DOI:
10.1039/C5SC01784J
(Edge Article)
Chem. Sci., 2015,
6, 5172-5176
Water stabilization of Zr6-based metal–organic frameworks via solvent-assisted ligand incorporation†
Received
16th May 2015
, Accepted 19th June 2015
First published on 1st July 2015
Abstract
Water stability in metal–organic frameworks (MOFs) is critical for several practical applications. While water instability is mainly thought to stem from linker hydrolysis, MOFs with strong, hydrolysis-resistant metal-linker bonds can be susceptible to damage by capillary forces, which cause cavities and channels to collapse during activation from water. This study utilizes metal node functionalization as a strategy to create vapor-stable and recyclable MOFs.
Introduction
Porous metal–organic frameworks (MOFs) are hybrid solid-state compounds1 that have attracted voluminous research efforts for applications relevant to gas storage and separation,2 chemical catalysis,3 and optoelectronic4 and thermoelectric devices.5 However, many of these applications require recyclability, which is intimately tied to the stability of the corresponding frameworks in ambient conditions as well as in the presence of water.5,6 It is worth noting that only a modest fraction of the total known MOFs are both thermally and chemically stable. Stable MOFs include those derived from nodes with high valent metal ions7 and some imidazolate, pyrazolate, and multitopic carboxylate linkers. Among the more remarkable examples are ZIFs,8 pyrazolate-based frameworks,9 MILs,10 and ZrIV-based UiOs.7,11 While the microporous UiO-66 framework, consisting of 12-connected [Zr6(μ3–O)4(μ3–OH)4]12+ nodes linked through 1,4-benzene-dicarboxylates (BDC),7 was established to be mechanically,7,12 thermally,7 hydrolytically and chemically stable,7,13 other Zr6 based MOFs with larger pores, such as UiO-67, constructed with a longer linker (BPDC, 4,4′-biphenyl-dicarboxylate), have often been found to lose porosity upon exposure and activation from water.13b,14 We recently showed that the instability of these Zr6-based MOFs is not due to hydrolysis, which is an energetically disfavored process (ΔG ≈ +26 kcal mol−1 with ΔG‡ ≈ +38 kcal mol−1) owing to a strong ZrIV-carboxylate connectivity, but instead stems from capillary-force-driven channel collapse during removal of water.13a The water-exposed MOF sample, when exchanged with a solvent exerting less capillary force, was shown to retain permanent porosity by thermal activation under dynamic vacuum.13a,15 Some applications of MOFs, however, may not be compatible with stepwise solvent evacuation.6,13b,16 The availability of an alternative stabilization method clearly would be desirable.
Given the breadth of applications specifically of Zr6-based MOFs to condensed-phase chemistry,17 we have focused on understanding the origin of such capillary-force-driven collapse, while devising a strategy that would enable MOFs to be recycled (i.e. repetitively solvent evacuated) via simple thermal activation. The Zr6-based mesoporous MOFs are intriguing, particularly those featuring eight-connected [Zr6(μ3–O)4(μ3–OH)4(–OH)4(–OH2)4]8+ nodes linked by tetra-carboxylate ligands (Fig. 1a) giving rise to framework structures with hydroxyl and aqua ligands18 protruding into mesoporous channels. These non-bridging hydroxyl and aqua ligands provide polar and tunable primary interaction points or ‘anchors’ for water clusters to attach5 and are, thus, ideal for studying the role of polar nodes in capillary-force-driven pore collapse.
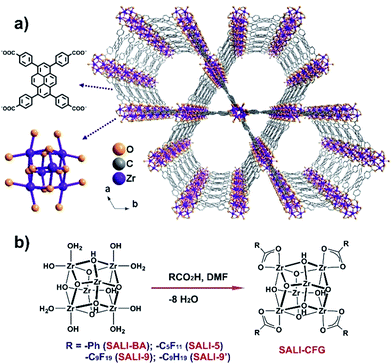 |
| Fig. 1 (a) Structure of NU-1000 (H-atoms were removed for clarity) and (b) preparation of SALI-CFGs via Solvent-Assisted Ligand Incorporation (SALI). | |
We have established that the non-bridging hydroxyl and aqua ligands of the Zr6-nodes of the mesoporous MOF NU-1000 [molecular formula Zr6(μ3–O)4(μ3–OH)4(–OH)4(–OH2)4(TBAPy)2; Fig. 1a; H4TBAPy is the 1,3,6,8-tetrakis(p-benzoic-acid)pyrene]17c,18-19 can facilitate a variety of node-functionalization schemes including metalation via AIM (Atomic layer deposition In a Metal–organic framework)17c and grafting organic chemical functionalities via SALI (Solvent-Assisted Ligand Incorporation; Fig. 1b).19,20 These modifications not only facilitate various chemical processes,19,20 but also potentially provide a means to control the pore diameter and the accessibility of the nodes by condensed water. For example, a SALI-derived NU-1000 framework possesses a node coordination similar to that established in the UiO-66 structure,19i.e. a [Zr6(μ3–O)4(μ3–OH)4]12+ node with twelve carboxylate ligands without any terminal hydroxyl or aqua ligand (Fig. 1b). Recent literature reports have established that in microporous UiO-66 and isostructural MOF-801 (with fumarate as the linker),5,6 the bridging hydroxo ligands on the Zr6-oxo node play a key role as a primary interaction site for the guest water molecules, which form a hydrogen bonding network that facilitates further uptake of water molecules. Since SALI-decoration in NU-1000 replaces the terminal hydroxo and aqua ligands at the Zr6-oxo nodes, the incoming carboxylate functional groups (CFGs) of various size not only reduce the pore volume but also potentially produce a ‘protected’ node that interacts less strongly with water molecules (Fig. 1b).5,6,21
Results and discussion
Given that NU-1000 undergoes capillary-force-driven pore collapse when activated (thermal evacuation) from its liquid-water-filled form,13a we wanted to test its stability under water vapor conditions at various vapor partial pressures (or relative humidities, RH) using N2 as carrier gas at a constant total pressure of 1 bar.22 As can be seen in Fig. 2a, at RH ≈ 70%, the mesoporous NU-1000 channels undergo capillary condensation and reach saturation with 1.14 mg mg−1 total uptake (corresponding to a water accessible pore volume of 1.14 cc g−1, where the N2 accessible pore volume = 1.4 cc g−1).23 Removal of the condensed water, during the desorption cycle, was achieved by progressive lowering of the vapor concentration or partial pressure with dry N2 carrier gas and occurs with considerable hysteresis. However, the second adsorption isotherm, under identical conditions, evinces only a 0.43 mg mg−1 saturation uptake. The decrease in the total uptake indicates a decrease in the porosity, which possibly commenced during the first desorption cycle. Likewise, the third adsorption shows 0.31 mg mg−1 of total uptake with a further decrease of the porosity. The powder X-ray diffraction (PXRD) pattern of the recovered sample revealed a lower crystallinity (Fig. 2c), as the diffraction peaks centered at 2.55, 5.1 and 7.4 degrees (2θ) are significantly broadened and the peaks at higher angles less intense. Consistent with these findings, the N2 isotherm of the recovered (after the third cycle), thermally activated sample shows significant reductions of both the BET surface area (320 m2 g−1) and the N2 accessible total pore volume down (0.15 cc g−1) (Fig. 3a and Table 1).
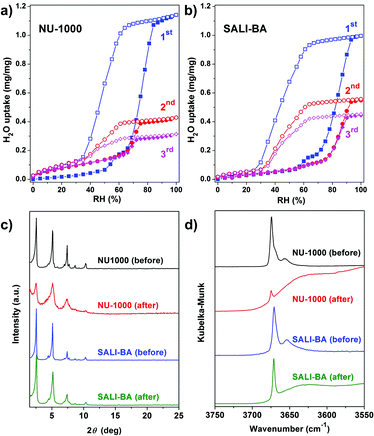 |
| Fig. 2 Multi-cycle water vapor adsorption (solid symbols) and desorption (open symbols) isotherms of (a) NU-1000 and (b) SALI-BA at 298 K; (c) PXRD patterns and (d) DRIFTS data for NU-1000 and SALI-BA samples before and after three water isotherm cycles. | |
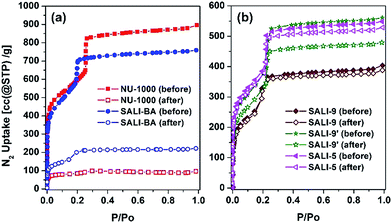 |
| Fig. 3 N2 adsorption isotherms for (a) NU-1000 and (b) SALI-CFG samples before (solid symbol) and after (open symbol) multi-cycle (twenty cycles, except for three-cycle experiments for NU-1000 and SALI-BA) water vapor sorption experiments. | |
Table 1 BET surface areas, pore volumes, multi-cycle water uptake for NU-1000 and SALI-CFG Samples
MOF |
Ligand |
BET surface area (m2 g−1) |
Pore volume (cc g−1) |
Multi-cycle saturation H2O uptake (mg mg−1); T = 298 K |
BET surface area (m2 g−1) after H2O sorption |
1st |
2nd |
3rd |
20th |
NU-1000
|
OH−, H2O |
2145 |
1.46 |
1.14 |
0.43 |
0.31 |
— |
320 |
SALI-BA
|
C6H5CO2− |
2005 |
1.21 |
1.00 |
0.56 |
0.45 |
— |
570 |
SALI-5
|
CF3(CF2)4CO2− |
1270 |
0.85 |
0.72 |
0.72 |
0.71 |
0.70 |
1250 |
SALI-9
|
CF3(CF2)8CO2− |
900 |
0.63 |
0.44 |
0.43 |
0.43 |
0.43 |
890 |
SALI-9′
|
CH3(CH2)8CO2− |
1190 |
0.87 |
0.78 |
0.73 |
0.72 |
0.68 |
1170 |
To gain insight into the role of the polar hydroxyl and aqua ligands, we compared the results for NU-1000 with those obtained benzoate functionalized NU-1000 (termed SALI-BA), where the accessible terminal hydroxyl and aqua ligands are replaced by benzoate ligands (Fig. 1b). Similar to NU-1000, SALI-BA is subject to capillary condensation at RH ≈ 70% and reaches a water saturation uptake of 1.0 mg mg−1, which tracks with the N2 accessible pore volume (1.21 cc g−1; Fig. 2b; Table 1). SALI-BA exhibited a diminished uptake during the second adsorption. However, the corresponding reduction of the pore volume is less severe (∼44%) than that observed for the unfunctionalized NU-1000 (∼62%). The magnitude of the pore volume reduction suggests that a benzoate functionalized node (1) provides a less effective ‘anchoring’ site and (2) engenders lower pore volume for the capillary-condensed water, rendering a lower degree of damage during the desorption. The corresponding PXRD pattern and the N2 isotherm of the recovered (after third cycle), thermally activated sample indicated better retention of crystallinity and porosity relative to the unfunctionalized compound (see Fig. 2c and 3a). DRIFTS data of the recovered SALI-BA sample show retention of the characteristic bridging hydroxyl peak at 3671 cm−1 whereas the recovered unfunctionalized NU-1000 displays a broad brand at the expense of the sharp peaks at 3674 and 3671 cm−1 associated with the terminal and bridging hydroxyl and the terminal aqua ligands, respectively (Fig. 2d). These data suggest node structure alteration and possibly a partially pore-collapsed framework. We now turn our attention to SALI-CFG materials featuring flexible functional groups that may protect the node further by inhibiting access of condensed water to the MOF node. Perfluorodecanoic-acid-functionalized NU-1000 (termed SALI-9)19b is an example of a highly-stable mesoporous MOF with a reduced pore volume, rendering a total water vapor uptake that is ∼40% as great as that of unfunctionalized NU-1000 (Fig. 4, ESI-4a;†Table 1). Remarkably, over the course of 20 cycles of water vapor sorption/desorption, the porosity and crystallinity of SALI-9 remain essentially unchanged. It is worth noting that the N2-accessible pore volume of SALI-9 sample is measured to be 0.63 cc g−1,19bi.e. somewhat lower than that of the parent material and consistent with the volumetric demands of the perfluoroalkane chains themselves. Even though functionalization significantly reduces the MOF pore volume, it is interesting to note that water condensation,5 in SALI-9, commences at essentially the same vapor pressure as for NU-1000 (Fig. 2). Corresponding contact angle measurements (Fig. ESI-7†), a bulk measurement of external-surface interactions with liquid water, indicate enhanced hydrophobicity for SALI-9 relative to unfunctionalized NU-1000. The recovered sample (after 20 cycles) after thermal activation reveals essentially no degradation of the crystallinity, porosity and Zr6-oxo node structure (see Fig. 3, ESI-5 and 6†).
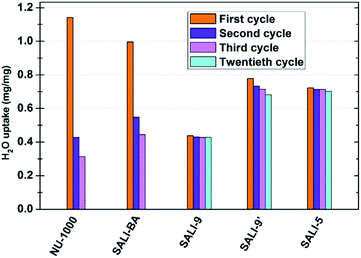 |
| Fig. 4 Saturation water uptake for NU-1000 and SALI-CFG samples in multi-cycle water vapor sorption experiments recorded at 298 K. | |
To understand if the acquired stability is due mainly to the hydrophobic nature of the perfluoroalkane decoration or to concurrent reduction of the pore size and, therefore, water cluster size, we studied the corresponding –C9H19 and the shorter –C5F11 chains in NU-1000 (SALI-9′ and SALI-5, respectively). Due to its lower molar mass compared to the corresponding –C9F19 variant, SALI-9′ shows higher gravimetric vapor uptake (ca. 68% of that of the unfunctionalize MOF; Fig. 4, ESI-4†). The stability of SALI-9′ to repetitive infilling and removal of water is nearly as good as that of SALI-9, as can be seen from a modest 10% decrease in saturation water uptake after 20 cycles of vapor sorption and desorption.24 The N2 isotherm, PXRD, and DRIFTS data (Fig. 3 and ESI 5 and 6†) of the recovered thermally activated sample reveal essentially complete retention of the crystallinity, porosity, and node coordination environment. Likewise, the shorter perfluoroalkane variant, SALI-5, shows excellent performance: significantly higher water uptake (∼63% of NU-1000) along with stability (Fig. 3 and 4; Table 1) similar to that of SALI-9.
Given that the pyrene macrocycle of the NU-1000 linker is hydrophobic, these experimental results led us to hypothesize that within the mesoporous MOF channel, the polar [Zr6(μ3–O)4(μ3–OH)4(–OH)4(–OH2)4(–COO8)] node strongly interacts with the condensed water. Thus during the desorption cycle, condensed water can exert significant capillary force, which in turn results in pore collapse.13a,15 In the SALI-CFG samples, the terminal hydroxyl and aqua ligands were replaced with aromatic or alkyl carboxylates that limit the accessibility of the polar Zr6-oxo nodes by water. Effective protection is tied to both the size and hydrophobicity of the CFG introduced by the SALI reaction.
To gain molecular insight into this hypothesis, we performed molecular dynamics (MD) simulations for water in NU-1000, SALI-BA and SALI-5. (see ESI section 7 for details.†) The simulations predict that the number of water molecules sited within 15 Å of the Zr6-nodes varies with MOF identity in the order NU-1000 > SALI-BA > SALI-5 (Table S4†). Likewise the density of water in the hexagonal channels (which could affect the capillary force) is ranked as NU-1000 > SALI-BA ∼ SALI-5 (Table S3†). These results support our hypothesis that the improved stability in SALI-derived system is due to limited accessibility of water molecules near the Zr6-nodes.
Conclusions
In conclusion, our results suggest that strong interactions of condensed water with the polar nodes within the framework of NU-1000 can exert strain during removal of the water from the pores. While replacement of the polar hydroxyl and aqua ligands with aprotic, non-polar organic carboxylates can reduce the strength of the interaction of water with the node, a flexible alkyl chain can further limit the accessibility by water vapor. MD simulations revealed that a longer and flexible carboxylate-based ligand indeed provides more limited access of water molecules to the Zr6 nodes. Node functionalization, by methods such as SALI, can be an effective strategy for engendering MOF stability toward water removal and, therefore, MOF recyclability from liquid water without loss of permanent porosity.
Acknowledgements
OKF gratefully acknowledges funding from the Army Research Office (project number W911NF-13-1-0229). JTH and RQS gratefully acknowledge support from the Global Climate and Energy Project (GCEP).
Notes and references
-
(a) G. Férey, Chem. Soc. Rev., 2008, 37, 191–214 RSC
;
(b) S. Horike, S. Shimomura and S. Kitagawa, Nat. Chem., 2009, 1, 695–704 CrossRef CAS PubMed
;
(c) O. M. Yaghi, M. O'Keeffe, N. W. Ockwig, H. K. Chae, M. Eddaoudi and J. Kim, Nature, 2003, 423, 705–714 CrossRef CAS PubMed
.
-
(a) Y. He, W. Zhou, G. Qian and B. Chen, Chem. Soc. Rev., 2014, 43, 5657–5678 RSC
;
(b) O. K. Farha, A. Ö. Yazaydın, I. Eryazici, C. D. Malliakas, B. G. Hauser, M. G. Kanatzidis, S. T. Nguyen, R. Q. Snurr and J. T. Hupp, Nat. Chem., 2010, 2, 944–948 CrossRef CAS PubMed
;
(c) R. B. Getman, Y.-S. Bae, C. E. Wilmer and R. Q. Snurr, Chem. Rev., 2012, 112, 703–723 CrossRef CAS PubMed
;
(d) J.-R. Li, R. J. Kuppler and H.-C. Zhou, Chem. Soc. Rev., 2009, 38, 1477–1504 RSC
;
(e) J.-R. Li, J. Sculley and H.-C. Zhou, Chem. Rev., 2012, 112, 869–932 CrossRef CAS PubMed
;
(f) L. J. Murray, M. Dincă and J. R. Long, Chem. Soc. Rev., 2009, 38, 1294–1314 RSC
;
(g) Y. Peng, V. Krungleviciute, I. Eryazici, J. T. Hupp, O. K. Farha and T. Yildirim, J. Am. Chem. Soc., 2013, 135, 11887–11894 CrossRef CAS PubMed
;
(h) M. P. Suh, H. J. Park, T. K. Prasad and D.-W. Lim, Chem. Rev., 2012, 112, 782–835 CrossRef CAS PubMed
;
(i) K. Sumida, D. L. Rogow, J. A. Mason, T. M. McDonald, E. D. Bloch, Z. R. Herm, T.-H. Bae and J. R. Long, Chem. Rev., 2012, 112, 724–781 CrossRef CAS PubMed
;
(j) C. E. Wilmer, O. K. Farha, Y.-S. Bae, J. T. Hupp and R. Q. Snurr, Energy Environ. Sci., 2012, 5, 9849–9856 RSC
;
(k) C. E. Wilmer, O. K. Farha, T. Yildirim, I. Eryazici, V. Krungleviciute, A. A. Sarjeant, R. Q. Snurr and J. T. Hupp, Energy Environ. Sci., 2013, 6, 1158–1163 RSC
;
(l) H.-C. Zhou, J. R. Long and O. M. Yaghi, Chem. Rev., 2012, 112, 673–674 CrossRef CAS PubMed
.
-
(a) J. Liu, L. Chen, H. Cui, J. Zhang, L. Zhang and C.-Y. Su, Chem. Soc. Rev., 2014, 43, 6011–6061 RSC
;
(b) J. Lee, O. K. Farha, J. Roberts, K. A. Scheidt, S. T. Nguyen and J. T. Hupp, Chem. Soc. Rev., 2009, 38, 1450–1459 RSC
;
(c) L. Ma, C. Abney and W. Lin, Chem. Soc. Rev., 2009, 38, 1248–1256 RSC
;
(d) M. Yoon, R. Srirambalaji and K. Kim, Chem. Rev., 2012, 112, 1196–1231 CrossRef CAS PubMed
.
-
(a) Z. Hu, B. J. Deibert and J. Li, Chem. Soc. Rev., 2014, 43, 5815–5840 RSC
;
(b) L. E. Kreno, K. Leong, O. K. Farha, M. Allendorf, R. P. Van Duyne and J. T. Hupp, Chem. Rev., 2012, 112, 1105–1125 CrossRef CAS PubMed
;
(c) Y. Cui, Y. Yue, G. Qian and B. Chen, Chem. Rev., 2012, 112, 1126–1162 CrossRef CAS PubMed
;
(d) S. Jin, H.-J. Son, O. K. Farha, G. P. Wiederrecht and J. T. Hupp, J. Am. Chem. Soc., 2013, 135, 955–958 CrossRef CAS PubMed
;
(e) C. A. Kent, D. Liu, L. Ma, J. M. Papanikolas, T. J. Meyer and W. Lin, J. Am. Chem. Soc., 2011, 133, 12940–12943 CrossRef CAS PubMed
;
(f) C. A. Kent, B. P. Mehl, L. Ma, J. M. Papanikolas, T. J. Meyer and W. Lin, J. Am. Chem. Soc., 2010, 132, 12767–12769 CrossRef CAS PubMed
;
(g) A. Shigematsu, T. Yamada and H. Kitagawa, J. Am. Chem. Soc., 2011, 133, 2034–2036 CrossRef CAS PubMed
;
(h) M. So, S. Jin, H.-J. Son, G. P. Wiederrecht, O. K. Farha and J. T. Hupp, J. Am. Chem. Soc., 2013, 135, 15698–15701 CrossRef CAS PubMed
;
(i) H.-J. Son, S. Jin, S. Patwardhan, S. J. Wezenberg, N. C. Jeong, M. So, C. E. Wilmer, A. A. Sarjeant, G. C. Schatz, R. Q. Snurr, O. K. Farha, G. P. Wiederrecht and J. T. Hupp, J. Am. Chem. Soc., 2013, 135, 862–869 CrossRef CAS PubMed
;
(j) M. L. Aubrey, R. Ameloot, B. M. Wiers and J. R. Long, Energy Environ. Sci., 2014, 7, 667–671 RSC
;
(k) S. Horike, D. Umeyama and S. Kitagawa, Acc. Chem. Res., 2013, 46, 2376–2384 CrossRef CAS PubMed
;
(l) N. C. Jeong, B. Samanta, C. Y. Lee, O. K. Farha and J. T. Hupp, J. Am. Chem. Soc., 2012, 134, 51–54 CrossRef CAS PubMed
;
(m) G. K. H. Shimizu, J. M. Taylor and S. Kim, Science, 2013, 341, 354–355 CrossRef CAS PubMed
;
(n) C. Wang, T. Zhang and W. Lin, Chem. Rev., 2012, 112, 1084–1104 CrossRef CAS PubMed
.
- J. Canivet, A. Fateeva, Y. Guo, B. Coasne and D. Farrusseng, Chem. Soc. Rev., 2014, 43, 5594–5617 RSC
.
-
(a) H. Furukawa, F. Gándara, Y.-B. Zhang, J. Jiang, W. L. Queen, M. R. Hudson and O. M. Yaghi, J. Am. Chem. Soc., 2014, 136, 4369–4381 CrossRef CAS PubMed
;
(b) N. C. Burtch, H. Jasuja and K. S. Walton, Chem. Rev., 2014, 114, 10575–10612 CrossRef CAS PubMed
;
(c) J. J. Low, A. I. Benin, P. Jakubczak, J. F. Abrahamian, S. A. Faheem and R. R. Willis, J. Am. Chem. Soc., 2009, 131, 15834–15842 CrossRef CAS PubMed
;
(d) E. Soubeyrand-Lenoir, C. Vagner, J. W. Yoon, P. Bazin, F. Ragon, Y. K. Hwang, C. Serre, J.-S. Chang and P. L. Llewellyn, J. Am. Chem. Soc., 2012, 134, 10174–10181 CrossRef CAS PubMed
;
(e) P. Guo, D. Dutta, A. G. Wong-Foy, D. W. Gidley and A. J. Matzger, J. Am. Chem. Soc., 2015, 137, 2651–2657 CrossRef CAS PubMed
;
(f) K. A. Cychosz and A. J. Matzger, Langmuir, 2010, 26, 17198–17202 CrossRef CAS PubMed
.
- J. H. Cavka, S. Jakobsen, U. Olsbye, N. Guillou, C. Lamberti, S. Bordiga and K. P. Lillerud, J. Am. Chem. Soc., 2008, 130, 13850–13851 CrossRef PubMed
.
- K. S. Park, Z. Ni, A. P. Côté, J. Y. Choi, R. Huang, F. J. Uribe-Romo, H. K. Chae, M. O'Keeffe and O. M. Yaghi, Proc. Natl. Acad. Sci. U. S. A., 2006, 103, 10186–10191 CrossRef CAS PubMed
.
- V. Colombo, S. Galli, H. J. Choi, G. D. Han, A. Maspero, G. Palmisano, N. Masciocchi and J. R. Long, Chem. Sci., 2011, 2, 1311–1319 RSC
.
- G. Férey, C. Mellot-Draznieks, C. Serre, F. Millange, J. Dutour, S. Surblé and I. Margiolaki, Science, 2005, 309, 2040–2042 CrossRef PubMed
.
-
(a) V. Bon, I. Senkovska, I. A. Baburin and S. Kaskel, Cryst. Growth Des., 2013, 13, 1231–1237 CrossRef CAS
;
(b) V. Bon, I. Senkovska, M. S. Weiss and S. Kaskel, CrystEngComm, 2013, 15, 9572–9577 RSC
.
-
(a) H. Wu, Y. S. Chua, V. Krungleviciute, M. Tyagi, P. Chen, T. Yildirim and W. Zhou, J. Am. Chem. Soc., 2013, 135, 10525–10532 CrossRef CAS PubMed
;
(b) H. Wu, T. Yildirim and W. Zhou, J. Phys. Chem. Lett., 2013, 4, 925–930 CrossRef CAS
.
-
(a) J. E. Mondloch, M. J. Katz, N. Planas, D. Semrouni, L. Gagliardi, J. T. Hupp and O. K. Farha, Chem. Commun., 2014, 50, 8944–8946 RSC
;
(b) J. B. DeCoste, G. W. Peterson, H. Jasuja, T. G. Glover, Y.-g. Huang and K. S. Walton, J. Mater. Chem. A, 2013, 1, 5642–5650 RSC
.
- G. C. Shearer, S. Forselv, S. Chavan, S. Bordiga, K. Mathisen, M. Bjørgen, S. Svelle and K. P. Lillerud, Top. Catal., 2013, 56, 770–782 CrossRef CAS PubMed
.
- J. E. Mondloch, O. Karagiaridi, O. K. Farha and J. T. Hupp, CrystEngComm, 2013, 15, 9258–9264 RSC
.
-
(a) A. Ö. Yazaydın, A. I. Benin, S. A. Faheem, P. Jakubczak, J. J. Low, R. R. Willis and R. Q. Snurr, Chem. Mater., 2009, 21, 1425–1430 CrossRef
;
(b) C. Janiak and S. K. Henninger, Chimia, 2013, 67, 419–424 CrossRef CAS PubMed
;
(c) S. K. Henninger, F. Jeremias, H. Kummer and C. Janiak, Eur. J. Inorg. Chem., 2012, 2625–2634 CrossRef CAS PubMed
;
(d) J.-P. Zhang, A.-X. Zhu, R.-B. Lin, X.-L. Qi and X.-M. Chen, Adv. Mater., 2011, 23, 1268–1271 CrossRef CAS PubMed
;
(e) F. Jeremias, A. Khutia, S. K. Henninger and C. Janiak, J. Mater. Chem., 2012, 22, 10148–10151 RSC
.
-
(a) Y. Chen, T. Hoang and S. Ma, Inorg. Chem., 2012, 51, 12600–12602 CrossRef CAS PubMed
;
(b) D. Feng, Z.-Y. Gu, J.-R. Li, H.-L. Jiang, Z. Wei and H.-C. Zhou, Angew. Chem., Int. Ed., 2012, 51, 10307–10310 CrossRef CAS PubMed
;
(c) J. E. Mondloch, W. Bury, D. Fairen-Jimenez, S. Kwon, E. J. DeMarco, M. H. Weston, A. A. Sarjeant, S. T. Nguyen, P. C. Stair, R. Q. Snurr, O. K. Farha and J. T. Hupp, J. Am. Chem. Soc., 2013, 135, 10294–10297 CrossRef CAS PubMed
;
(d) W. Morris, B. Volosskiy, S. Demir, F. Gándara, P. L. McGrier, H. Furukawa, D. Cascio, J. F. Stoddart and O. M. Yaghi, Inorg. Chem., 2012, 51, 6443–6445 CrossRef CAS PubMed
;
(e) F. Vermoortele, M. Vandichel, B. Van de Voorde, R. Ameloot, M. Waroquier, V. Van Speybroeck and D. E. De Vos, Angew. Chem., Int. Ed., 2012, 51, 4887–4890 CrossRef CAS PubMed
;
(f) D. Feng, Z.-Y. Gu, Y.-P. Chen, J. Park, Z. Wei, Y. Sun, M. Bosch, S. Yuan and H.-C. Zhou, J. Am. Chem. Soc., 2014, 136, 17714–17717 CrossRef CAS PubMed
;
(g) H.-L. Jiang, D. Feng, K. Wang, Z.-Y. Gu, Z. Wei, Y.-P. Chen and H.-C. Zhou, J. Am. Chem. Soc., 2013, 135, 13934–13938 CrossRef CAS PubMed
;
(h) S. Pullen, H. Fei, A. Orthaber, S. M. Cohen and S. Ott, J. Am. Chem. Soc., 2013, 135, 16997–17003 CrossRef CAS PubMed
;
(i) H. Fei, S. Pullen, A. Wagner, S. Ott and S. M. Cohen, Chem. Commun., 2015, 51, 66–69 RSC
;
(j) J. E. Mondloch, M. J. Katz, W. C. Isley III, P. Ghosh, P. Liao, W. Bury, G. W. Wagner, M. G. Hall, J. B. DeCoste, G. W. Peterson, R. Q. Snurr, C. J. Cramer, J. T. Hupp and O. K. Farha, Nat. Mater., 2015, 14, 512–516 CrossRef PubMed
;
(k) D. Feng, T.-F. Liu, J. Su, M. Bosch, Z. Wei, W. Wan, D. Yuan, Y.-P. Chen, X. Wang, K. Wang, X. Lian, Z.-Y. Gu, J. Park, X. Zou and H.-C. Zhou, Nat. Commun., 2015, 6, 5979 CrossRef PubMed
;
(l) S. B. Kalidindi, S. Nayak, M. E. Briggs, S. Jansat, A. P. Katsoulidis, G. J. Miller, J. E. Warren, D. Antypov, F. Corà, B. Slater, M. R. Prestly, C. Mart-Gastaldo and M. J. Rosseinsky, Angew. Chem., Int. Ed., 2015, 54, 221–226 CrossRef CAS PubMed
;
(m) K. E. deKrafft, W. S. Boyle, L. M. Burk, O. Z. Zhou and W. Lin, J. Mater. Chem., 2012, 22, 18139–18144 RSC
;
(n) K. Tulig and K. S. Walton, RSC Adv., 2014, 4, 51080–51083 RSC
;
(o) P. Nunes, A. C. Gomes, M. Pillinger, I. S. Gonçalves and M. Abrantes, Microporous Mesoporous Mater., 2015, 208, 21–29 CrossRef CAS PubMed
;
(p) P. Li, R. C. Klet, S.-Y. Moon, T. C. Wang, P. Deria, A. W. Peters, B. M. Klahr, H.-J. Park, S. S. Al-Juaid, J. T. Hupp and O. K. Farha, Chem. Commun., 2015 10.1039/C5CC03398E
.
- N. Planas, J. E. Mondloch, S. Tussupbayev, J. Borycz, L. Gagliardi, J. T. Hupp, O. K. Farha and C. J. Cramer, J. Phys. Chem. Lett., 2014, 5, 3716–3723 CrossRef CAS
.
-
(a) P. Deria, W. Bury, J. T. Hupp and O. K. Farha, Chem. Commun., 2014, 50, 1965–1968 RSC
;
(b) P. Deria, J. E. Mondloch, E. Tylianakis, P. Ghosh, W. Bury, R. Q. Snurr, J. T. Hupp and O. K. Farha, J. Am. Chem. Soc., 2013, 135, 16801–16804 CrossRef CAS PubMed
.
-
(a) P. Deria, W. Bury, I. Hod, C.-W. Kung, O. Karagiaridi, J. T. Hupp and O. K. Farha, Inorg. Chem., 2015, 54, 2185–2192 CrossRef CAS PubMed
;
(b) I. Hod, W. Bury, D. M. Gardner, P. Deria, V. Roznyatovskiy, M. R. Wasielewski, O. K. Farha and J. T. Hupp, J. Phys. Chem. Lett., 2015, 6, 586–591 CrossRef CAS
.
-
(a) H. Jasuja, Y.-g. Huang and K. S. Walton, Langmuir, 2012, 28, 16874–16880 CrossRef CAS PubMed
;
(b) D. Ma, Y. Li and Z. Li, Chem. Commun., 2011, 47, 7377–7379 RSC
.
- Given that the assessment of crystallinity and porosity from PXRD and N2 isotherm of a partially collapsed MOF sample could be misleading, recording multi-cycle water isotherm is preferable to assess the water stability [ref. 5 and 6a].
- Pore volumes were calculated from the saturation uptake (at STP) as the volume of corresponding amount of liquids.
- Note that the major decrease in water uptake was recorded during the second cycle, one reason can be that the studied sample was not fully decorated with decanoic acid (ca. 3.8 instead of 4 per Zr6-oxo node; see ESI section S3†). Thus the unfunctionalized node sites can potentially bahave like those in NU-1000.
Footnote |
† Electronic supplementary information (ESI) available. See DOI: 10.1039/c5sc01784j |
|
This journal is © The Royal Society of Chemistry 2015 |
Click here to see how this site uses Cookies. View our privacy policy here.