Flexible metal–organic framework-based one-dimensional photonic crystals†
Received
10th July 2014
, Accepted 23rd October 2014
First published on 28th October 2014
Abstract
A flexible metal–organic framework (MOF)-based one-dimensional photonic crystal (1DPC) was fabricated by a spin-coating method. The flexible MOF, NH2-MIL-88B (MIL = Materials from Institute Lavoisier), was selected and implanted as the intrinsic functional layer of 1DPCs because of its selective breathing behavior upon exposure to various guests. TiO2 nanoparticles were used as another component to ensure a high refractive index contrast. The optical properties of the 1DPCs were tailored by varying the number of bilayers, incident angles, and physical thickness of the individual slabs. The fabricated 1DPCs showed a selective response toward various organic vapors as a result of the selective breathing behavior of the NH2-MIL-88B layer. Selective quantitative measurements of the ethanol concentration were achieved when the 1DPC was exposed to the vapors of an ethanol and water mixture. The fabricated 1DPCs exhibited high long-term, thermal, and mechanical stability, which are beneficial for practical applications.
Introduction
One-dimensional photonic crystals (1DPCs), also known as Bragg stacks or Bragg mirrors, consist of alternating layers of materials with high and low refractive indices and planar interfaces between each pair of layers.1 They have distinct wavelengths of reflection, which are governed by the thickness and associated refractive index (RI) of each layer; this distinction endows their specific color.2 With the change in wavelength of maximum reflectance, the color changes depending on the thickness and associated RI caused by an ex-stimulus. Such color changes can be visually recognized by the naked eye. These features make 1DPCs excellent platforms for a variety of physical, chemical, and biological sensors.2,3
1DPCs have been known for many decades by optical scientists and are one of the simplest possible structures of photonic crystals with the easiest to predict optical properties. However, limited attention has been given to 1DPCs because of the lack of dynamic tunability of their structures and the high cost of fabrication. Therefore, new chemically active materials with a thickness and RI, which can be dynamically tuned, can be used as alternatives to the conventional passive condensed layer of 1DPCs. Many efforts have been devoted for implementing functionality within 1DPC by the bottom–up assembly of tailor-made inorganic or hybrid materials, such as dielectric and conductive metal oxides,4–7 sol–gel-based materials,8–10 zeolites,11 clays,12–14 super-paramagnetic colloidal particles,15,16 liquid crystals,17,18 organic polymers,19–21 and hydrogels.21,22 However, the inorganic layers of 1DPCs typically show a limited tuning range of the diffraction wavelength owing to the sluggish volume change and small changes in RI. 1DPCs, which contain a polymer layer, normally exhibit a pronounced shift in the diffraction peak by ex-stimuli. Nevertheless, their disadvantages include the complicated synthesis of specific polymers, poor stability, and complex preparation process.23–27 Therefore, achieving highly stable 1DPCs with an intrinsic functional layer, which can change both the thickness and RI remains a challenge.
Metal–organic frameworks (MOFs)28 are a class of hybrid materials formed by the self-assembly of metal ions or metal oxide clusters and organic bridging ligands. MOFs are attractive candidates for chemical sensing because of their modular tailorability, rich host–guest interactions, and widely tunable sorption behavior.29–33 Hinterholzinger et al.34 reported the first MOF-based 1DPC, in which ZIF-8 was used as the active component. Sensing can be realized by the observation of an optical signal displayed by distinct stop band shifts upon analyte sorption; however, they developed MOFs with rigid structures. Only the effective RI of MOFs evidently changed upon the adsorption of guests; this phenomenon is similar to that of an inorganic material. A unique type of MOF with a flexible framework has been developed.35–37 These MOFs can largely expand without apparent bond breaking in the network upon exposure to environmental vapors and gases or the adsorption of other guest molecules. In the present study, we correlated this “breathing effect” with the potential implication in 1DPCs. NH2-MIL-88B29,38–41 (MIL = Materials from Institute Lavoisier) materials are typical flexible MOFs, which have been investigated since their exceptionally large “breathing effect” was reported in 2007.
In this paper, we report the first flexible MOF-based 1DPCs, which were fabricated by a spin-coating method (Scheme 1). The MOF, NH2-MIL-88B material, was selected and implanted as an alternating thin layer of 1DPCs. TiO2 nanoparticles were used as another component to ensure high RI contrast. The optical response of the 1DPCs can be tailored by changing either the number of bilayers, incident angles, or physical thickness of the individual slabs. The fabricated 1DPCs show a selective response toward various organic vapors as a result of the change in RI contrast and physical thickness originating from the adsorption of organic vapors in the nanopores of the NH2-MIL-88B layer. Furthermore, a quantitative measurement of the ethanol (EtOH) concentration was performed, in which a linear shift in the reflection peak of the 1DPC was observed upon exposure to the vapors of EtOH and water mixture.
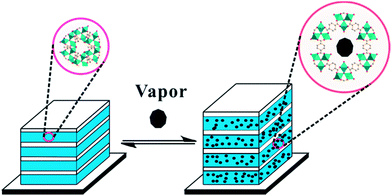 |
| Scheme 1 Schematic representation of flexible MOF-based 1DPCs on silicon wafers upon exposure to chemical vapors. | |
Experimental section
Materials
FeCl3·6H2O (97%), 2-aminoterephthanlic acid (H2N-BDC, 99%), pluronic F127 (EO97PO69EO97, Mn 7000–12
000), CH3COOH (≥99.5%), acetone, methanol (MeOH), EtOH, iso-propanol (i-PrOH), N,N-dimethylformamide (DMF), and H2O were used as received without further purification. Silicon wafers (2 cm × 2 cm) were used as substrates for the deposition of 1DPCs. The substrates were pre-cleaned with soap and water, and subsequently treated with Piranha solution (H2SO4/H2O2, volumetric ratio 7
:
3). After thorough rinsing with deionized water, the wafers were dried under a hot air flow and stored in EtOH.
Preparation of TiO2 sol
The TiO2 sol was synthesized using a procedure based on the hydrolysis of titanium butoxide.19,42 In brief, 4 mL titanium butoxide was dissolved in 2 mL i-PrOH in a round-bottom flask and stirred for 5 min. DI water (0.21 g) and concentrated HCl (17 μL) were mixed with 4 mL i-PrOH and stirred for 5 min. The mixture solution was dripped into a solution in a round-bottom flask for approximately 10 min. The resulting solution was stirred for 12 h at room temperature. Then, a colloidal suspension of anatase crystallites was obtained. For powder X-ray diffraction (XRD), the colloid was dried at 550 °C for 1 h in air.
Preparation of NH2-MIL-88B alcoholic suspensions
NH2-MIL-88B alcoholic suspensions were synthesized following previous reports.43,44 An aqueous solution of FeCl3·6H2O was poured into an F127 aqueous solution. The resulting solution was stirred for 1 h before injecting 0.2 mL acetic acid. After stirring for an additional 1 h, H2N-BDC was added. The reaction mixture was stirred for 2 h before transferring to a Teflon autoclave for crystallization for 12 h at 110 °C. The dark brown solid product was centrifuged, washed and re-dispersed in EtOH to obtain colloidal suspensions for spin-coating. For the analyses of the 1DPCs, the particles were dried under vacuum at 60 °C.
Fabrication of 1DPCs
To obtain different film thicknesses, the TiO2 sol was diluted with special volumes of i-PrOH, and NH2-MIL-88B alcoholic suspensions were diluted with different volumes of EtOH, prior to use. The 1DPCs were fabricated by spin-coating 200 μL of NH2-MIL-88B alcoholic suspensions or TiO2 sol alternately at 3000 rpm for 60 s onto a silicon wafer. Each layer was baked at 200 °C for 20 min. All the 1DPCs consisted of four (NH2-MIL-88B/TiO2) bilayers, except those used to examine the effect of the bilayer number.
Organic vapor testing
Organic vapor testing was conducted in a quartz cuvette (10 mm × 10 mm × 45 mm). After adding 200 μL of the solvents into the cuvette, typical 1DPCs deposited on silicon wafers (9 mm × 15 mm) were carefully fixed in the cuvette without forming contact with the solvents. The reflection spectra of the 1DPCs were monitored at 20 °C. A similar method was used for the quantitative measurements of the EtOH concentration. The concentration of EtOH was varied by adding 20 μL EtOH each time to 180 μL H2O.
Characterization
Scanning electron microscopy (SEM) images were obtained using a Hitachi S-4800 electron microscope with a primary electron energy of 5.0 kV. The samples were sputtered with a thin layer of Au prior to imaging. Transmission electron microscopy (TEM, JEOL JEM 1230 electron microscope) was employed with an accelerating voltage of 200 kV. Powder X-ray diffraction (XRD) patterns were collected using a Bruker D8 in theta–theta geometry from 5° to 50° (2θ) using a step size of 0.02° and 2 s per step in continuous mode with a graphite-monochromated CuKα radiation source. Fourier transform infrared (FTIR) spectra were collected using a Bruker Tensor 27 spectrophotometer in the spectral range 4000–400 cm−1 using the KBr disk method. Ellipsometry measurements were conducted using an ellipsometer (Woollam XLS-100) at an angle of 65°/70°/75° and within the spectral range of 300–900 nm at room temperature. An AvaSpec-1024 spectrophotometer with standard mirror optics was used to measure the specular reflectance in the 360–800 nm range at the normal incidence. Evolution of the specular reflectance spectra of the 1DPCs with incident angles was conducted using an ellipsometer (Woollam XLS-100) at an angle of 30°/45°/60° and within the spectral range of 300–900 nm at room temperature.
Results and discussion
Preparation and characterization of 1DPCs
Stable colloidal suspensions of anatase TiO2 and NH2-MIL-88B nanoparticles were synthesized and characterized by XRD, TEM/SEM, and FTIR spectroscopy (Fig. S1–S3†). The as-prepared TiO2 nanoparticles were of high quality in terms of the size and uniformity (particle size of 5 nm to 10 nm). The monodispersed and non-aggregated NH2-MIL-88B nanoparticles were approximately 150 nm long and 50 nm wide. The 1DPCs were obtained by alternating NH2-MIL-88B precursor solution and titania sol by spin-coating. The average RIs between 370 and 780 nm of the NH2-MIL-88B and titania layers were determined by spectroscopic ellipsometry to be 1.045 and 1.806, respectively (Fig. S4 and S5†). The position of the Bragg peak and the reflectivity of the 1DPCs was calculated using the following equations: | λmax = (nLhL + nHhH)sin θ, | (1) |
| 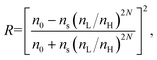 | (2) |
where m is the diffraction order (an integer); λmax is the position of the Bragg peak; nL, hL, nH, and hH are the RIs and physical thicknesses of the two different layers; n0 and ns are RIs of the surrounding environment and substrate, respectively; N is the number of bilayers; and θ is the incident angle. On the basis of the equations, the structural color of the 1DPCs can be tuned by designing the number of layers, incident angles, thickness, and RI of 1DPCs.
Tuning the optical properties of the 1DPCs can be achieved by increasing the number of bilayers (N), as shown in Fig. 1a. The photonic bandwidth becomes narrower and the intensity of the peak grows with increasing numbers of bilayers, which is in good agreement with a previous study, which showed the optical properties of the 1DPCs with respect to the number of bilayers.40Fig. 1b shows the effect of varying incident angles on the optical properties of 1DPCs. With increasing incident angle, the specular reflectance spectra of the 1DPCs shifted toward the shorter wavelength, which is a typical feature of 1DPCs. The observed color changes were from faint red to yellow-green, and then finally to green. Fig. 1b also shows that the reflectivity of the 1DPCs decreases with increasing incident angle, which is in accordance with the experimental results in a previous study.42
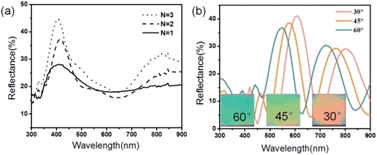 |
| Fig. 1 (a) Evolution of the specular reflectance spectra of the 1DPCs with the number of bilayers. (b) Evolution of the specular reflectance spectra of the 1DPCs with incident angles. Inset images showing corresponding colors of the 1DPCs at different incident angles. | |
The optical response of the 1DPC can also be tuned through the entire visible spectral range by varying the thickness of the NH2-MIL-88B and TiO2 slabs. The thickness of each layer can be readily controlled by manipulating either the concentration of the NH2-MIL-88B precursor solution and titania sol or the spin-coating rate. In these experiments, the thickness of the NH2-MIL-88B layers was controlled by manipulating the concentration of the NH2-MIL-88B precursor solution. Four typical NH2-MIL-88B-based 1DPCs were obtained (Fig. 2a). All the as-prepared 1DPCs displayed uniform colors over a large area with minimal accidental striations and cracks. They exhibited different vivid colors from blue, green, and orange to dark wine. NH2-MIL-88B crystal is an Fe-based MOF and exhibits a dark brown color.
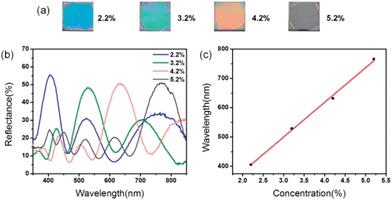 |
| Fig. 2 (a) Images of the 1DPCs fabricated with various concentrations of the NH2-MIL-88B precursor solution; (b) evolution of the specular reflectance spectra with the concentration of the NH2-MIL-88B precursor solution; (c) relationship between the positions of the stop band and the concentration of the NH2-MIL-88B precursor solution. | |
Therefore, a high-quality 1DPC was constructed. Fig. 2b shows the reflectance spectra of the as-prepared 1DPCs and their photonic stop bands centered at 406, 529, 632, and 766 nm. Fig. 2c shows the relationship between the photonic stop band position and NH2-MIL-88B suspension concentration. The Bragg peak was directly proportional to the suspension concentration. The thickness was also linear to the suspension concentration.44 Therefore, the peak shift trend was consistent with the Bragg equation.
The film thickness was measured from the cross-sectional SEM images of the NH2-MIL-88B-based 1DPC, as shown in Fig. 3. TiO2 nanoparticles can partially infiltrate into the NH2-MIL-88B layers because of the small size of the TiO2 nanoparticles (5 nm to 10 nm). Nevertheless, the contrast between the two layers in the 1DPCs is still high enough to show a Bragg peak with a reflectivity of >40% on a silicon substrate. The thickness of the TiO2 layer was estimated to be approximately 40 nm, whereas the thicknesses of the NH2-MIL-88B layers were approximately 124, 181, 237, and 293 nm. The corresponding positions of the photonic stop band were calculated to be 404, 523, 640, and 757 nm, respectively, using eqn (1), which agrees with the experimental results. The slab thickness of TiO2 could also be tailored over a wide range of values, which allowed the position of the stop band to be tuned across the entire visible range.
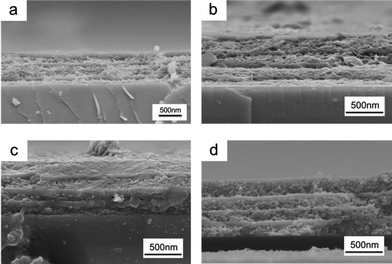 |
| Fig. 3 Cross-section SEM images of 1DPCs fabricated with 4 wt% titania sol and (a) 2.2 wt%, (b) 3.2 wt%, (c) 4.2 wt%, and (d) 5.2 wt% NH2-MIL-88B suspension. | |
Selective response of 1DPCs toward organic vapors
Typical (NH2-MIL-88B/TiO2)4 1DPCs, which were fabricated with 5 wt% NH2-MIL-88B and 3 wt% TiO2 suspension, were used to examine the selective response of various organic vapors by monitoring the visible reflectance spectra. Fig. S6–S11† display the changes in the reflectance spectra of the (NH2-MIL-88B/TiO2)4 1DPCs with time after exposure to various organic vapors, in which significant redshifts of the photonic stop band were observed. The final stable spectra are shown in Fig. 4a. As the solvents varied from DMF to i-PrOH, H2O, MeOH, acetone, and EtOH, the photonic stop band shifted to 8.7, 15.7, 25.1, 36.1, 49.0, and 66.4 nm, respectively (Fig. 4b). The chromaticity coordinates of the 1DPC (Fig. 4c) also changed from CIE: (0.426, 0.414) to (0.447, 0.406), (0.466, 0.391), (0.464, 0.393), (0.468, 0.378), (0.475, 0.366), and (0.467, 0.352). Fig. 4d shows images of the 1DPCs in air and saturated vapors of water and EtOH, which provide a visual verification of the color variations. Conventional porous 1DPCs only show the linear relationship between the reflectance peak and the RI of guests,6,14 whereas (ZIF-8/TiO2)4 1DPC exhibits size-selectivity based on molecular sieving abilities of ZIF-8.34 In this study, however, the selectivity was facilitated by the selective breathing behavior of NH2-MIL-88B. Such a behavior depends on the nature of the organic solvents and their interactions with NH2-MIL-88B (Fig. S12†). The refractive indices of the organic solvents are similar, between 1.33 and 1.48. Therefore, the red shift of the interference peak is mainly induced from the swelling of the NH2-MIL-88B framework. First, the saturated vapor pressure of the solvent is the key factor in the swelling of the framework. In general, the bigger the saturated vapor pressure, the larger the lattice of NH2-MIL-88B expands. Second, apolar solvents induced a small swelling magnitude because of the weak interactions with the organic linker. Third, the distinctive kinetics of breathing for each MIL-88B–solvent association also influences the redshift in the fixed periods. For example, MIL-88B breathes very fast (within a few seconds) in the presence of ethanol, but considerably slower in the presence of water. As a result of the combination of these factors, flexible MOF 1DPCs can selectively sense various vapors.44
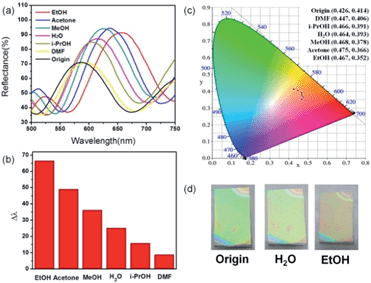 |
| Fig. 4 (a) Reflection spectra; (b) shift of the photonic stop band; (c) chromaticity coordinates; (d) typical images of 1DPCs upon exposure to various organic vapors. | |
Furthermore, the optical response of 1DPC toward a mixture of vapors was investigated to demonstrate its high chemical selectivity. An EtOH and water mixture was selected. The concentration of EtOH was varied by adding 20 μL EtOH each time to 180 μL H2O. The reflectance spectra of 1DPC were monitored. As shown in Fig. 5a, the reflection peak shifted to a longer wavelength with increasing EtOH concentration from 0 vol% to 43.8 vol%. The adsorption of EtOH vapor instead of water vapor results in further expansion of the NH2-MIL-88B crystal, and the photonic stop band correspondingly shifts. In addition, a linear increase in Δλ as a function of the EtOH concentration (0 vol% to 43.8 vol%) was observed (Fig. 5b). When the concentration of EtOH was >43.8 vol%, the reflection peak was almost constant. Quantitative measurements of the EtOH concentration of an aqueous EtOH solution by the direct detection of the mixed vapor of EtOH/H2O generated by the solution are rarely reported.
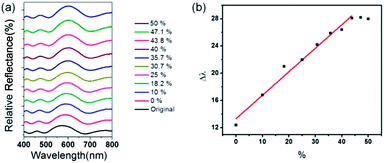 |
| Fig. 5 (a) Reflective spectra of a 4-bilayer 1DPCs with the volume fraction of EtOH in water; (b) relationship between the shift of the stop band and the volume fraction of EtOH in water–EtOH mixture. | |
Stability of the 1DPC sensor
The stability of 1DPCs is another important parameter in an evaluation of the sensing performance for their potential applications and long-term maintenance. The fabricated 1DPCs can retain their color after incubation at 200 °C for 20 min or in air for at least 4 months; such characteristics can be attributed to the perfect stability of the prepared materials and their microstructures (Fig. S13†). Moreover, the mechanical stability was examined by the continuous sonication of 1DPC in water. After sonication (150 W) for 0.5 h, no delamination or peeling occurred on the cross-cut surface, which indicates a strong adherence of the film to the substrate (Fig. S14†). Finally, the recycling stability was further characterized by three baking–detecting cycles. The shift and recovery of reflection peak of the 1DPCs exhibit their high recycling stability (Fig. S15†). The high durability, thermal stability, and strong mechanical stability of the fabricated 1DPCs guarantee the long-term application of the 1DPCs under practical conditions.
In addition, the NH2-MIL-88B photonic film has been reported to selectively respond to varying vapors, in which a pronounced redshift (over 300 nm for EtOH) can be achieved; such a redshift can be attributed to the selectivity and large swelling–shrinkage of NH2-MIL-88B.44 However, the flexible MOF-based 1DPCs fabricated in the current study do not possess such high tunability, probably because the TiO2 nanoparticles are small enough to infiltrate into the voids among the MOF nanoparticles. TiO2 nanoparticles strongly combine the MOFs, which limits the swelling of the flexible MOF nanoparticles. The tenability can potentially be improved by optimizing the size of the TiO2 nanoparticles. Nevertheless, the flexible MOF-based 1DPCs show better mechanical stability compared to the NH2-MIL-88B photonic film and exhibit unique selective behavior. This behavior relies on the distinct interactions between the guests and the flexible MOFs, rather than only the size-selectivity for the conventional porous 1DPCs.
Conclusion
Flexible MOF-based 1DPCs with vivid structural colors were fabricated by alternate spin-coating of a NH2-MIL-88B solution and titania sol. The optical properties of the 1DPCs could be tuned by varying the number of bilayers, incident angles, and physical thickness of the individual slabs. The fabricated 1DPCs can be used as a selective sensing platform, which benefits from the selective breathing response of the flexible MOFs toward guest molecules. The selective quantitative measurement of EtOH can also be realized from the mixture of EtOH and water because the shift of photonic stop band linearly increases with increasing EtOH concentration (0 vol% to 43.8 vol%). The fabricated 1DPCs exhibit exceptional stability in a high temperature, ultrasonic bath, and long-term storage. Given that the unique characteristic of the MOFs elicited the rapid increase in reports of post-synthetic modification, the proposed 1DPCs, which combine the advantages of conventional nanoparticle-based 1DPCs (high porosity and thermal stability) and organic/hybrid organic–inorganic 1DPCs (large swelling and easy modification), are expected to play an increasingly important role in the development of 1DPCs.
Acknowledgements
This work was supported by the National Natural Science Foundation of China (21203247, 61171020) and Research Project of National University of Defense Technology (JC12-02-12). We thank Dr Tianliang Qu for the help with Ellipsometry measurements.
Notes and references
- G. von Freymann, V. Kitaev, B. V. Lotsch and G. A. Ozin, Chem. Soc. Rev., 2013, 42, 2528 RSC.
- C. Fenzl, T. Hirsch and O. S. Wolfbeis, Angew. Chem., Int. Ed., 2014, 53, 3318 CrossRef CAS PubMed.
- H. Xu, P. Wu, C. Zhu, A. Elbaz and Z. Z. Gu, J. Mater. Chem. C, 2013, 1, 6087 RSC.
- Z. Wu, D. Lee, M. F. Rubner and R. E. Cohen, Small, 2007, 3, 1445 CrossRef CAS PubMed.
- I. Pavlichenko, A. T. Exner, M. Guehl, P. Lugli, G. Scarpa and B. V. Lotsch, J. Phys. Chem. C, 2011, 116, 298 Search PubMed.
- L. González-García, G. Lozano, A. Barranco, H. Míguez and A. R. González-Elipe, J. Mater. Chem., 2010, 20, 6408–6412 RSC.
- M. N. Ghazzal, M. Joseph, H. Kebaili, J. De Coninck and E. M. Gaigneaux, J. Mater. Chem., 2012, 22, 22526 RSC.
- S. Y. Choi, M. Mamak, G. von Freymann, N. Chopra and G. A. Ozin, Nano Lett., 2006, 6, 2456 CrossRef CAS PubMed.
- M. zbarhoum, J. M. Morrill, D. Riassetto and M. H. Bartl, Chem. Mater., 2011, 23, 5177 CrossRef.
- M. N. Ghazzal, O. Deparis, A. Errachid, H. Kebaili, P. Simonis, P. Eloy, J. P. Vigneron, J. De Coninck and E. M. Gaigneaux, J. Mater. Chem., 2012, 22, 25302 RSC.
- L. Tosheva and V. P. Valtchev, Chem. Mater., 2005, 17, 2494 CrossRef CAS.
- B. V. Lotsch and G. A. Ozin, ACS Nano, 2008, 2, 2065 CrossRef CAS PubMed.
- B. V. Lotsch and G. A. Ozin, Adv. Mater., 2008, 20, 4079–4084 CrossRef CAS.
- Y. Dou, J. Han, T. Wang, M. Wei, D. G. Evans and X. Duan, J. Mater. Chem., 2012, 22, 14001 RSC.
- J. Ge, L. He, Y. Hu and Y. Yin, Nanoscale, 2011, 3, 177 RSC.
- W. Luo, H. Ma, F. Mou, M. Zhu, J. Yan and J. Guan, Adv. Mater., 2014, 26, 1058 CrossRef CAS PubMed.
- D. J. Mulder, A. P. H. J. Schenning and C. W. M. Bastiaansen, J. Mater. Chem. C, 2014, 2, 6695–6705 RSC.
- Y. Jiang, D. Xu, X. Li, C. Lin, W. Li, Q. An, C. Tao, H. Tang and G. Li, J. Mater. Chem., 2012, 22, 11943 RSC.
- Z. Wang, J. Zhang, J. Li, J. Xie, Y. Li, S. Liang, Z. Tian, C. Li, Z. Wang, T. Wang, H. Zhang and B. Yang, J. Mater. Chem., 2011, 21, 1264 RSC.
- C. Liu, C. Yao, Y. Zhu, J. Ren, K. Lan, H. Peng and L. Ge, RSC Adv., 2014, 4, 27281 RSC.
- M. Karaman, S. E. Kooi and K. K. Gleason, Chem. Mater., 2008, 20, 2262 CrossRef CAS.
- Y. Kang, J. J. Walish, T. Gorishnyy and E. L. Thomas, Nat. Mater., 2007, 6, 957 CrossRef CAS PubMed.
- L. Zhai, A. J. Nolte, R. E. Cohen and M. F. Rubner, Macromolecules, 2004, 37, 6113 CrossRef CAS.
- R. Jakubiak, T. J. Bunning, R. A. Vaia, L. V. Natarajan and V. P. Tondiglia, Adv. Mater., 2003, 15, 241 CrossRef CAS.
- V. K. S. Hsiao, W. D. Kirkey, F. Chen, A. N. Cartwright, P. N. Prasad and T. J. Bunning, Adv. Mater., 2005, 17, 2211 CrossRef CAS.
- J. Shi, V. K. S. Hsiao, T. R. Walker and T. J. Huang, Sens. Actuators, B, 2008, 129, 391 CrossRef CAS PubMed.
- T. Kazmierczak, H. Song, A. Hiltner and E. Baer, Macromol. Rapid Commun., 2007, 28, 2210 CrossRef CAS.
- S. T. Meek, J. A. Greathouse and M. D. Allendorf, Adv. Mater., 2011, 23, 249 CrossRef CAS PubMed.
- C. Serre, C. Mellot-Draznieks, S. Surblé, N. Audebrand, Y. Filinchuk and G. Férey, Science, 2007, 315, 1828 CrossRef CAS PubMed.
- L. E. Kreno, K. Leong, O. K. Farha, M. Allendorf, R. P. Van Duyne and J. T. Hupp, Chem. Rev., 2011, 112, 1105 CrossRef PubMed.
- G. Lu and J. T. Hupp, J. Am. Chem. Soc., 2010, 132, 7832 CrossRef CAS PubMed.
- Y.-n. Wu, F. Li, W. Zhu, J. Cui, C. Tao, C. Lin, P. M. Hannam and G. Li, Angew. Chem., Int. Ed., 2011, 50, 12518 CrossRef CAS PubMed.
- Y.-n. Wu, F. Li, Y. Xu, W. Zhu, C. Tao, J. Cui and G. Li, Chem. Commun., 2011, 47, 10094 RSC.
- F. M. Hinterholzinger, A. Ranft, J. M. Feckl, B. Ruhle, T. Bein and B. V. Lotsch, J. Mater. Chem., 2012, 22, 10356 RSC.
- G. Ferey and C. Serre, Chem. Soc. Rev., 2009, 38, 1380 RSC.
- K. Barthelet, J. Marrot, D. Riou and G. Férey, Angew. Chem., Int. Ed., 2002, 41, 281 CrossRef CAS.
- C. Serre, F. Millange, C. Thouvenot, M. Noguès, G. Marsolier, D. Louër and G. Férey, J. Am. Chem. Soc., 2002, 124, 13519 CrossRef CAS PubMed.
- A. C. McKinlay, J. F. Eubank, S. Wuttke, B. Xiao, P. S. Wheatley, P. Bazin, J. C. Lavalley, M. Daturi, A. Vimont, G. De Weireld, P. Horcajada, C. Serre and R. E. Morris, Chem. Mater., 2013, 25, 1592 CrossRef CAS.
- N. A. Ramsahye, T. K. Trung, L. Scott, F. Nouar, T. Devic, P. Horcajada, E. Magnier, O. David, C. Serre and P. Trens, Chem. Mater., 2013, 25, 479 CrossRef CAS.
- P. Horcajada, F. Salles, S. Wuttke, T. Devic, D. Heurtaux, G. Maurin, A. Vimont, M. Daturi, O. David, E. Magnier, N. Stock, Y. Filinchuk, D. Popov, C. Riekel, G. Férey and C. Serre, J. Am. Chem. Soc., 2011, 133, 17839 CrossRef CAS PubMed.
- M. Ma, A. Bétard, I. Weber, N. S. Al-Hokbany, R. A. Fischer and N. Metzler-Nolte, Cryst. Growth Des., 2013, 13, 2286 CAS.
- Z. Wang, J. Zhang, J. Xie, C. Li, Y. Li, S. Liang, Z. Tian, T. Wang, H. Zhang, H. Li, W. Xu and B. Yang, Adv. Funct. Mater., 2010, 20, 3784 CrossRef CAS.
- M.-H. Pham, G.-T. Vuong, A.-T. Vu and T.-O. Do, Langmuir, 2011, 27, 15261 CrossRef CAS PubMed.
- Z. Hu, C. Tao, H. Liu, X. Zou, H. Zhu and J. Wang, J. Mater. Chem. A, 2014, 2, 14222 CAS.
Footnotes |
† Electronic supplementary information (ESI) available: Characterization of NH2-MIL-88B and TiO2 nanoparticles, optical responses of 1DPC upon various organic vapors, photographs of 1DPC after high thermal, long term and ultrasonic treatments. See DOI: 10.1039/c4tc01501k |
‡ These authors equally contributed to this work. |
|
This journal is © The Royal Society of Chemistry 2015 |
Click here to see how this site uses Cookies. View our privacy policy here.