DOI:
10.1039/C4SC03516J
(Edge Article)
Chem. Sci., 2015,
6, 1979-1985
Chemiluminescent probes for imaging H2S in living animals†
Received
14th November 2014
, Accepted 31st December 2014
First published on 2nd January 2015
Abstract
Hydrogen sulphide (H2S) is an endogenous mediator of human health and disease, but precise measurement in living cells and animals remains a considerable challenge. We report the total chemical synthesis and characterization of three 1,2-dioxetane chemiluminescent reaction-based H2S probes, CHS-1, CHS-2, and CHS-3. Upon treatment with H2S at physiological pH, these probes display instantaneous light emission that is sustained for over an hour with high selectivity against other reactive sulphur, oxygen, and nitrogen species. Analysis of the phenol/phenolate equilibrium and atomic charges has provided a generally applicable predictive model to design improved chemiluminescent probes. The utility of these chemiluminescent reagents was demonstrated by applying CHS-3 to detect cellularly generated H2S using a multi-well plate reader and to image H2S in living mice using CCD camera technology.
Introduction
Hydrogen sulphide (H2S) is increasingly recognized as an important mediator of mammalian physiology and pathology, playing roles in vasorelaxation,1 angiogenesis,2 redox regulation,3 neuromodulation,4 lifespan,5 Huntington's disease,6 Down syndrome,7 diabetes,8 and cancer.9 In mammals, endogenous H2S is generated from cystathionine β-synthase (CBS),10 cystathionine γ-lyase (CSE),11 and 3-mercaptopyruvate sulphur transferase (3MST).12 Similar to its reactive cousins, nitric oxide (NO) and hydrogen peroxide (H2O2),13 H2S mediates cellular function via direct chemical interaction with biological molecules and can have widely disparate effects that depend on concentration, tissue localization, and the molecular environment.14–16 For example, H2S in colon cancer promotes tumour growth by stimulating angiogenesis and supporting cellular energetics,17 whilst H2S in prostate cancer slows cell growth, disrupts androgen receptor transactivation, and reduces angiogenesis by inhibiting the function of hypoxia-inducible factor 1.18 Given the delicate site- and concentration-dependent actions of H2S, easy methods for accurate spatiotemporal detection in living cells and animals are in urgent demand and promise to significantly contribute to an increased understanding of this reactive signalling molecule.
Common methods of H2S detection, including the methylene blue assay, ion-selective electrodes, amperometric sensors, and gas chromatography, have varying strengths and weaknesses, but all generally fall short of being able to detect H2S inside of intact living organisms.19 Fluorescent probes offer the ability to target specific analytes20,21 and there has been an explosion of recent activity in the development of dyes responsive to H2S and its derivatives.22–24 Unfortunately, imaging endogenous H2S remains rare, and often requires advanced confocal microscopy setups to allow precise same-cell tracking or two-photon excitation coupled with ratiometric imaging.25,26 This instrumentation is unavailable to many researchers, reducing the potential impact of these newly developed tools.27 Furthermore, there are few examples of in vivo imaging of H2S,28 and imaging deep mammalian tissue remains a frontier field for H2S detection. At the heart of the matter is a lack of sensitivity and depth penetration due to background autofluorescence, light scattering, photoactivation/photobleaching, and probe kinetics that requires long incubation times to accumulate signal. In order to address these technological challenges and develop tools to better elucidate the precise mechanisms of H2S production and function, we have herein developed a series of selective and sensitive chemiluminescent probes for rapid imaging of H2S in living animals.
Triggered chemiluminescence emission can provide a highly sensitive readout of biological analytes.29 Chemiluminescence doesn't require light excitation, thereby drastically reducing background from autofluorescence and photoactivation of azide functional groups. Whereas bioluminescence (chemiluminescence derived from living systems that express bioluminescent enzymes such as luciferase) has found wide application for preclinical analysis of biological parameters using genetically modified organisms,30 small molecule chemiluminescence can be used with wild-type animals and opens up exciting opportunities for clinical imaging. Recently, there have appeared select examples of chemiluminescent agents for the detection of H2S in vitro.31,32 Although quite promising, the need for enzymatic additives and alkaline conditions introduces cytotoxicity and can potentially release sulphide from proteins and other base-labile sulphur pools,33,34 ultimately hindering their potential for whole animal imaging.
To overcome these issues and expand the scope of chemiluminescent detection technology for small molecule biological analytes, we focused on using sterically stabilized 1,2-dioxetane systems35 to develop a series of new chemiluminescent H2S probes that display instantaneous light production under biologically compatible conditions. Sterically stabilized dioxetanes have been used for femtogram detection of enzymatic analytes36 and have demonstrated potential for in vivo imaging.37 We introduce three first generation chemiluminescent H2S probes, CHS-1, CHS-2, and CHS-3, derived from sterically stabilized spiroadamantane 1,2-dioxetane scaffolds modified with self-immolative 4-azidobenzyl carbonates as the H2S response site (Scheme 1).38 This article reports their synthetic preparation, optical response and selectivity for H2S, experimental and computational mechanistic investigations of their chemiluminescent response, detection of cellular H2S using a multi-well plate reader, and a noteworthy demonstration of whole animal chemiluminescent imaging of H2S.
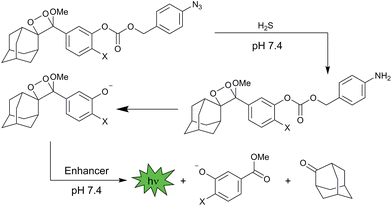 |
| Scheme 1 Spiroadamantane 1,2-dioxetanes for chemiluminescent H2S detection at neutral pH. | |
Results and discussion
Design and synthesis of CHS-1, CHS-2, and CHS-3
The CHS probe series was designed such that chemiluminescent emission would be initiated by the H2S-mediated reduction of the azide group, followed by self-immolative carbonate cleavage yielding the free phenolate bearing the 1,2-dioxetane (Scheme 1). The negatively charged phenolate will emit light spontaneously upon decomposition via an intramolecular chemically initiated electron exchange luminescence (CIEEL) mechanism.39–41 This newly produced light can be observed directly or by transfer of its energy to acceptor molecules like quantum dots, rhodamine, or fluorescein.42 In our studies, we employ a commercially available Emerald II Enhancer solution that consists of a cationic polymer and a dye with similar photophysical properties to fluorescein. The polymer reduces water-induced quenching by providing a hydrophobic environment for the chemiluminescent reaction and the dye effectively red shifts the luminescence emission making it more amenable for biological imaging applications.
By adapting a literature procedure,43 we optimized an efficient and modular synthetic route to access the probes CHS-1, CHS-2, and CHS-3 (Scheme 2). First, unsubstituted, fluorinated, and chlorinated 3-methoxybenzaldehyde derivatives 1a–c were reacted with trimethyl orthoformate in the presence of p-toluenesulfonic acid to give acetals 2a–c. These acetals were subjected to triethyl phosphite and boron trifluoride diethyl etherate at 0 °C, which afforded the diethyl methoxy (3-methoxyphenyl) methyl phosphonates 3a–c. Next, enol ethers 4a–c were obtained through Horner–Wadsworth–Emmons reaction by treating phosphonates 3a–c with nBuLi and 2-adamantanone.44 Nucleophilic demethylation using sodium ethanethiolate provided the phenols 5a–c. The activated ester 6, prepared according to analogous literature procedures, was coupled to the phenols to provide 7a–c, azide-bearing precursors to the final 1,2-dioxetanes.45,46 Finally, a [2 + 2] cycloaddition with singlet oxygen was accomplished by bubbling oxygen through a solution of 7a–c and the sensitizer Rose bengal with visible light irradiation, delivering the chemiluminescent probes CHS-1, CHS-2, and CHS-3.
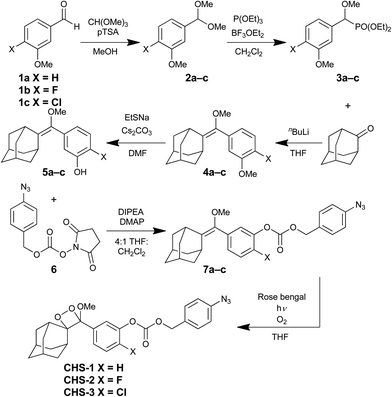 |
| Scheme 2 Syntheses of CHS-1, CHS-2, and CHS-3. | |
Response and selectivity
With the first three CHS probes in hand, we proceeded to measure their luminescent responses to H2S using an F-7000 Hitachi spectrophotometer. At pH 7.4, treatment of the CHS probes with H2S resulted in instantaneous luminescent emission that increased over a course of 10 minutes in a dose-dependent manner (Fig. 1). Addition of the fluorescein-based Emerald II Enhancer provided a red-shifted peak centred at 545 nm compared to the 470 nm emission of the phenolate (Fig. 1, insets). Under these physiologically relevant conditions, we observed an increase in the integrated luminescent response to H2S from CHS-1 to CHS-2 to CHS-3, giving 5-fold, 4-fold, and 12-fold turn-on responses respectively. It should be noted that no background corrections were performed and the values in Fig. 1 are direct instrumental values to provide an accurate comparison between probes. At pH 10, CHS-1 provided the highest chemiluminescence intensity and response, giving a 7-fold increase in photon emission in the first 10 minutes after adding 200 μM H2S (Fig. S1†). Under these alkaline conditions, the H2S-triggered chemiluminescent emissions of the fluorinated CHS-2 and chlorinated CHS-3 were lower than CHS-1. The background signal also increases with CHS-2 and CHS-3, reducing the relative increases over control to 2-fold and 3-fold respectively. A key advantage of these probes is that they display an immediate concentration-dependent emission of light that persists for over an hour (Fig. S2†), an ideal property for the high-throughput detection and imaging of H2S generated in biological environments.
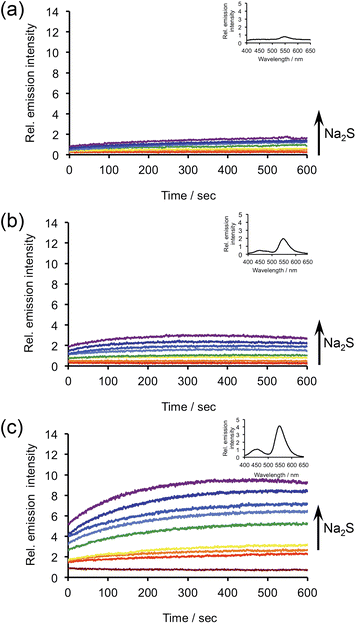 |
| Fig. 1 Time scans of the chemiluminescent emission at 545 nm from (a) 40 μM CHS-1, (b) 40 μM CHS-2, or (c) 40 μM CHS-3 and 0, 5, 10, 20, 40, 80, 100, 150, 200 μM Na2S in 20 mM HEPES buffer (pH 7.4) containing 20% Emerald II Enhancer. Insets are chemiluminescence spectra of (a) 40 μM CHS-1, (b) 40 μM CHS-2, and (c) 40 μM CHS-3 and 200 μM Na2S, acquired immediately after adding probes. | |
We next tested the selectivity of CHS-1, CHS-2, and CHS-3 for H2S against other biologically relevant reactive sulphur, oxygen, and nitrogen (RSON) species. The response of CHS-1 to 200 μM Na2S in 20 mM HEPES buffered to pH 7.4 was tested against other RSON species by adding 5 mM reduced glutathione (GSH), 1 mM L-cysteine and homocysteine, and 200 μM of S-nitrosoglutathione, sulfite (SO32−), hydrogen peroxide (H2O2), hypochlorite (OCl−), tert-butyl hydroperoxide (tBuOOH), nitroxyl (HNO), nitric oxide (NO), and nitrite (NO2−). None of the species tested displayed significant increases in luminescence intensity over the blank control (Fig. 2a). Additionally, the response to Na2S was minimally perturbed by the presence of physiological levels of GSH, L-cysteine, and homocysteine. We observed similar results when CHS-2 and CHS-3 were evaluated for their selectivity in 20 mM HEPES at pH 7.4 (Fig. 2b and c). These response and selectivity data demonstrate that CHS-1, CHS-2, and CHS-3 are able to detect H2S at physiologically relevant pH with minimal interference from competing analytes.
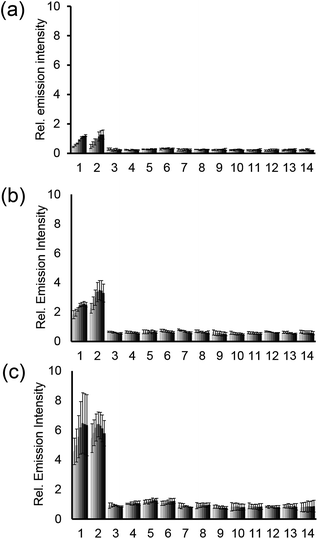 |
| Fig. 2 Chemiluminescent responses of (a) 40 μM CHS-1, (b) 40 μM CHS-2, or (c) 40 μM CHS-3 to biologically relevant RSON species in 20 mM HEPES buffer (pH 7.4) containing 20% Emerald II Enhancer. Bars represent chemiluminescent emission at 545 nm and 30, 60, 120, 240, 360, 480 and 600 s after addition of RSON species. Data shown are for 5 mM glutathione, 1 mM cysteine and homocysteine, and 200 μM for other RSON species. Legend: (1) Na2S; (2) Na2S, glutathione, L-cysteine, and homocysteine; (3) glutathione; (4) S-nitrosoglutathione; (5) L-cysteine; (6) homocysteine; (7) HNO; (8) NO; (9) NaNO2; (10) Na2SO3; (11) H2O2; (12) NaClO; (13) tBuOOH; (14) blank. Error bars are ± S.D. | |
Mechanistic studies of chemiluminescent H2S detection
We next sought to understand the factors that lead to increased light production and optimized response for 1,2-dioxetane chemiluminescent probes. First, we investigated the role of the phenol/phenolate equilibrium. Since the measurement of the pKa of the phenolate dioxetane products of the CHS probes is complicated by their rapid chemiluminescent decomposition, we used the reported experimental values of phenol, 2-fluorophenol, and 2-chlorophenol as an approximation.47 We plotted the integrated chemiluminescent emission of CHS-1, CHS-2, and CHS-3 in response to 200 μM Na2S at pH 7.4 and pH 10 (Fig. 3a and b) against the experimental pKa values for phenol, 2-fluorophenol, and 2-chlorophenol (Fig. 3c and d). The H2S-stimulated emission intensity at pH 7.4 displays an increasing trend with increased phenol acidity, indicating that ionization of the phenol is critical to attaining good luminescent response under these conditions (Fig. 3c). On the other hand, there is no clear correlation with phenol pKa and the chemiluminescent response at pH 10 (Fig. 3d). We further investigated the nature of the chemiluminescent emission by performing quantum chemistry calculations on the phenolate structures released from CHS-1, CHS-2, and CHS-3 after reaction with Na2S (Tables S1–S3†). Geometries were optimized using density functional theory (DFT) at the B3LYP/6-311+G(d,p) level of theory, and the charge on each atom was calculated using an electrostatic potential (ESP) model at the M06/6-311+G(d,p) level of theory. All calculations were carried out with the integral equation formalism polarizable continuum model (IEF-PCM) in water as solvent using the Gaussian 09 program package. While we found no correlation between the charge on the phenolic oxygen (O8) and the chemiluminescent response at pH 7.4 (Fig. 3e), an excellent correlation (R2 > 0.99) was observed with the response at pH 10 (Fig. 3f). ESP charge calculations performed with B3LYP and ωB97XD functionals also provided good correlations (R2 > 0.97) of the O8 charge and the chemiluminescent response at pH 10 (Fig. S3†). These data indicate that at lower pH, the chemiluminescent emission is governed by the equilibrium between the protonated phenol and unprotonated phenolate oxygen, and are in agreement with previous observations.48CHS-3 is the most readily ionized and therefore shows the highest chemiluminescent emission. On the other hand, at pH 10 the phenolate species dominates for all three deprotected dioxetanes and the efficiency of chemiluminescent emission is predicted by the more negative O8 charge density, probably due to an increased propensity towards initiation of the first intramolecular electron transfer step of CIEEL.49,50 These data reveal trends that, when taken together, provide a powerful predictive model for the design of improved chemiluminescent reagents.
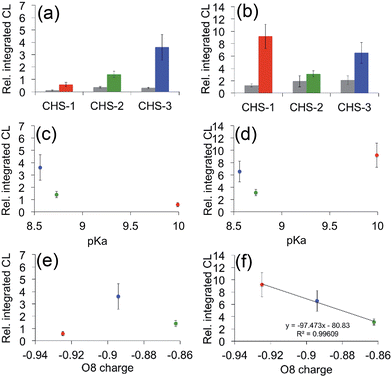 |
| Fig. 3 Analysis of chemiluminescent responses. (a and b) Bar graphs for the integrated chemiluminescent emission over 10 min at (a) pH 7.4 or (b) pH 10 of CHS-1, CHS-2, and CHS-3 to 0 μM Na2S (grey bars) and 200 μM Na2S (colored bars). (c and d) Plot of the integrated chemiluminescent emission over 10 min at (c) pH 7.4 or (d) pH 10 of 200 μM Na2S and 40 μM CHS-1 (red), CHS-2 (green) and CHS-3 (blue) versus experimental pKa values of model compounds phenol, 2-fluorophenol, and 2-chlorophenol. (e and f) Plot of the integrated chemiluminescent emission over 10 min at (e) pH 7.4 or (f) pH 10 of 200 μM Na2S and 40 μM CHS-1 (red), CHS-2 (green) and CHS-3 (blue) versus the calculated atomic charge on the phenolate oxygen (O8). All luminescent measurements were acquired in 20 mM HEPES buffer (pH 7.4) or 100 mM glycine buffer (pH 10) containing 20% Emerald II Enhancer. The reported values are averages of the integrated emission intensities over 10 min (n = 4–7). Error bars represent ± S.D. The ESP atomic charges were calculated at the M06/6-311+G(d,p) level of theory using geometries optimized at the B3LYP/6-311+G(d,p) level of theory. Calculations were carried out with the IEF-PCM water solvation model using the Gaussian 09 program package. | |
Using CHS-3 to detect cellular H2S
Our results show that CHS-3 provides the most robust light emission at physiological pH when compared to CHS-1 and CHS-2. We therefore examined the ability of CHS-3 to detect cellular H2S production in human lung adenocarcinoma epithelial cells (A549). These cells express the enzyme cystathionine γ-lyase (CSE),51 which can utilize homocysteine (Hcy) as a substrate for H2S production. We first demonstrated the ability of CHS-3 to detect exogenous Na2S in a multi-well plate reader format (Fig. 4a), and determined an estimated detection limit (blank control + 3 S.D.) of 5.4 μM. We then incubated A549 cells with Hcy, a substrate for the enzyme CSE. This resulted in ∼10% increase (n = 12, p = 0.044) in luminescent emission from CHS-3 compared with vehicle treated cells (Fig. 4b). Pre-incubation with the CSE inhibitor D,L-propargylglycine (PAG) before adding Hcy attenuates signal observed from CHS-3. Although the observed increase is small, it is statistically significant and the efficacy of CHS-3 for endpoint detection of H2S generated by whole cells at physiological pH is an important advance that sets the stage for imaging H2S in living animals.
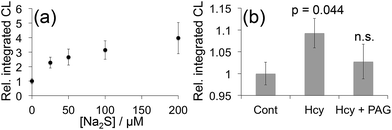 |
| Fig. 4 Detection of cellular H2S using a multi-well plate reader. (a) Luminescent responses of 40 μM CHS-3 and 0, 25, 50, 100, and 200 μM Na2S in 20 mM HEPES buffer (pH 7.4) containing 20% Emerald II Enhancer. (b) A549 cells were treated with a vehicle control (Cont), 200 μM Hcy (Hcy), or 200 μM Hcy after being pre-treated with 200 μM PAG (Hcy + PAG) for 20 min. 20 min after incubating with Hcy or vehicle, the cells were washed and treated with 40 μM CHS-3 and 125 μL Emerald II Enhancer. The reported values represent the average luminescent intensity of replicate experiments (n = 12, p = 0.044). Error bars represent ± S.E.M. | |
In vivo imaging of H2S using CHS-3
We next investigated the ability of CHS-3 to image H2S at physiological pH using an IVIS Spectrum. An opaque 96-well plate was loaded with 0, 25, 50, 100, and 200 μM Na2S in 20 mM HEPES buffer (pH 7.4) containing 20% Emerald II Enhancer. CHS-3 was added at 40 μM and imaged to reveal a clear increase in luminescence intensity with increasing H2S concentrations (Fig. 5a). Averages of repeated experiments provide a good linear response in the range of 0–200 μM when imaged 30 s after exposure to H2S (Fig. 5b). We next applied a mouse carcass model to determine if CHS-3 would have sufficient light output to be observable through mammalian tissue. The carcasses of sacrificed SCID/BALB-C mice were injected with CHS-3 and either a vehicle control (Fig. S4a and b†) or 0.4 μmol H2S (Fig. S4c and d†) into the peritoneal cavity. An increase in luminescence could be clearly observed in the peritoneum of the carcass injected with H2S versus the carcass injected with the vehicle control.
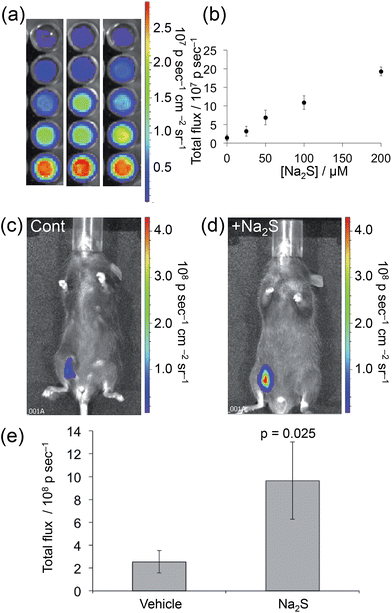 |
| Fig. 5 Imaging H2S using CHS-3. (a) Images 30 s after adding 40 μM CHS-3 to 0, 25, 50, 100, and 200 μM Na2S in 20 mM HEPES buffer (pH 7.4) containing 20% Emerald II Enhancer (n = 3). (b) Plot of total photon flux versus H2S concentration for the experiments described in (a). (c and d) Images of living C6 brown mice 30 s after administering i.p. injections of 0.08 μmol CHS-3 and (c) vehicle control or (d) 0.4 μmol Na2S in 100 μL 20 mM HEPES buffer (pH 7.4) containing 20% Emerald II Enhancer. (e) Quantification of the total photon flux from three replicates of the experiments described in (c) and (d). Statistical analyses were performed with a two-tailed Student's t-test (n = 3, p = 0.025). Error bars are ± S.D. | |
Confident that light emission from CHS-3 was capable of significant tissue penetration, we finally sought to establish the ability of CHS-3 to image H2S in living animals. C6 brown mice were administered i.p. injections on one side of their peritoneal cavity. The skin was raised during injections to avoid puncturing internal organs. Images were acquired 30 s after injecting 0.08 μmol CHS-3 and either 0.4 μmol Na2S or a vehicle control (H2O) in 100 μL HEPES buffered at pH 7.4 containing 20% Emerald II Enhancer. The final concentration of Na2S in the injection was 4 mM. While vehicle control experiments produced modest signal (Fig. 5c), the mice that received Na2S treatments displayed robust emission of light that was easily detected through their tissue (Fig. 5d). Quantification of the total photon flux from replicate experiments (Fig. S5†) revealed ∼4-fold increase (n = 3, p = 0.025) in the luminescence response in the Na2S treated mice versus vehicle controls (Fig. 5e). The agents were well tolerated and the mice showed no immediate outward signs of malaise. Taken together, these data provide a key milestone towards the development of a new class of in vivo imaging tools for investigating biological hydrogen sulphide.
Conclusions
We have designed and synthesized three 1,2-dioxetane chemiluminescent reaction-based H2S probes, CHS-1, CHS-2, and CHS-3 that display immediate light emission upon reacting with H2S at physiological pH, a significant advance for H2S detection technology. These reagents provide a sensitive and selective detection platform for H2S using spectrophotometers, multi-well plate readers, and IVIS Spectrum instruments. We have provided a computational and experimentally supported mechanistic framework that serves as a predictive model for designing sterically hindered 1,2-dioxetanes with improved light emission based on the pKa of the phenol released after reacting with H2S and the relative atomic charge densities of these structures. Finally, we demonstrated that CHS-3 was not only capable of detecting cellular H2S produced by A549 cells treated with homocysteine at neutral pH, but also has the rare ability to image H2S in living animals. While CHS-3 provides an exciting proof of principle for in vivo chemiluminescence imaging, we are currently engaged in improving the sensitivity and biocompatibility of chemiluminescent 1,2-dioxetane reagents for diverse applications, and anticipate that these new optimized systems will provide powerful whole animal imaging tools across a range of biological analytes and parameters.
Acknowledgements
This work was supported by Southern Methodist University (start-up funds to A.R.L) and the Center for Drug Design, Discovery, and Delivery (CD4 seed money to A.R.L.). Imaging was facilitated by the SW-SAIR, a Resource of the Simmons Cancer Center supported in part by NIH P30 1CA142543 and used an IVIS Spectrum, which was purchased under NIH 1S10RR024757. The Texas Advanced Computing Center (TACC) at the University of Texas at Austin and Southern Methodist University's Center for Scientific Computation provided computing resources. We thank Maciej Kukula of the Shimadzu Center for Advanced Analytical Chemistry at the University of Texas at Arlington for acquiring high-resolution mass spectrometry data. The authors would also like to thank Dr Son's research group for use of the irradiation lamp.
Notes and references
- W. Zhao and R. Wang, Am. J. Physiol.: Heart Circ. Physiol., 2002, 283, H474 CAS.
- A. Papapetropoulos, A. Pyriochou, Z. Altaany, G. Yang, A. Marazioti, Z. Zhou, M. G. Jeschke, L. K. Branski, D. N. Herndon, R. Wang and C. Szabó, Proc. Natl. Acad. Sci. U. S. A., 2009, 106, 21972 CrossRef CAS PubMed.
-
(a) Y. Kimura and H. Kimura, FASEB J., 2004, 18, 1165 CAS;
(b) M. Lee, C. Schwab, S. Yu, E. McGeer and P. L. McGeer, Neurobiol. Aging, 2009, 30, 1523 CrossRef CAS PubMed.
- K. Abe and H. Kimura, J. Neurosci., 1996, 16, 1066 CAS.
- D. L. Miller and M. B. Roth, Proc. Natl. Acad. Sci. U. S. A., 2007, 104, 20618 CrossRef CAS PubMed.
- B. D. Paul, J. I. Sbodio, R. Xu, M. S. Vandiver, J. Y. Cha, A. M. Snowman and S. H. Snyder, Nature, 2014, 509, 96 CrossRef CAS PubMed.
- P. Kamoun, M. C. Belardinelli, A. Chabli, K. Lallouchi and B. Chadefaux-Vekemans, Am. J. Med. Genet., Part A, 2003, 116, 310 CrossRef PubMed.
- L. Wu, W. Yang, X. Jia, G. Yang, D. Duridanova, K. Cao and R. Wang, Lab. Invest., 2009, 89, 59 CrossRef CAS PubMed.
-
(a) J. Huang, S. Kumar, N. Abbassi-Ghadi, P. Španěl, D. Smith and G. B. Hanna, Anal. Chem., 2013, 85, 3409 CrossRef CAS PubMed;
(b) C. Szabó, C. Coletta, C. Chao, K. Módis, B. Szczesny, A. Papapetropoulos and M. R. Hellmich, Proc. Natl. Acad. Sci. U. S. A., 2013, 110, 12474 CrossRef PubMed.
- S. Singh, D. Padovani, R. A. Leslie, T. Chiku and R. Banerjee, J. Biol. Chem., 2009, 284, 22457 CrossRef CAS PubMed.
- M. H. Stipanuk and P. W. Beck, Biochem. J., 1982, 206, 267 CAS.
- N. Shibuya, M. Tanaka, M. Yoshida, Y. Ogasawara, T. Togawa, K. Ishii and H. Kimura, Antioxid. Redox Signaling, 2009, 11, 703 CrossRef CAS PubMed.
- J. M. Fukuto, S. J. Carrington, D. J. Tantillo, J. G. Harrison, L. J. Ignarro, B. A. Freeman, A. Chen and D. A. Wink, Chem. Res. Toxicol., 2012, 25, 769 CrossRef CAS PubMed.
-
(a) A. K. Mustafa, M. M. Gadalla, N. Sen, S. Kim, W. Mu, S. K. Gazi, R. K. Barrow, G. Yang, R. Wang and S. H. Snyder, Sci. Signaling, 2009, 2, ra72 Search PubMed;
(b) T. Ida, T. Sawa, H. Ihara, Y. Tsuchiya, Y. Watanabe, Y. Kumagai, M. Suematsu, H. Motohashi, S. Fujii, T. Matsunaga, M. Yamamoto, K. Ono, N. O. Devarie-Baez, M. Xian, J. M. Fukuto and T. Akaike, Proc. Natl. Acad. Sci. U. S. A., 2014, 111, 7606 CrossRef CAS PubMed.
- T. L. Guidotti, Int. J. Toxicol., 2010, 29, 569 CrossRef CAS PubMed.
- K. Módis, C. Coletta, K. Erdélyi, A. Papapetropoulos and C. Szabo, FASEB J., 2012, 27, 601 CrossRef PubMed.
- C. Szabo, C. Coletta, C. Chao, K. Módis, B. Szczesny, A. Papapetropoulos and M. R. Hellmich, Proc. Natl. Acad. Sci. U. S. A., 2013, 110, 12474 CrossRef CAS PubMed.
-
(a) B. Wu, H. Teng, G. Yang, L. Wu and R. Wang, Br. J. Pharmacol., 2012, 167, 1492 CrossRef CAS PubMed;
(b) K. Zhao, S. Li, L. Wu, C. Lai and G. Yang, J. Biol. Chem., 2014, 289, 20824 CrossRef CAS PubMed.
- K. R. Olson, E. R. DeLeon and F. Liu, Nitric Oxide, 2014, 41, 11 CrossRef CAS PubMed.
- Reviews:
(a) H. Kobayashi, M. Ogawa, R. Alford, P. L. Choyke and Y. Urano, Chem. Rev., 2010, 110, 2620 CrossRef CAS PubMed;
(b) J. Chan, S. C. Dodani and C. J. Chang, Nat. Chem., 2012, 2, 973 CrossRef PubMed;
(c) Y. Yang, Q. Zhao, W. Feng and F. Li, Chem. Rev., 2013, 114, 192 CrossRef PubMed;
(d) Y. You and W. Nam, Chem. Sci., 2014, 5, 4123 RSC.
- Recent examples:
(a) T. Hirayama, G. C. Van de Bittner, L. W. Gray, S. Lutsenko and C. J. Chang, Proc. Natl. Acad. Sci. U. S. A., 2012, 109, 2228 CrossRef CAS PubMed;
(b) T. Hirayama, K. Okuda and H. Nagasawa, Chem. Sci., 2013, 4, 1250 RSC;
(c) H. Y. Au-Yeung, J. Chan, T. Chantarojsiri and C. J. Chang, J. Am. Chem. Soc., 2013, 135, 15165 CrossRef CAS PubMed;
(d) J. Yeow, A. Kaur, M. D. Anscomb and E. J. New, Chem. Commun., 2014, 50, 8181 RSC;
(e) E. L. Smith, C. R. Bertozzi and K. E. Beatty, ChemBioChem, 2014, 15, 1101 CrossRef CAS PubMed;
(f) J. Yin, Y. Kwon, D. Kim, D. Lee, G. Kim, Y. Hu, J. H. Ryu and J. Yoon, J. Am. Chem. Soc., 2014, 136, 5351 CrossRef CAS PubMed;
(g) A. R. Longstreet, M. Jo, R. R. Chandler, K. Hanson, N. Zhan, J. J. Hrudka, H. Mattoussi, M. Shatruk and D. T. McQuade, J. Am. Chem. Soc., 2014, 136, 15493 CrossRef CAS PubMed.
- Reviews:
(a) W. Xuan, C. Sheng, Y. Cao, W. He and W. Wang, Angew. Chem., Int. Ed., 2012, 51, 2282 CrossRef CAS PubMed;
(b) V. S. Lin and C. J. Chang, Curr. Opin. Chem. Biol., 2012, 16, 595 CrossRef CAS PubMed;
(c) C. X. Duan and Y. G. Liu, Curr. Med. Chem., 2013, 20, 2929 CrossRef CAS;
(d) N. Kumar, V. Bhalla and M. Kumar, Coord. Chem. Rev., 2013, 257, 2335 CrossRef CAS PubMed;
(e) G. K. Kolluru, X. Shen, S. C. Bir and C. G. Kevil, Nitric Oxide, 2013, 5 CrossRef CAS PubMed;
(f)
M. D. Pluth, T. S. Bailey, M. D. Hammers and L. A. Montoya, in Biochalcogen Chemistry: The Biological chemistry of Sulfur, Selenium, and Tellurium, 2013, p. 15 Search PubMed;
(g) A. R. Lippert, J. Inorg. Biochem., 2014, 133, 136 CrossRef CAS PubMed;
(h) B. Peng and M. Xian, Asian J. Org. Chem., 2014, 3, 914 CrossRef CAS;
(i) V. S. Lin, W. Chen, M. Xian and C. J. Chang, Chem. Soc. Rev., 2014 10.1039/C4CS00298A , Advance Article.
- Recent examples:
(a) A. R. Lippert, E. J. New and C. J. Chang, J. Am. Chem. Soc., 2011, 133, 10078 CrossRef CAS PubMed;
(b) H. Peng, Y. Cheng, C. Dai, A. L. King, B. L. Predmore, D. J. Lefer and B. Wang, Angew. Chem., Int. Ed., 2011, 50, 9672 CrossRef CAS PubMed;
(c) Y. Qian, J. Karpus, O. Kabil, S. Y. Zhang, H. L. Zhu, R. Banerjee, J. Zhao and C. He, Nat. Commun., 2011, 2, 495 CrossRef PubMed;
(d) K. Sasakura, K. Hanaoka, N. Shibuya, Y. Mikami, Y. Kimura, T. Ueno, T. Komatsu, T. Terai, H. Kimura and T. Nagano, J. Am. Chem. Soc., 2011, 133, 18003 CrossRef CAS PubMed;
(e) C. Liu, J. Pan, S. Li, Y. Zhao, L. Y. Wu, C. E. Berkman, A. R. Whorton and M. Xian, Angew. Chem., Int. Ed., 2011, 50, 10327 CrossRef CAS PubMed;
(f) C. Liu, B. Peng, S. Li, C. M. Park, A. R. Whorton and M. Xian, Org. Lett., 2012, 14, 2184 CrossRef CAS PubMed;
(g) L. A. Montoya and M. D. Pluth, Chem. Commun., 2012, 48, 4767 RSC;
(h) S. K. Das, C. S. Lim, S. Y. Yang, J. H. Han and B. R. Cho, Chem. Commun., 2012, 48, 8395 RSC.
-
(a) W. Chen, C. Liu, B. Peng, Y. Zhao, A. Pacheco and M. Xian, Chem. Sci., 2013, 4, 2892 RSC;
(b) C. Liu, W. Chen, W. Shi, B. Peng, Y. Zhao, H. Ma and M. Xian, J. Am. Chem. Soc., 2014, 136, 7257 CrossRef CAS PubMed.
- V. S. Lin, A. R. Lippert and C. J. Chang, Proc. Natl. Acad. Sci. U. S. A., 2013, 110, 7131 CrossRef CAS PubMed.
- S. K. Bae, C. H. Heo, D. J. Choi, D. Sen, E. H. Joe, B. R. Cho and H. M. Kim, J. Am. Chem. Soc., 2013, 135, 9915 CrossRef CAS PubMed.
- A. Papapetropoulos, M. Whiteman and G. Cirino, Br. J. Pharmacol., 2014 DOI:10.1111/bph.12806 , in press.
-
(a) Q. Wan, Y. Song, Z. Li, X. Gao and H. Ma, Chem. Commun., 2013, 49, 502 RSC;
(b) W. Sun, J. Fan, C. Hu, J. Cao, H. Zhang, X. Xiong, J. Wang, S. Cui, S. Sun and X. Peng, Chem. Commun., 2013, 49, 3890 RSC;
(c) N. Dufton, J. Natividad, E. F. Verdu and J. L. Wallace, Sci. Rep., 2012, 2, 499 Search PubMed.
-
(a) S. Sabelle, P. Y. Renard, K. Pecorella, S. de Suzzoni-Dézard, C. Créminon, J. Grassi and C. Mioskowski, J. Am. Chem. Soc., 2002, 124, 4874 CrossRef CAS PubMed;
(b) J. A. Richard, L. Jean, A. Romieu, M. Massonneau, P. Noack-Fraissignes and P. Y. Renard, Org. Lett., 2007, 9, 4853 CrossRef CAS PubMed;
(c) J. Koci, V. Grandclaude, M. Massonneau, J. A. Richard, A. Romieu and P. Y. Renard, Chem. Commun., 2011, 47, 6713 RSC;
(d) J. M. Baumes, J. J. Gassensmith, J. Giblin, J. J. Lee, A. G. White, W. J. Culligan, W. M. Leevy, M. Kuno and B. D. Smith, Nat. Chem., 2010, 2, 1025 CrossRef CAS PubMed;
(e) I. S. Turan and E. U. Akkaya, Org. Lett., 2014, 16, 1680 CrossRef CAS PubMed;
(f) D. Lee, S. Khaja, C. J. Velasquez-Castano, M. Dasari, C. Sun, J. Petros, W. R. Taylor and N. Murthy, Nat. Mater., 2007, 6, 765 CrossRef CAS PubMed.
- K. O'Neill, S. K. Lyons, W. M. Gallagher, K. M. Curran and A. T. Byrne, J. Pathol., 2010, 220, 317 Search PubMed.
- T. S. Bailey and M. P. Pluth, J. Am. Chem. Soc., 2013, 135, 16697 CrossRef CAS PubMed.
- I. S. Turan and F. Sozmen, Sens. Actuators, B, 2014, 201, 13 CrossRef PubMed.
- V. du Vigneaud, J. Biol. Chem., 1927, 75, 393 CAS.
- T. S. Bailey, L. N. Zakharov and M. D. Pluth, J. Am. Chem. Soc., 2014, 136, 10573 CrossRef CAS PubMed.
-
(a) A. P. Schaap and S. D. Gagnon, J. Am. Chem. Soc., 1982, 104, 3504 CrossRef CAS;
(b) A. P. Schaap, T. S. Chen, R. S. Handley, R. DeSilva and B. P. Giri, Tetrahedron Lett., 1987, 28, 1155 CrossRef CAS;
(c) L. F. M. L. Ciscato, D. Weiss, R. Beckert and W. J. Baader, J. Photochem. Photobiol., A, 2011, 218, 41 CrossRef CAS PubMed;
(d) M. Matsumoto and N. Watanabe, Bull. Chem. Soc. Jpn., 2005, 78, 1899 CrossRef CAS;
(e) N. Watanabe, H. Kino, H. K. Ijuin, M. Yamada and M. Matsumoto, Tetrahedron, 2012, 68, 6079 CrossRef CAS PubMed.
- I. Bronstein, C. S. Martin, J. J. Fortin, C. E. Olesen and J. C. Voyta, Clin. Chem., 1996, 42, 1542 CAS.
- L. Liu and R. P. Mason, PLoS One, 2010, 5, e12024 Search PubMed.
- S. Gnaim and D. Shabat, Acc. Chem. Res., 2014, 47, 2970 CrossRef CAS PubMed.
- G. B. Schuster, Acc. Chem. Res., 1979, 12, 366 CrossRef CAS.
- W. Adam, I. Bronstein, A. V. Trofimov and R. F. Vasil'ev, J. Am. Chem. Soc., 1999, 121, 958 CrossRef CAS.
- L. F. M. L. Ciscato, F. A. Augusto, D. Weiss, F. H. Bartoloni, E. L. Bastos, S. Albrecht, H. Brandl, T. Zimmermann and W. J. Baader, ARKIVOC, 2012, 3, 391 CrossRef.
- J. Y. Park, J. Gunpat, L. Liu, B. Edwards, A. Christie, X. J. Xie, L. J. Kricka and R. P. Mason, Luminescence, 2014, 29, 553 CrossRef CAS PubMed.
-
(a)
I. Y. Bronstein, B. Edwards and A. L. Sparks, U. S. Pat. 5,538,847, filed May 7, 1993, and issued July 23, 1996;
(b)
I. Y. Bronstein, B. Edwards, A. L. Sparks and J. C. Voyta, U. S. Pat. WO1997014954 A1, filed Oct 17, 1996, and issued Aug 24, 1997.
- C. A. Roeschlaub and P. G. Sammes, J. Chem. Soc., Perkin Trans. 1, 2000, 2243 RSC.
- T. Chen, Y. Zheng, Z. Xu, M. Zhao, Y. Xu and J. Cui, Tetrahedron Lett., 2013, 54, 2980 CrossRef CAS PubMed.
- A. R. Lippert, T. Gschneidtner and C. J. Chang, Chem. Commun., 2010, 46, 7510 RSC.
-
(a) M. D. Lipak, K. C. Gross, P. G. Seybold, S. Feldgus and G. C. Shields, J. Am. Chem. Soc., 2002, 124, 6421 CrossRef PubMed;
(b) J. Han and F. M. Tao, J. Phys. Chem. A, 2006, 110, 257 CrossRef CAS PubMed.
- M. Matsumoto, J. Photochem. Photobiol., C, 2004, 5, 27 CrossRef CAS PubMed.
- L. F. M. L. Ciscato, F. H. Bartoloni, D. Weiss, R. Beckert and W. J. Baader, J. Org. Chem., 2010, 75, 6574 CrossRef CAS PubMed.
- E. L. Bastos, S. da Silva and W. J. Baader, J. Org. Chem., 2013, 78, 4432 CrossRef CAS PubMed.
- L. P. Fang, Q. Lin, C. S. Tang and X. M. Liu, Pharmacol. Res., 2010, 61, 298 CrossRef CAS PubMed.
Footnote |
† Electronic supplementary information (ESI) available: Detailed experimental procedures, characterization of compounds, computational methods, optimized geometries, atomic charges, supplementary figures, and replicate images. See DOI: 10.1039/c4sc03516j |
|
This journal is © The Royal Society of Chemistry 2015 |
Click here to see how this site uses Cookies. View our privacy policy here.