and the overall reaction is given by eqn (6). Thus, once one mol of C6H6˙− is produced, n moles of hydrogen can be produced from n moles of benzene to form the radical anion of a benzene polymer [(C6H5(C6H4)n−1C6H5)˙−], which may undergo charge recombination with SWCNT˙+ to regenerate the SWCNT.
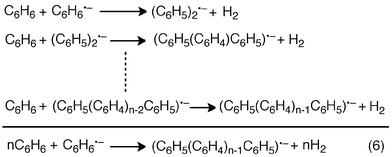 |
| Scheme 2 | |
According to Scheme 1, biphenyl is produced by the four-photon process when the maximum value of the quantum yield is 25%. However, the polymerisation of benzene induced by photo-generated C6H6˙− in Scheme 2 gains a leverage effect to increase the quantum yield of H2 evolution above that expected from the four-photon process. Indeed, the highest quantum yield of H2 evolution was determined to be 130% at a laser power of 82 mJ per pulse under the conditions in Fig. 2a, where the photon number of the laser pulse was calibrated by ferrioxalate actinometry (see the experimental section in ESI†).40
The efficiency of the photocatalytic H2 evolution is affected by substitution of the benzene ring with electron donating or withdrawing substituents (Table 1). The efficiency of photocatalytic H2 evolution is highest for benzene and lowest for 1,2-dimethoxybenzene, because substitution on the benzene ring may retard the radical coupling with hydrogen and the electron donating substituents (methoxy group) may slow down electron transfer to the SWCNT** in Scheme 1.
Radical intermediates involved in the photocatalytic H2 evolution from benzene with SWCNTs were detected by ESR spectroscopy measured at 77 K as shown in Fig. 5. The observed ESR signal in the region of g = 2.0025 can be assigned to radical anions derived from benzene,41 which are overwrapped with SWCNT˙+.42 The intensity of the ESR signal increased with increasing photoirradiation time because the stability of radical anions is expected to increase as the polymerisation of benzene in Scheme 2 proceeds.
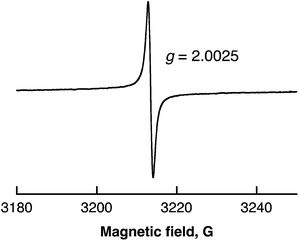 |
| Fig. 5 ESR spectrum of benzene containing SWCNTs (0.12 mg mL−1) after laser pulse irradiation at 532 nm (40 mJ per pulse; 10 Hz) for 10 min at 77 K. | |
Laser-induced hydrogen evolution from water with SWCNTs
Hydrogen evolution also occurred in deaerated H2O (2.5 mL) containing dispersed metal-free SWCNTs (2.0 mg) under Nd–YAG laser pulse irradiation (λ = 532 nm; 600 mW; 10 Hz) as shown in Fig. 6. The amount of evolved H2 after 5 h reached 16.4 μmol, which is 2.7 × 104 times larger than the amount of SWCNT (0.6 nmol) calculated from the tube diameter and average length of SWCNTs with a zig–zag structure used in this study. When H2O was replaced by D2O, the deuterated hydrogen molecules such as D2 and HD were also evolved (see Fig. S5 in ESI†) and the KIE value was determined from the ratio of the H2 evolution in H2O vs. D2O (Fig. 7) to be 1.9, which is somewhat smaller than the value in benzene. These results indicate that the hydrogen source of evolved H2 is water.
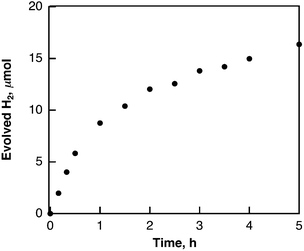 |
| Fig. 6 Time course of hydrogen evolution in deaerated H2O containing SWCNTs (0.80 mg mL−1) under laser irradiation at 532 nm (60 mJ per pulse; 10 Hz). | |
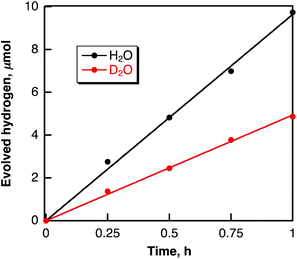 |
| Fig. 7 Time courses of H2 and D2 evolution in deaerated H2O (black circles) and D2O (red circles), respectively, containing SWCNTs (0.80 mg mL−1) under laser irradiation at 532 nm (60 mJ per pulse; 10 Hz). | |
In contrast to the case of benzene, no oxidized form of water (dioxygen or hydrogen peroxide) was produced after H2 evolution (see Fig. S3 in ESI†). In such a case, SWCNTs may be oxidized, accompanied by the laser-induced H2 evolution. Comparison of the TEM images of SWCNTs before and after laser photoirradiation in H2O (Fig. 8) indicates that the tubular morphology remained after the H2 evolution by laser photoirradiation. Comparison of the Raman spectra of SWCNTs before and after laser photoirradiation of SWCNTs dispersed in deaerated H2O are shown in Fig. 9. The G-bands at 1590 and 1570 cm−1 decreased with the appearance of the D-band at 1340 cm−1. The increased D/G ratio observed after the photoirradiation suggests that sidewall functionalisation of SWCNTs occurred.43,44 The IR spectra also suggest that SWCNTs were hydroxylated to exhibit O–H stretching vibrations at 3200–3600 cm−1 (Fig. S6 in ESI†).
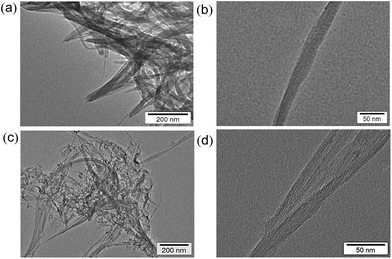 |
| Fig. 8 TEM images of SWCNTs (a, b) before and (c, d) after laser photoirradiation (60 mJ per pulse, 10 Hz) for 2 h in deaerated H2O at 298 K. | |
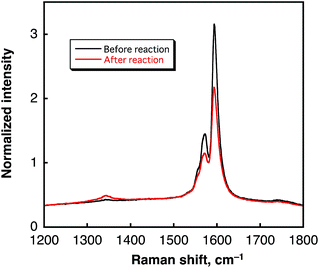 |
| Fig. 9 Raman spectra of SWCNTs before laser irradiation (black line) and (b) SWCNTs obtained by laser light irradiation (λ = 532 nm; 60 mJ per pulse; 10 Hz) for 5 h in deaerated water (0.80 mg mL−1, red line). | |
Comparison of the weight loss of SWCNTs before and after the laser irradiation in H2O observed in the TG measurements (Fig. S7 in ESI†) also suggests that SWCNTs were hydroxylated. The number of OH groups of one SWCNT is estimated from the elemental analyses of SWCNTs before (C 96.81% and H 0.19%)45 and after evolution of 16.4 μmol of H2 (C 91.88% and H 0.60%) to be 18
000. Thus, the H2 evolution is accompanied by the two-electron oxidation of a SWCNT (attachment of two OH groups). The stoichiometry of the laser-induced H2 evolution with SWCNTs in H2O is given by eqn (7).
|  | (7) |
The laser-induced H2 evolution rate with SWCNTs in H2O increases with the increasing amount of SWCNTs to reach a constant value as shown in Fig. 10. This shows sharp contrast with the case of the laser-induced H2 evolution with SWCNTs in benzene, when the rate of H2 evolution was independent of the amount of SWCNTs as discussed above based on Scheme 1. The pH dependence of the H2 evolution rate was also examined as shown in Fig. 11, where the rate of H2 evolution is rather independent of pH.
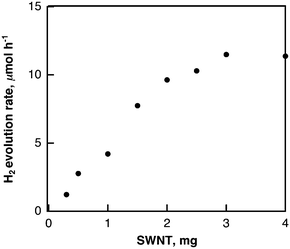 |
| Fig. 10 Plot of rate of H2 evolution in deaerated H2O (2.5 mL) containing various amounts of SWCNTs under laser irradiation at 532 nm (60 mJ per pulse; 10 Hz) vs. amount of SWCNTs. | |
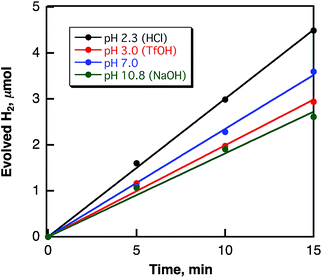 |
| Fig. 11 Time courses of H2 evolution in deaerated H2O containing various amounts of SWCNTs (0.80 mg mL−1) under laser irradiation at 532 nm (60 mJ per pulse; 10 Hz) at various pHs. | |
The overall stoichiometry agrees with that in eqn (7). The EPR spectrum exhibits a radical intermediate in the H2 evolution with SWCNTs from water as shown in Fig. 12. The EPR signal was clearly observed at g = 2.0030 under laser irradiation at 77 K in frozen water containing SWCNTs. The g value is larger than the signal at g = 2.0025 observed in benzene (Fig. 5). Such a large g value indicates the existence of SWCNT(OH)˙ as a radical intermediate due to the spin–orbit coupling of oxygen in the laser-induced H2 evolution with SWCNTs in water.
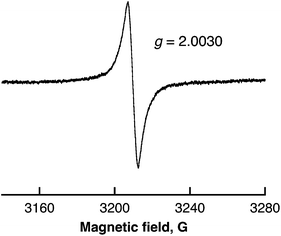 |
| Fig. 12 ESR spectrum of frozen water containing SWCNTs (0.80 mg mL−1) after laser pulse irradiation at 532 nm (60 mJ per pulse; 10 Hz) for 10 min at 77 K. | |
As was the case for the laser-induced H2 evolution in benzene, the initial rates of laser-induced H2 evolution in H2O are proportional to the fourth power of laser intensity as shown in Fig. 13. This suggests that the doubly excited SWCNT (SWCNT**) and the subsequent bimolecular reaction are involved in the H2 evolution. In the case of H2O, a solvated electron (e−(H2O)) may be produced by the reaction of SWCNT** with H2O as shown in Scheme 3, where the bimolecular reaction of e−(H2O) produces H2 and two equiv. of OH− as indicated by the pulse radiolysis study of H2O.46–49 The OH− may be attached to a SWCNT to produce SWCNT(OH)−, which is oxidized by the hole of SWCNT˙+ to afford SWCNT(OH)˙, which may disproportionate to yield the dihydroxylated SWCNT [SWCNT(OH)2], accompanied by the regeneration of the SWCNT.
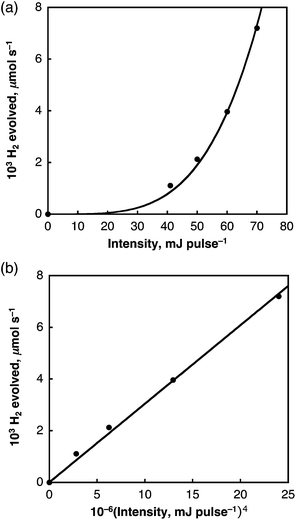 |
| Fig. 13 (a) Plot of initial rate of H2 evolved in H2O vs. laser power intensity at 532 nm; (b) plot of initial rate of H2 evolved vs. the fourth power of laser intensity. | |
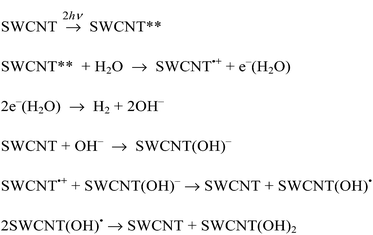 |
| Scheme 3 | |
Conclusions
In conclusion, SWCNTs have been demonstrated to act as efficient photocatalysts for H2 evolution from benzene derivatives under laser irradiation. The TON was over 1 million based on one nanotube. The rate of H2 evolution increased with increasing the laser intensity, exhibiting a fourth power dependence, because hydrogen was evolved via the radical coupling of radical anions derived from benzene as the rate-determining step and a benzene radical anion was produced by electron transfer from the doubly excited state of a SWCNT to benzene, which requires two photons. The polymerisation of benzene induced by photogenerated C6H6˙− accompanied by H2 evolution gains a leverage effect to increase the quantum yield of H2 evolution to as high as 130%, which is much larger than that expected from the four-photon process (25%). Laser-induced H2 evolution also occurred with SWCNTs in H2O, also exhibiting a fourth power dependence for the H2 evolution rate. In this case, H2 was evolved via the electron-transfer reduction of water by the doubly excited state of a SWCNT, and a SWCNT was oxidized to yield the dihydroxylated SWCNT. Metal-free laser-induced H2 evolution in aromatic compounds and H2O with SWCNTs found in this study paves a new way for efficient pinpoint hydrogen evolution, which may find various applications.
Experimental section
Materials
Chemicals were purchased from a commercial source and used without purification. SWCNTs (synthetic method: arc plasma jet, diameter: 1.4 nm approximate length: 1–5 μm, percentage of carbon: >99%) were obtained from Meijo Nano Carbon, Japan. The solution dispersed SWCNTs were prepared by ultrasonication (42 kHz, 125 W) for 5 min. Benzene was of spectral grade, obtained commercially and used without further purification. Deuterated benzene (C6D6, 99%) was obtained from Cambridge Isotope Laboratories, Inc., and was used as received. Benzonitrile was distilled over P2O5in vacuo.50 Mesitylene, p-xylene, chlorobenzene and 1,4-dimethoxybenzene were obtained commercially and used as received. Potassium ferrioxalate used as an actinometer was prepared according to the literature and purified by recrystallisation from hot water.39 D2 gas (99.5%) was commercially obtained from Sumitomo Seika Chemicals Co., Ltd. Purification of water (18.2 MΩ cm) was performed with a Milli-Q system (Millipore, Direct-Q 3 UV).
Reaction procedure
The photocatalytic hydrogen evolution was carried out by the following procedure. C6H6, C6D6 or benzene derivative solutions (2.5 cm3) containing SWCNTs (0.15 mg) in a square quartz cuvette (10 mm i.d.) sealed with a rubber septum was deaerated by bubbling with nitrogen through a stainless steel needle for 5 min. The solution was then irradiated with a Nd:YAG laser (LS2134UTF) at λ = 532 nm with the power of 50 mJ per pulse at room temperature. The gas in the headspace was analyzed using a Shimadzu GC-14B gas chromatograph (detector, TCD; column temperature, 50 °C; column, active carbon with 60–80 mesh particle size; carrier gas, N2) to quantify the evolved hydrogen. The reaction solution was analyzed by a Shimadzu GC-17A gas chromatograph and Shimadzu MS-QP5000 mass spectrometer to quantify the produced biphenyl, and HPLC [detector, UV at λ = 280 nm (SPD-10A, Shimadzu); column, Shim Pack VP-ODS; eluent, CH3CN: 0.40 mL min−1, water: 0.10 mL min−1] to qualify the generated terphenyls. Hydrogen evolved in C6D6 after 2 h laser irradiation was detected using a Shimadzu GC-8A gas chromatograph [detector, TCD; column temperature, 77 K (liquid N2); column, Hydro Isopack (2.0 m, 4.0 mm i.d., GTR TEC Co., Ltd.); carrier gas, Ne] to analyze H2, HD and D2 gases. In the case of measuring the laser intensity dependence, a benzene solution (2.5 cm3) containing SWCNTs (0.15 mg) in a square quartz cuvette (10 mm i.d.) sealed with a rubber septum was deaerated by bubbling with nitrogen through a stainless steel needle for 5 min. The solution was then irradiated using a Nd:YAG laser (LS2134UTF) at λ = 532 nm with the various laser intensities (18–82 mJ per pulse) at room temperature. The amount of hydrogen evolved was analyzed at 10, 20 and 30 min using a Shimadzu GC-14B gas chromatograph.
Characterisation of SWCNTs as catalysts
Transmission electron microscopy (TEM) images of SWCNTs before and after 2 h laser irradiation (λ = 532 nm; 50 mJ per pulse; 10 Hz) in a benzene solution were measured using a JEOL JEM 2100 operating at 200 kV. TG data were recorded on a SII TG/DTA 7200 instrument. SWCNTs, (∼1 mg) before and after the reaction , were heated from 25 °C to 600 °C with a ramp rate of 2 °C min−1. A certain amount of α-Al2O3 was used as a reference for DTA measurements. Raman spectra were obtained using a JASCO NR-1800 with a 514.5 nm Ar laser. IR spectra were recorded on a JASCO FT/IR-6200, using KBr pellets.
Quantum yield determinations
A standard actinometer (potassium ferrioxalate)39 was used for the quantum yield determination of hydrogen evolution from benzene with SWCNTs. A square quartz cuvette (10 mm i.d.) containing a benzene solution (2.5 cm3) of SWCNTs (0.15 mg) was irradiated using a Nd:YAG laser (LS2134UTF) at λ = 532 nm with the various laser intensities (18–82 mJ per pulse). Under the conditions of actinometry experiments, SWCNTs absorbed essentially 100% of the incident light of λ = 532 nm. The light intensity of the laser light of λ = 532 nm was determined as 6.5 × 10−9 einstein s−1 at 8.0 mJ per pulse. The photochemical reaction was monitored using a Shimadzu GC-14B gas chromatograph. The quantum yields were determined from the amount of hydrogen evolved.
EPR measurements
The EPR spectra were measured on a JEOL X-band EPR spectrometer (JES-ME-LX) using a quartz EPR tube containing SWCNTs (24 μg) dispersed in deaerated benzene (0.20 cm3) by laser irradiation (λ = 532 nm; 40 mJ per pulse; 10 Hz) for 10 min at 77 K. The internal diameter of the EPR tube is 4.5 mm, which is small enough to fill the EPR cavity but large enough to obtain good signal-to-noise ratios during the EPR measurements. The amplitude of modulation was chosen to optimize the resolution and the signal-to-noise (S/N) ratio of the observed spectra. The g values were calibrated with an Mn2+ marker.
Acknowledgements
This work was supported by Grants-in-Aid (nos 26620154 and 26288037 to K.O. and nos 24350069 and 25600025 to Y.Y.) from the Ministry of Education, Culture, Sports, Science and Technology (MEXT) and an ALCA project from JST, Japan (to S.F.). We acknowledge Research Centre for Ultra-Precision Science & Technology in Osaka University for TEM measurements.
Notes and references
-
(a) L. Dai, Acc. Chem. Res., 2013, 46, 31 CrossRef CAS PubMed;
(b) L. Dai, D. W. Chang, J.-B. Baek and W. Lu, Small, 2012, 8, 1130 CrossRef CAS PubMed;
(c) T. Chen and L. Dai, Mater. Today, 2013, 16, 272 CrossRef CAS PubMed.
-
(a) P. V. Kamat and G. C. Schatz, J. Phys. Chem. C, 2009, 113, 15437 CrossRef;
(b) P. V. Kamat, J. Phys. Chem. Lett., 2011, 2, 242 CrossRef CAS.
- U. Sahaym and N. M. Grant, J. Mater. Sci., 2008, 43, 5395 CrossRef CAS.
-
(a) A. Arico, Nat. Mater., 2005, 4, 366 CrossRef CAS PubMed;
(b) D. R. Rolison, J. W. Long, J. C. Lytle, A. E. Fischer, C. P. Rhodes, T. M. McEvoy, A. M. Bourg and M. E. Lubers, Chem. Soc. Rev., 2009, 38, 226 RSC.
-
(a) M. Z. Jacobson, Energy Environ. Sci., 2009, 2, 148 RSC;
(b) R. M. Dell and D. A. J. Rand, J. Power Sources, 2001, 100, 2 CrossRef CAS.
- N. Keller, N. I. Maksimova, V. V. Roddatis, M. Schur, G. Mestl, Y. V. Butenko, V. L. Kuznetsov and R. Schlögl, Angew. Chem., Int. Ed., 2002, 41, 1885 CrossRef CAS.
- J. Zhang, D. Su, A. Zhang, D. Wang, R. Schlögl and C. Hébert, Angew. Chem., Int. Ed., 2007, 46, 7319 CrossRef CAS PubMed.
-
(a) N. Zhang, Y. Zhang and Y.-J. Xu, Nanoscale, 2012, 4, 5792 RSC;
(b) N. Zhang, Y. Zhang, M.-Q. Yang, Z.-R. Tang and Y.-J. Xu, J. Catal., 2013, 299, 210 CrossRef CAS PubMed;
(c) Y. Zhang, Z.-R. Tang, X. Fu and Y.-J. Xu, ACS Nano, 2011, 5, 7426 CrossRef CAS PubMed.
- F. Goettmann, A. Fischer, M. Antonietti and A. Thomas, Angew. Chem., Int. Ed., 2006, 45, 4467 CrossRef CAS PubMed.
- J. J. Díaz, L. M. C. Suárez and J. L. Figueiredo, Appl. Catal., A, 2006, 311, 51 CrossRef PubMed.
-
(a) S. Iijima and T. Ichihashi, Nature, 1993, 363, 603 CrossRef CAS;
(b) K. Hata, D. N. Futaba, K. Mizuno, T. Namai, M. Yumura and S. Iijima, Science, 2004, 306, 1362 CrossRef CAS PubMed.
-
(a) Y. Chen and J. Zhang, Acc. Chem. Res., 2014, 47, 2273 CrossRef CAS PubMed;
(b) M. F. L. De Volder, S. H. Tawfick, R. H. Baughman and A. J. Hart, Science, 2013, 339, 535 CrossRef CAS PubMed;
(c) L. Kavan and L. Dunsch, ChemPhysChem, 2011, 12, 47 CrossRef CAS PubMed.
- D. M. Guldi, G. M. A. Rahman, F. Zerbetto and M. Prato, Acc. Chem. Res., 2005, 38, 871 CrossRef CAS PubMed.
- A. C. Dillon, K. M. Jones, T. A. Bekkedahl, C. H. Kiang, D. S. Bethune and M. J. Heben, Nature, 1997, 386, 377 CrossRef CAS.
-
(a) C. N. R. Rao and A. Govindaraj, Acc. Chem. Res., 2002, 35, 998 CrossRef CAS PubMed;
(b) C. N. R. Rao and A. Govindaraj, RSC Nanosci. Nanotechnol., 2011, 18, 1 CAS;
(c) C. J. Shearer, A. Cherevan and D. Eder, Adv. Mater., 2014, 26, 2295 CrossRef CAS PubMed;
(d) Y. Lin, J. Ren and X. Qu, Acc. Chem. Res., 2014, 47, 1097 CrossRef CAS PubMed.
-
(a) B. Mu, J. Zhang, T. P. McNicholas, N. F. Reuel, S. Kruss and M. S. Strano, Acc. Chem. Res., 2014, 47, 979 CrossRef CAS PubMed;
(b) D. A. Heller, H. Jin, B. M. Martinez, D. Patel, B. M. Miller, T.-K. Yeung, P. V. Jena, C. Hoebartner, T. Ha, S. K. Silverman and M. S. Strano, Nat. Nanotechnol., 2009, 4, 114 CrossRef CAS PubMed.
-
(a) Y. Miyauchi, J. Mater. Chem. C, 2013, 1, 6499 RSC;
(b) S. Schafer, N. M. B. Cogan and T. D. Krauss, Nano Lett., 2014, 14, 3138 CrossRef CAS PubMed.
-
(a) J. Gao, J. Zhong, L. Bai, J. Liu, G. Zhao and X. Sun, Sci. Rep., 2014, 4, 3606 Search PubMed;
(b) M. Lefévre, E. Proietti, F. Jaouen and J. Dodelet, Science, 2009, 324, 71 CrossRef PubMed;
(c) D. H. Lee, W. J. Lee, S. O. Kim and Y. H. Kim, Phys. Rev. Lett., 2011, 106, 175502 CrossRef.
- D. R. Rolision, Science, 2003, 299, 1698 CrossRef PubMed.
-
(a) T. Iwasita, Electrochim. Acta, 2002, 47, 3663 CrossRef CAS;
(b) E. A. Batista, G. R. P. Malpass, A. J. Motheo and T. Iwasita, Electrochem. Commun., 2003, 5, 843 CrossRef CAS PubMed.
-
A. Züttel, A. Borgschulte and L. Schlapbach, Hydrogen as a Future Energy Carrier, Wiley-VCH, Weinheim, Germany, 2008 Search PubMed.
- S. Fukuzumi, Eur. J. Inorg. Chem., 2008, 1351 CrossRef CAS.
-
(a) Q. Xiang, J. Yu and M. Jaroniec, J. Am. Chem. Soc., 2012, 134, 6575 CrossRef CAS PubMed;
(b) Q. Xiang, J. Yu and M. Jaroniec, Nanoscale, 2011, 3, 3670 RSC;
(c) Q. Xiang, J. Yu and M. Jaroniec, J. Phys. Chem. C, 2011, 115, 7355 CrossRef CAS.
- A. Iwase, Y. H. Ng, Y. Ishiguro, A. Kudo and R. Amal, J. Am. Chem. Soc., 2011, 133, 11054 CrossRef CAS PubMed.
- Q. Li, B. Guo, J. Yu, J. Ran, B. Zhang, H. Yan and J. R. Gong, J. Am. Chem. Soc., 2011, 133, 10878 CrossRef CAS PubMed.
-
(a) W. Fan, Q. Lai, Q. Zhang and Y. Wang, J. Phys. Chem. C, 2011, 115, 10694 CrossRef CAS;
(b) A. Mukherji, B. Seger, G. Q. Lu and L. Wang, ACS Nano, 2011, 5, 3483–3492 CrossRef CAS PubMed.
-
(a) M. G. Walter, E. L. Warren, J. R. McKone, S. W. Boettcher, Q. Mi, E. A. Santori and N. S. Lewis, Chem. Rev., 2010, 110, 6446 CrossRef CAS PubMed;
(b) X. Chen, S. Shen, L. Guo and S. S. Mao, Chem. Rev., 2010, 110, 6503 CrossRef CAS PubMed.
-
(a) K. Maeda and K. Domen, J. Phys. Chem. Lett., 2010, 1, 2655 CrossRef CAS;
(b) K. Maeda, ACS Catal., 2013, 3, 1486 CrossRef CAS;
(c) A. Kudo and Y. Miseki, Chem. Soc. Rev., 2009, 38, 253 RSC.
-
(a) J.-M. Lehn and J.-P. Sauvage, Nouv. J. Chim., 1977, 1, 449 CAS;
(b) K. Kalyanasundaram, Coord. Chem. Rev., 1982, 46, 159 CrossRef CAS;
(c) J. R. Darwent, P. Douglas, A. Harriman, G. Porter and M.-C. Richoux, Coord. Chem. Rev., 1982, 44, 83 CrossRef CAS;
(d) J. Kiwi and M. Grätzel, J. Am. Chem. Soc., 1979, 101, 7214 CrossRef CAS;
(e) M. Grätzel, Acc. Chem. Res., 1981, 14, 376 CrossRef.
-
(a) D.-L. Jiang, C.-K. Choi, K. Honda, W.-S. Li, T. Yuzawa and T. Aida, J. Am. Chem. Soc., 2004, 126, 12084 CrossRef CAS PubMed;
(b) Y. Amao, Y. Tomonou and I. Okura, Sol. Energy Mater. Sol. Cells, 2003, 79, 103 CrossRef CAS.
-
(a) M. S. Oskoui, M. Khatamian, M. Haghighi and A. Yavari, RSC Adv., 2014, 4, 19569 RSC;
(b) F. Chen, J. Zai, M. Xu and X. Qian, J. Mater. Chem. A, 2013, 1, 4316 RSC;
(c) F. Dai, J. Zai, R. Yi, M. L. Gordin, H. Sohn, S. Chen and D. Wang, Nat. Commun., 2014, 5, 4605 CrossRef PubMed.
-
(a) J. Li, S. K. Cushing, P. Zheng, T. Senty, F. Meng, A. D. Bristow, A. Manivannan and N. Wu, J. Am. Chem. Soc., 2014, 136, 8438 CrossRef CAS PubMed;
(b) Q. Xiang, J. Yu and M. Jaroniec, J. Am. Chem. Soc., 2012, 134, 6575 CrossRef CAS PubMed;
(c) F. Meng, J. Li, S. K. Cushing, M. Zhi and N. Wu, J. Am. Chem. Soc., 2013, 135, 10286 CrossRef CAS PubMed;
(d) C. Yang, Z. Wang, T. Lin, H. Yin, X. Lu, D. Wan, T. Xu, C. Zheng, J. Lin, F. Huang, X. Xie and M. Jiang, J. Am. Chem. Soc., 2013, 135, 17831 CrossRef CAS PubMed.
-
(a) S. R. Lingampalli and C. N. R. Rao, J. Mater. Chem. A, 2014, 2, 7702 RSC;
(b) J. Wang, Z. Guan, J. Huang, Q. Li and J. Yang, J. Mater. Chem. A, 2014, 2, 7960 RSC;
(c) S. Wang, L. Zhao, L. Bai, J. Yan, Q. Jiang and J. Lian, J. Mater. Chem. A, 2014, 2, 7439 RSC;
(d) J. Yang and H. S. Shin, J. Mater. Chem. A, 2014, 2, 5979 RSC.
-
(a) S. Fukuzumi and Y. Yamada, J. Mater. Chem., 2012, 22, 24284 RSC;
(b) S. Fukuzumi and T. Suenobu, Dalton Trans., 2013, 18 RSC;
(c) S. Fukuzumi, D. Hong and Y. Yamada, J. Phys. Chem. Lett., 2013, 4, 3458 CrossRef CAS;
(d) S. Fukuzumi and Y. Yamada, ChemSusChem, 2013, 6, 1834 CrossRef CAS PubMed;
(e) Y. Yamada, S. Shikano and S. Fukuzumi, J. Phys. Chem. C, 2013, 117, 13143 CrossRef CAS.
-
(a) H. Kotani, T. Ono, K. Ohkubo and S. Fukuzumi, Phys. Chem. Chem. Phys., 2007, 9, 1487 RSC;
(b) H. Kotani, R. Hanazaki, K. Ohkubo, Y. Yamada and S. Fukuzumi, Chem.–Eur. J., 2011, 17, 2777 CrossRef CAS PubMed;
(c) Y. Yamada, T. Miyahigashi, H. Kotani, K. Ohkubo and S. Fukuzumi, J. Am. Chem. Soc., 2011, 133, 16136 CrossRef CAS PubMed;
(d) Y. Yamada, T. Miyahigashi, H. Kotani, K. Ohkubo and S. Fukuzumi, Energy Environ. Sci., 2012, 5, 6111 RSC.
- Metal-free H2 evolution from polymeric carbon nitride has been reported. See: X. Wang, K. Maeda, A. Thomas, K. Takanabe, G. Xin, J. M. Carlsson, K. Domen and M. Antonietti, Nat. Mater., 2009, 8, 76 CrossRef CAS PubMed.
- Number of carbon atoms on SWCNT was estimated by diameter and length of SWCNT (ϕ = 1.4 nm; l = 1–5 μm) and carbon–carbon bond length (0.14 nm) to be 2.7 × 105 carbons per SWCNT. Number of moles of SWCNT in solution is calculated by 0.15 (mg)/(12 × 2.7 × 105) to be 4.5 × 10−8 mmol.
-
(a) M. G. Stevens, K. M. Sellers, S. Subramoney and H. C. Foley, Chem. Commun., 1998, 2679 RSC;
(b) J. Mortensen and J. Heinze, Angew. Chem., Int. Ed. Engl., 1984, 23, 84 CrossRef.
- K. Meerholz and J. Heinze, J. Am. Chem. Soc., 1989, 111, 2325 CrossRef CAS.
-
(a) C. G. Hatchard and C. A. Parker, Proc. R. Soc. London, Ser. A, 1956, 235, 518 CrossRef CAS;
(b)
J. G. Calvert and J. N. Pitts, Photochemistry, Wiley, New York, 1966, p. 783 Search PubMed.
-
(a) R. G. Kooser, W. V. Volland and J. H. Freed, J. Chem. Phys., 1969, 50, 5243 CrossRef CAS PubMed;
(b) B. G. Segal, M. Kaplan and G. K. Frankel, J. Chem. Phys., 1965, 43, 4191 CrossRef CAS PubMed.
- Aromatic carbon centered radical ion was observed close to g = 2.0023 which is a value of free spin. J. B. Jones and L. S. Singer, Carbon, 1982, 20, 379 CrossRef.
-
(a) J. Chen, M. A. Hamon, H. Hu, Y. Chen, A. M. Rao, P. C. Eklund and R. C. Haddon, Science, 1998, 282, 95 CrossRef CAS;
(b) H. Hu, B. Zhao, M. A. Hamon, K. Kamaras, M. E. Itkis and R. C. Haddon, J. Am. Chem. Soc., 2003, 125, 14893 CrossRef CAS PubMed.
- Y. Maeda, J. Higo, Y. Amagai, J. Matsui, K. Ohkubo, Y. Yoshigoe, M. Hashimoto, K. Eguchi, M. Yamada, T. Hasegawa, Y. Sato, J. Zhou, J. Lu, T. Miyashita, S. Fukuzumi, T. Murakami, K. Tohji, S. Nagase and T. Akasaka, J. Am. Chem. Soc., 2013, 135, 6356 CrossRef CAS PubMed.
- 0.19% of hydrogen may be produced from adsorbed water on SWCNTs surface, accompanied by the hydroxylation of SWCNTs.
- L. Turi and P. J. Rossky, Chem. Rev., 2012, 112, 5641 CrossRef CAS PubMed.
- Y. Muroya, M. Lin, V. de Waele, Y. Hatano, Y. Katsumura and M. Mostafavi, J. Phys. Chem. Lett., 2010, 1, 331 CrossRef CAS.
- B. Pastina and J. A. LaVerne, J. Phys. Chem. A, 1999, 103, 209 CrossRef CAS.
- C. Fourdrin, H. Aarrachi, C. Latrille, S. Esnouf, F. Bergaya and S. Le Caër, Environ. Sci. Technol., 2013, 47, 9530 CrossRef CAS PubMed.
-
W. L. F. Armarego and C. L. L. Chai, in Purification
of Laboratory Chemicals, Butterworth-Heinemann, Oxford, 6th edn, 2009 Search PubMed.
Footnote |
† Electronic supplementary information (ESI) available: GC and HPLC analyses for products characterisation (Fig. S1–S3 and S5), time course data of H2 evolution in various solvents (S4), IR (S6), TG (S7) and experimental details (S8). See DOI: 10.1039/c4sc02269f |
|
This journal is © The Royal Society of Chemistry 2015 |
Click here to see how this site uses Cookies. View our privacy policy here.