DOI:
10.1039/C5AN01843A
(Paper)
Analyst, 2016,
141, 270-278
Indicator displacement assay for cholesterol electrochemical sensing using a calix[6]arene functionalized graphene-modified electrode†
Received
7th September 2015
, Accepted 15th November 2015
First published on 16th November 2015
Abstract
A novel electrochemical method has been developed towards cholesterol detection based on competitive host–guest interaction by selecting methylene blue (MB) and calix[6]arene functionalized graphene (CX6–Gra) as the “reporter pair”. In the presence of cholesterol, the MB molecules are displaced by cholesterol in the CX6–Gra.MB complex, leading to a “switch off” electrochemical response. A linear response range of 0.50 to 50.00 μM for cholesterol with a low detection limit of 0.20 μM (S/N = 3) was obtained by using the proposed method. This method could be successfully utilized to detect cholesterol in serum samples, and may be expanded to the analysis of other non-electroactive species. Besides, the host–guest interaction between cholesterol and CX6 was studied by molecular modeling calculations, which revealed that the complexation could reduce the energy of the system and the complex of a 1
:
1 host–guest stoichiometry had the lowest binding free energy of −8.01 kcal mol−1. In addition, the constructed electrochemical sensing platform is important as it does not use any enzyme or antibody for the detection of cholesterol efficiently and selectively over common interfering species.
Introduction
The concept of indicator displacement assay (IDA) has received considerable interest with the development of supramolecular chemistry, which exploits the potential of artificial receptors, particularly macrocyclic hosts, for its promising applications in molecular recognition and sensing.1,2 The sensing principle of IDA relies on the competition between a test substance and an indicator for the same binding site on the host.3 When an analyte is added to a solution containing a host·indicator complex, the analyte displaces the indicator from the binding site. Upon displacement of the indicator, a change in signal is observed. Although IDA has been widely applied in the fluorescence sensing field,4–10 it is rarely investigated for electrochemical sensing applications except for a few researchers that contributed to this area by using natural β-cyclodextrin as the macrocyclic host.11–13
Calixarenes, recognised as the third class of macrocyclic host molecule after crown ethers and cyclodextrins, have become important receptors because they can form stable host–guest complexes with various organic, inorganic, and biological guest molecules, which show high supramolecular recognition and enrichment capability.14,15 Water-soluble calixarenes, particularly p-sulfonated derivatives, have been widely investigated to develop different electrochemical sensing platforms and separation matrices due to their biocompatibility and simplicity of synthesis.10,16 Graphene is a material that holds great promise for potential applications in many technological fields because of its extraordinary thermal, mechanical, and electrical properties.17 However, the preparation of soluble graphene is challenging as graphene is known to have poor solubility.18 The advantage of water-soluble calixarenes as well as cyclodextrin functionalization is that they offer high water solubility to graphene, and guest molecules incorporated into calixarenes are easily accessible to graphene.16 It has been reported that the composites of calixarenes and carbon materials (e.g. carbon nanotubes, graphene) could be formed by π–π interactions and hydrogen interactions.16,19–21 If graphene is modified with water-soluble calixarenes, it is likely to form new materials simultaneously possessing a large surface area and good conductivity of graphene, and high supramolecular recognition and enrichment capability of calixarenes. Therefore, the integration of graphene and water-soluble calixarenes can be potentially applied in the field of electrochemical sensing or biosensing, and thus arouses extensive research interest.
Cholesterol is a vital component in cells and tissues of humans, playing a functional role in the construction of cell membranes or serving as a biosynthetic precursor of bile acids, vitamin D, steroid hormones, etc.22 The normal level of total cholesterol in healthy human serum is ∼200 mg dL−1.23 Excess cholesterol in blood serum forms plaques in the arteries of blood vessels which prevent blood circulation and cause cardiovascular diseases.9 Thus the levels of total cholesterol in serum and food are major parameters for diagnostic treatment. Herein, a sensitive and selective electrochemical approach for cholesterol sensing based on a competitive host–guest recognition between CX6 Scheme 1(a) and signal probe/target molecules using a CX6–Gra modified electrode was developed for the first time. Methylene blue (MB) and cholesterol were selected as the probe and target molecules, respectively. Due to the host–guest interaction, MB molecules can enter into the hydrophobic inner cavity of CX6 Scheme 1(a), and the CX6–Gra modified glassy carbon electrode displays a remarkable cathodic peak. In the presence of cholesterol, a competitive interaction to CX6 occurs and the MB molecules are displaced by cholesterol Scheme 1(b). This results in a decreased reduction peak current of MB (Scheme 1). MB is a well-known redox probe and hence can be easily detected using the differential pulse voltammetry (DPV) technique.
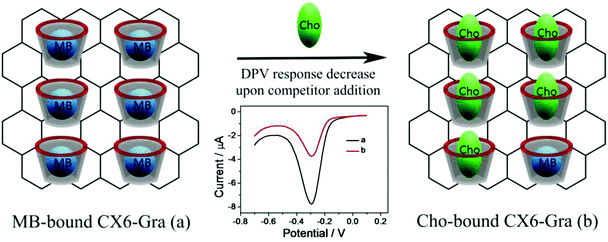 |
| Scheme 1 Competitive host–guest molecular recognition of cholesterol (Cho) using CX6–Gra against MB. | |
Materials and methods
Chemicals and materials
Graphite oxide was purchased from Nanjing XFNANO Materials Tech Co., Ltd (Nanjing, China). 4-Sulfocalix[6]arene hydrate (CX6) was obtained from Tokyo Chemical Industry Co., Ltd (Tokyo, Japan). Corticosterone, estrone, β-estradiol, and β-sitosterol were obtained from Shanghai Adamas Reagent Co., Ltd (Shanghai, China). All other reagents were of analytical grade. Phosphate buffer (PBS, 0.1 M, pH 7.0) was prepared by mixing stock solutions of 0.1 M KH2PO4 and K2HPO4 and was used as the working solution. All aqueous solutions were prepared with deionized water (DW, 18 MΩ cm).
Apparatus
Differential pulse voltammetry (DPV) and electrochemical impedance spectroscopy (EIS) experiments were performed with a CHI 660E Electrochemical Workstation from Shanghai Chenhua Instrument (Shanghai, China) and conducted using a three-electrode system, with the modified GCE as the working electrode, a platinum wire as the counter electrode, and a saturated calomel electrode (SCE) as the reference electrode. The morphology of the prepared sample was characterized by QUNT200 scanning electron microscopy (SEM, Hillsboro, Oregon, USA) and JEM 2100 transmission electron microscopy (TEM, Tokyo, Japan). UV-visible spectra were analyzed using a U-2001 Hitachi (Tokyo, Japan) UV spectrophotometer. Raman spectra were obtained on a 400F PERKINELMER Raman spectrometer (Shelton, USA) with a 514.5 nm wavelength incident laser light. Fluorescence titration experiments were carried out using an Hitachi F-4500 fluorescence spectrophotometer (Tokyo, Japan) at room temperature. A fourier transform infrared (FTIR) study was performed over the wavenumber range of 4000–400 cm−1 using a Thermo Fisher SCIENTIFIC Nicolet IS10 (Massachusetts, USA) FTIR impact 410 spectrophotometer using KBr pellets. Thermogravimetric analysis (TGA) was carried out using Q50 TGA (TA Instruments, New Castle, Delaware, USA), from 25 to 800 °C at a heating rate of 5 °C min−1 in argon.
Absorbance and fluorescence titration experiments
A stock solution of MB (500 μM) and a stock solution of CX6 (500 μM) in 0.1 M PBS (pH 7.0) were prepared. As cholesterol has very low solubility in water, a 500 μM stock solution of cholesterol was prepared in ethanol. The dye stock solution was diluted with 0.1 M PBS (pH 7.0) to a final concentration of 10 μM. CX6 was then gradually added into the MB solution and mixed by vortexing well for 5 min before the absorbance or fluorescence was recorded. For the competitive displacement of MB from the CX6 by cholesterol, the required amount of cholesterol was gradually added into the mixture of CX6 and MB and mixed by vortexing well for 5 min before the absorbance or fluorescence was recorded.
Molecular docking
The crystal structures of 4-sulfocalix[6]arene hydrate (CX6) (ID: FEQYOQ) and cholesterol (ID: CHOEST21) were obtained from the Cambridge Crystallographic Data Centre (CCDC) and optimized using molecular dynamics simulation with the Gaussian 03 program. Both the optimized structures were used as a starting structure in the docking study. AutoDock4.2 with the Lamarckian Genetic Algorithm (LGA) was used for the docking study. An initial population of 150 individuals with a maximum number of energy evaluations of 25
000
000 and a maximal number of generations of 27
000 were used as an end criterion. An elitism value of one was used and a probability of mutation and crossing-over of 0.02 and 0.8 were used, respectively. We have defined the conformational search space implementing an 60 × 60 × 60 grid and 0.375 Å spacing between each point in such a way that it covered both the external surface and the internal cavity of CX6. A total of 50 docking runs were carried out. At the end of each run, the solutions were separated into clusters according to their lowest RMSD and the best energy score value based on an empirical free energy function. Clustering was performed on the docked complexes with a cut-off of 2 Å. From the docking calculations, the lowest energy conformation was selected as the cholesterol/CX6 binding mode, and the binding free energy of the cholesterol/CX6 complex was calculated using the semi-empirical method PM3.
Preparation of CX6–Gra
The graphite oxide was exfoliated into graphene oxide (GO) sheets by ultrasonication at room temperature for 1 h. The as-obtained yellow-brown aqueous suspension of GO was stored at room temperature and used for further experiment. Compared with the traditional procedure using highly toxic hydrazine as reductant, glucose was used as the reducing agent to reduce GO in DW. In a typical experiment, 20.0 mL of a 0.5 mg mL−1 GO aqueous suspension was mixed with 20.0 mL of a 1.0 mg mL−1 CX6 aqueous solution and the mixture was stirred at room temperature for 12 h. Then, 200.0 μL of ammonia solution and 50.0 mg of glucose were added into the mixture. After being vigorously shaken or stirred for a few minutes, the mixture was stirred at 95 °C for 60 min. After cooling to room temperature, the resulting stable black dispersion was centrifuged at 16
000 rpm and washed with DW 3 times. Finally, the resulting CX6–Gra material was obtained by freeze-drying. Additionally, Gra was prepared with a similar procedure in the absence of CX6.
Preparation of the modified electrodes
A glassy carbon electrode (GCE, 3 mm in diameter) was polished with 0.3 and 0.05 μm Al2O3 powder respectively and subsequently sonicated in ethanol and DW to remove the adsorbed substance and dried in air. The CX6–Gra was dissolved in DW at a concentration of 1.0 mg mL−1 with the aid of ultrasonic agitation for 20 min, resulting in a homogeneous suspension. To prepare the CX6–Gra modified electrode, 5 μL of the Gra suspension was dropped onto the electrode surface and dried at room temperature. The obtained electrode was noted as the CX6–Gra/GC electrode.
Electrochemical measurements
DPV was applied in 0.1 M PBS (pH 7.0) from 0.1 to −0.7 V with a pulse amplitude of 0.05 V and a pulse width of 0.05 s. EIS was recorded in the frequency range from 10−1 to 105 Hz with an amplitude of 5 mV using a 2.0 mM [Fe(CN)6]3−/4− redox couple (1
:
1) with 0.1 M KCl as the supporting electrolyte. All the measurements were carried out at room temperature. As cholesterol has very low solubility in water, 1.0 mM stock solution of cholesterol was prepared in ethanol and diluted to different concentrations by 0.1 M PBS (pH 7.0) for further use. Before electrochemical measurements, the CX6–Gra/GCE was incubated with 100 μM MB solution (in 0.1 M PBS, pH 7.0) for 30 min, and rinsed gently with DW. Then, the electrode was further incubated with different concentrations of the cholesterol solution for 30 min. After that, the electrode was rinsed gently with DW and the current response of the MB-bound CX6–Gra/GCE was investigated by DPV in 0.1 M PBS (pH 7.0).
Cholesterol detection in serum
Cholesterol detection in serum was performed using human serum. A stock solution of cholesterol (1.0 mM) was prepared in ethanol and diluted to different concentrations by 0.1 M PBS (pH 7.0) for further use. The serum sample was diluted 50 times with 0.1 M PBS (pH 7.0) and mixed with a known amount of cholesterol. Next, this solution was used to detect cholesterol according to the procedure described above.
Results and discussion
Absorbance and fluorescence spectra analysis
The absorption spectra of MB in the presence of various concentrations of CX6 were investigated. As shown in Fig. 1A, MB exists as a monomer/dimer equilibrium in 0.1 M PBS at pH 7.0. The absorption spectrum of MB shows a band for the monomer at 654 nm, and the band for the dimer is at 605 nm. Upon successive addition of CX6 (up to 20 μM), the monomer absorption band shows a systematic decrease. The dimer band decreased to reach a plateau value at a high CX6 concentration. This indicated that CX6 weakened the absorbance of the MB monomer by the formation of the inclusion complex and suppressed the dimer formation. Interestingly, the addition of cholesterol to the mixture of CX6 and MB led to a successive reversion of the monomer absorption band and a significant decrease of the dimer band (Fig. 1B). This may be attributed to the displacement of MB by cholesterol from the CX6 host. Fig. 1C shows the fluorescence titrations of MB (10 μM, λex = 640 nm) upon successive addition of CX6 (up to 2.5 μM) in 0.1 M PBS at pH 7.0. The addition of CX6 caused quenching of the fluorescence intensities of the MB solutions. In contrast, a typical displacement titration is depicted in Fig. 1D, where the addition of cholesterol reverts the fluorescence changes originally caused by the addition of CX6. The competitive fluorescence titrations confirmed the displacement of MB by cholesterol from the CX6 host.
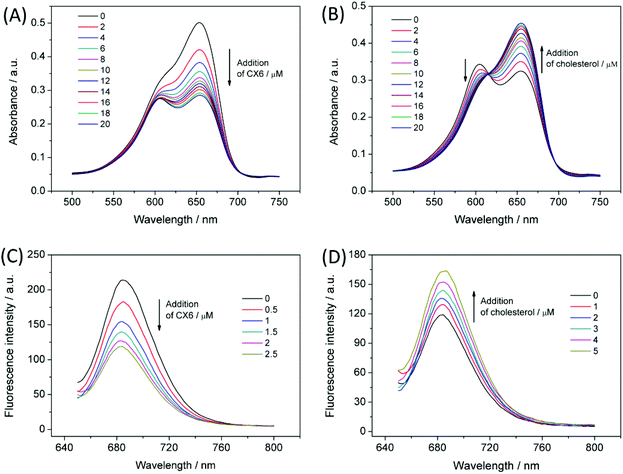 |
| Fig. 1 (A) Absorbance spectra of MB (10 μM) upon successive addition of CX6 (up to 20 μM) in 0.1 M PBS at pH 7.0; (B) absorbance spectra for the competitive displacement of MB (10 μM) from CX6 (20 μM) by cholesterol (up to 20 μM) in 0.1 M PBS at pH 7.0; (C) fluorescence titrations of MB (10 μM, λex = 640 nm) upon successive addition of CX6 (up to 2.5 μM) in 0.1 M PBS at pH 7.0; (D) fluorescence titration for the competitive displacement of MB (10 μM) from CX6 (2.5 μM) by cholesterol (up to 5.0 μM) in 0.1 M PBS at pH 7.0. | |
The mechanism of the competitive host–guest interaction
A double reciprocal plot of 1/(F0 − F) versus 1/[CX6] for MB to CX6 was obtained (Fig. S1†), indicating the existence of a 1
:
1 complex.5 From the plots the binding constant (K) for the 1
:
1 MB/CX6 complex was calculated to be 3.05 × 105 M−1. It was difficult to obtain the K value of the cholesterol/CX6 complex using the same method due to the negligible change of fluorescence intensity because cholesterol itself has no fluorescence. In order to rationalize our experimental data and to infer the inclusion pattern, molecular docking was performed to study the CX6/cholesterol inclusion complex. Typically, the more negative the binding energy is, the stronger the interaction is between the host and guest molecules. As listed in Table S1,† the lowest binding free energy (ΔG) was −8.01 kcal mol−1 for the host–guest complex of cholesterol and CX6 with a 1
:
1 stoichiometry calculated by the PM3 method. The K value of the cholesterol/CX6 complex could be estimated to be 7.50 × 106 M−1 from the following equation: ΔG = −RT
ln
K, where R is the gas constant and T is the experimental temperature. The K value of the cholesterol/CX6 complex was more than 20 times greater than that of MB/CX6, which demonstrated the stronger binding of cholesterol with CX6 than that with MB. The lowest energy docked conformation for the 1
:
1 complex of cholesterol and CX6, shown in Fig. 2A, reveals the partial inclusion of the cholesterol molecule in the hydrophobic cavity of CX6. The cyclohexanol part and the alkyl chain of the cholesterol molecule inserted into the cavity of the CX6 host molecule. Analysis of the host–guest interaction as obtained from the docking studies reveals that hydrogen bonding, electrostatic interactions, and hydrophobic interactions are the predominant driving forces of the host–guest complex formation. Firstly, the hydroxyl on cyclohexanol of the cholesterol molecule formed hydrogen bonds with the –SO3− of CX6 and the bond length is 2.0 Å. Secondly, as shown in Fig. 2B, strong electrostatic interactions form between the positive part of the cholesterol molecule and the negative –SO3− of CX6. Thirdly, as revealed in Fig. 2C, strong hydrophobic interactions also form between the hydrophobic alkyl chain of the cholesterol molecule and CX6.
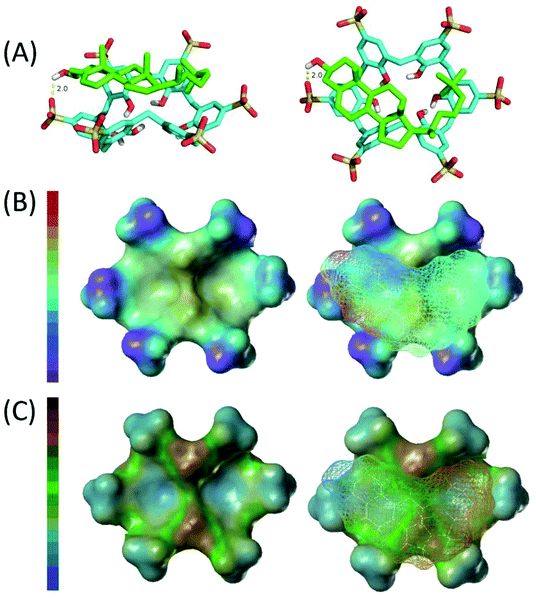 |
| Fig. 2 (A) Lowest energy cholesterol/CX6 docked complex for a 1 : 1 host–guest stoichiometry (left is the side view, right is the top view); (B) the electrostatic forces (left is the top view of CX6, right is the top view of the cholesterol/CX6 complex, red represents the strongest positive charge, and blue represents the strongest negative charge); (C) the hydrophobic forces (left is the top view of CX6, right is the top view of the cholesterol/CX6 complex, brown represents the strongest hydrophobic interaction, and blue represents the strongest hydrophilic interaction) of the cholesterol/CX6 docked complex for a 1 : 1 host–guest stoichiometry. | |
Characterization of the CX6–Gra composite
The CX6–Gra was prepared via a one-pot wet-chemical strategy based on glucose reduction of graphene oxide in the presence of CX6. The dispersibility of Gra and CX6–Gra was investigated as shown in Fig. S2.† Although GO is highly water soluble when it is chemically converted into Gra via glucose reduction, it forms agglomerated Gra due to the strong π–π stacking interaction between Gra sheets and there is a decrease of the surface hydrophilic groups originally present in GO. However, when the GO reduction is performed in the presence of CX6, Gra becomes highly water-soluble even after removing the free CX6 via high-speed centrifugation (16
000 rpm, 3 times) and no obvious precipitates are observed after being stored for more than 3 months.
The synthesized Gra and CX6–Gra materials were characterized by UV-vis spectroscopy. As shown in Fig. S3,† GO shows a strong absorption at 230 nm and a shoulder at 300 nm, which corresponds to the π–π* transition of the aromatic C
C bond and the n–π* transition of the C
O bond, respectively. After reduction, the peak at 230 nm gradually red-shifts to 260 nm, suggesting that the electronic conjugation within the Gra sheets is restored upon glucose reduction.10,24,25
Raman spectroscopy is one of the most widely used techniques to characterize the structural and electronic properties of Gra including disordered and defective structures, defect density, and doping levels. Fig. S4† shows the typical Raman spectra of GO, Gra and CX6–Gra. As expected, GO displays two prominent peaks at 1340 and 1584 cm−1 corresponding to the D and G bands, respectively. Gra shows two prominent peaks at 1347 and 1586 cm−1, corresponding to the breathing mode of the k-point phonons of A1g symmetry (D band) and the E2g phonons of the C sp2 atoms (G band) of Gra, respectively. The intensity ratio of the D band to the G band (ID/IG) is clearly higher when compared with that of GO (0.98 vs. 0.77), suggesting a decrease in sp2 domains and a partially ordered crystal structure of Gra induced by glucose reduction. The Raman spectrum of CX6–Gra was similar to that of Gra, indicating that the crystal structure of Gra is not severely affected by CX6.
The microstructure of CX6–Gra was characterized by SEM (Fig. S5†) and TEM (Fig. S6†) observation. The SEM and TEM images reveal that the CX6–Gra material consists of randomly aggregated thin, wrinkled sheets closely associated with each other. Since it is difficult to distinguish the CX6 molecules in the SEM and TEM images, the Gra and CX6–Gra materials were further characterized by FTIR and TGA analysis.
The synthesized Gra and CX6–Gra materials were characterized by FTIR as shown in Fig. S7.† By comparing the FTIR spectra of CX6, CX6–Gra, and Gra, significant features can be observed: firstly, the peaks for –SO3− at 1160 and 1040 cm−1, as seen in the spectrum of pure CX6, also appeared in the spectrum of CX6–Gra, indicating that CX6 was attached to the surface of Gra.16 Secondly, the alteration of the peak value at 3391 cm−1 of –OH in the CX6 stretching vibrations shifting to 3360 cm−1 in CX6–Gra was identified as a result of hydrogen interactions between the remaining oxygen-containing groups of Gra and the hydroxyl groups of CX6.10 In addition, it has been reported that the composites of calixarenes and carbon materials (e.g. carbon nanotubes, Gra) could be formed by π–π interactions and hydrogen interactions.16,19–21 These results demonstrated that CX6 had successfully self-assembled to Gra and formed a CX6–Gra nanocomposite.
The prepared CX6–Gra and the related materials were also characterized by TGA, as shown in Fig. S8.† The GO curve shows a small mass loss (20%) at approximately 160 °C and a major mass loss (50%) at approximately 300 °C due to the loss of adsorbed water and pyrolysis of the labile oxygen-containing functional groups, respectively. In contrast, Gra is more thermally stable than GO. After reduction, the mass loss is 24% compared to the 62% mass loss at 600 °C for GO. For the pristine Gra, the minor loss in mass (24%) at a temperature of approximately 600 °C was due to the pyrolysis of a very small amount of the remaining oxygen-containing functional groups. The CX6–Gra material exhibited an abrupt mass loss when the temperature was approximately 450 °C because of the decomposition of CX6; the mass loss reached about 42 wt% when the temperature was 600 °C. The amount of CX6 molecules grafted to Gra was estimated to be 18.0 wt%. This result was in accordance with a previous study.16
Design strategy of the electrochemical sensor
The design strategy of the proposed electrochemical sensor based on the competitive host–guest interaction between CX6 and MB (signal probe)/cholesterol (target) is illustrated in Scheme 1. MB molecules can enter into the inner cavity of CX6 due to the host–guest interaction, and the MB-bound CX6–Gra/GCE displays a remarkable reduction peak due to MB. However, in the presence of cholesterol, competitive association to CX6 occurs and the MB molecules are displaced by cholesterol molecules. This results in a decrease of the reduction peak current of the MB probe.
Feasibility of the electrochemical sensor
To demonstrate the assay feasibility of the proposed competitive electrochemical sensing platform, the DPV response of the CX6–Gra/GCE was investigated in 0.1 M pH 7.0 PBS. As can be seen from Fig. 4A, no detectable signal (curve a) is observed for the CX6–Gra/GCE in 0.1 M pH 7.0 PBS due to the absence of the redox mediator MB. After incubation in 100 μM MB solution for 30 min, the MB-bound CX6–Gra/GCE was then tested in 0.1 M pH 7.0 PBS and an obvious reduction peak of MB (curve b) can be observed at about −0.3 V. When the CX6–Gra/GCE was first incubated in 100 μM MB solution for 30 min and further incubated in 25 μM cholesterol solution for 30 min, then tested in 0.1 M pH 7.0 PBS, a decreased reduction peak (curve c) was obtained due to competitive association of cholesterol/MB to CX6. This is because cholesterol has a higher binding affinity to the CX6 cavity due to its hydrophobic nature. This suggests that the MB molecules present inside the CX6–Gra/GCE host can be replaced by cholesterol and the MB-bound CX6–Gra/GCE can be used to sensitively detect cholesterol by the competitive electrochemical sensing strategy shown in Scheme 1.
Electrochemical characterization of the modified electrodes
EIS was performed at a potential of 0.1 V and the frequency range was from 10−1 to 105 Hz, using a 2.0 mM [Fe(CN)6]3−/4− redox couple (1
:
1) with 0.1 M KCl as the supporting electrolyte. The value of the charge transfer resistance (Rct) of the modified electrode was estimated by the semicircle diameter. Fig. S9† illustrates the EIS of the bare GCE, Gra/GCE, and CX6–Gra/GCE. Obviously, the bare GCE exhibited a semicircle portion and the value of Rct was estimated to be approximately 800 Ω. Meanwhile the Rct value decreased dramatically, nearly to zero, for the Gra/GCE, indicating that the Gra/GCE formed high electron conduction pathways between the electrode and electrolyte, and had good conductivity and obviously improved the diffusion of ferricyanide toward the electrode interface. When the CX6–Gra modified the bare GCE, the semicircle increased to 1500 Ω; this is because the CX6 layer hindered the electron transfer and made the interfacial charge transfer difficult, suggesting that CX6 molecules were successfully immobilized on the surface of Gra. This result is in accordance with that of the TGA mass loss. A modified Randle's equivalent circuit is provided in Fig. 3 (inset). The impedance data were fitted with commercial software Zview2. The fitting curves obtained by the equivalent circuit are depicted in Fig. 3. A good fit was obtained with the model used for all experimental data. The semicircle portion (Rct), observed at higher frequencies in Fig. 3, corresponds to the electron transfer-limited process, whereas the linear part is characteristic of the lower frequency range and represents the diffusion-limited electron transfer process. The simulated values of the equivalent circuit elements are summarized in Table 1, where they show that the change of Rct was rather significant. The changes in Rct were much larger than those in other impedance elements from Table 1, therefore, the Rct value was considered as a suitable signal for expressing the interfacial properties of the as-prepared electrode. A constant phase element (CPE) was used instead of the classical capacitance to fit the impedance data. Rs was the ohmic resistance of the electrolyte solution. Zw was the Warburg impedance, resulting from the diffusion of ions from the electrolyte to the electrode interface.26
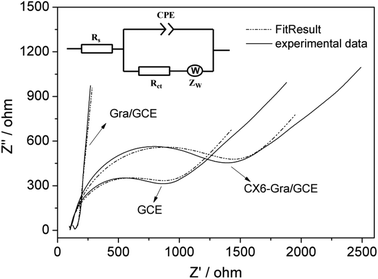 |
| Fig. 3 Nyquist diagram of the fitting curves compared with the experimental results. Inset: Randle's equivalent circuit corresponding to the impedance features. | |
Table 1 Simulated values of the equivalent circuit elements for the bare GC, Gra/GC, and CX6–Gra/GC electrodes
Electrode |
R
s (Ω) |
CPE (F) |
R
ct (Ω) |
Z
w (Ω) |
GCE |
101.4 |
2.5 × 10−6 |
805 |
0.28 |
Gra/GCE |
132.0 |
1.1 × 10−6 |
96.3 |
0.32 |
CX6–Gra/GCE |
102.8 |
3.4 × 10−6 |
1495 |
0.31 |
Quantitative analysis of cholesterol
Under optimal conditions (the incubation time of the CX6–Gra/GCE in MB solution and the MB-bound CX6–Gra/GCE in cholesterol solution was studied, which is provided in the ESI†), DPV was used to determine the concentrations of cholesterol because it is a highly sensitive and low detection limit electrochemical method. Fig. 4B shows the DPV curves of the electrochemical signal on the MB-bound CX6–Gra/GCE under different concentrations of cholesterol solution. The reduction peak currents of MB decreased with the increased cholesterol concentrations. Above 50 μM, the signal reached its saturation. Here, the remaining signal should be that of the non-specific adsorption of MB caused by the π–π interactions between MB and Gra. However, these will not make a difference to the DPV results, as MB remains intact on the surface of Gra in the beginning as well as at the end of the experiment. A control experiment was carried out to exclude the possibility of desorption of MB from the Gra surface during cholesterol detection (Fig. S12†). Fig. 4C shows the corresponding calibration curve for cholesterol quantification. The peak current changes were proportional to the cholesterol concentrations between 0.50 and 50.00 μM with a detection limit of 0.20 μM (S/N = 3). The corresponding regression equation was calculated as ΔI (μA) = 0.099C (μM) + 0.469 with a correlation coefficient of 0.996. The sensitivity was obtained from the slope of the calibration plot and was 0.099 μA μM−1. The detection limit was less than 1.0 μM which was quite low and satisfactory with respect to other recently reported articles. Table 2 illustrates a few of the recent literatures on cholesterol sensing platforms, through both enzymatic and non-enzymatic sensing routes. The detection limit and sensitivity of the present sensing strategy is comparatively better than the reported ones.
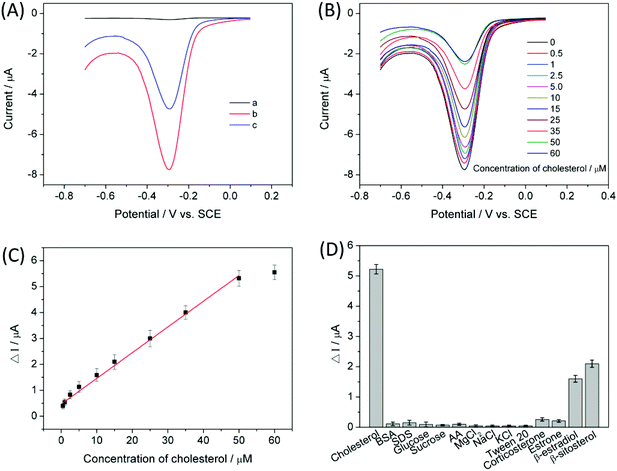 |
| Fig. 4 (A) DPV response of the CX6–Gra/GCE in 0.1 M pH 7.0 PBS (a); DPV response of the CX6–Gra/GCE incubated in 100 μM MB solution for 30 min and then tested in 0.1 M pH 7.0 PBS (b); DPV response of the CX6–Gra/GCE incubated in 100 μM MB solution for 30 min and further incubated in 25 μM cholesterol solution for 30 min and then tested in 0.1 M pH 7.0 PBS (c). (B) DPV curves of the proposed sensing platform under different concentrations of cholesterol. (C) Calibration curves for the determination of cholesterol using the proposed sensor. The error bars represent the standard deviations of three parallel tests. (D) Interference studies using different species in the developed cholesterol detection method, using DPV and keeping all the parameters constant. The cholesterol concentration was 50 μM against the concentration of all other substances, which was kept at 2.0 mM. | |
Table 2 Comparison of the present work with other recent literatures, using various electrodes or matrices for cholesterol sensing
Electrode or matrix |
Method |
Linear range (μM) |
LOD (μM) |
Ref. |
Nafion/ChOx/GNPs-MWCNTs/GCE |
DPV |
10.0–5000.0 |
4.3 |
27
|
Chit-Hb/chit-ChOx |
Amperometry |
10.0–600.0 |
9.5 |
28
|
ChEt-ChOx/ZnO-CuO/ITO/glass |
CV |
500.0–12 000.0 |
500.0 |
22
|
ChOx/PANI/PVP/graphene |
Amperometry |
50.0–10 000.0 |
1.0 |
29
|
ChOx/nano-ZnO/ITO |
CV |
130.0–10 360.0 |
13.0 |
30
|
ChOx/ZnO(T)/CT/GCE |
CV |
400.0–4000.0 |
200.0 |
31
|
Nafion/ChOx/Fe2O3 |
CV |
100.0–8000.0 |
18.0 |
32
|
AuE/dithiol/AuNPs/MUA/ChOx |
CV |
40.0–220.0 |
34.6 |
33
|
Grp/β-CD/methylene blue |
DPV |
1.0–100.0 |
1.0 |
12
|
Grp/β-CD/rhodamine 6G |
Fluorescence |
5.0–30.0 |
5.0 |
9
|
CX6–Gra/GCE |
DPV |
0.5–50.0 |
0.20 |
This work |
Selectivity
As we know, human blood serum contains many more biocomponents like salts, amino acids, carbohydrates, lipids etc., which can interfere with cholesterol detection and hamper the selectivity of the electrochemical sensor. Therefore, we have tested interference from common molecules present in human blood serum and found very negligible interference. As shown in Fig. 4D, some salts, carbohydrates, proteins, anionic surfactants, etc. including glucose, sucrose, ascorbic acid (AA), bovine serum albumin (BSA), sodium dodecyl sulphate (SDS), Tween 20, NaCl, KCl, and MgCl2 showed negligible interference even at a concentration of 2.0 mM compared to cholesterol detected at only a 50 μM concentration. In addition, several analogues of cholesterol including corticosterone, estrone, β-estradiol, and β-sitosterol were also used for the interference study. The results indicated that corticosterone and estrone exhibited weak interference while β-estradiol and β-sitosterol produced serious interference. This was ascribed to the similar structures of β-estradiol, β-sitosterol and cholesterol. The reproducibility and stability of the sensor were also studied and are supplied in the ESI.†
Real sample analysis
The proposed method was used to detect cholesterol in serum samples using standard addition methods to evaluate the feasibility of the MB-bound CX6–Gra/GCE for real sample analysis. The serum sample was diluted fifty times with 0.1 M pH 7.0 PBS. The results showed recoveries ranging from 99.0% to 104.4% and RSDs ranging from 2.45% to 4.32% (Table 3). The results demonstrated that this method can be extended for cholesterol detection in blood. Although 2.0 mM of β-estradiol and β-sitosterol seriously interfere with the detection of cholesterol (50 μM), the cholesterol detection in human serum was also very successful. This may be due to the very low concentration of β-estradiol in human plasma.34 Serum β-estradiol levels vary over a range from 41 to 272 pg mL−1 during the menstrual cycle in pre-menopausal women, and the β-estradiol levels decrease to about 4–14 pg mL−1 after the menopause.35 In the case of β-sitosterol, it mainly exists in plants. The proposed sensing platform may also be expanded to wide and potential applications in biological samples. It is worth noting that CX6 is more stable than cholesterol selective enzymes (mostly oxidase) under complex conditions. Thus, the present sensing platform seems to be more suitable for the analysis of practical cholesterol samples than traditional enzyme-based biosensors.
Table 3 Determination of cholesterol in human serum samples (n = 3)
Sample |
Added (μM) |
Found (μM) |
RSD (%) |
Recovery (%) |
1 |
5.0 |
4.95 ± 0.22 |
4.32 |
99.0 |
2 |
10.0 |
10.22 ± 0.35 |
3.51 |
102.2 |
3 |
20.0 |
20.08 ± 0.54 |
2.45 |
104.4 |
Conclusions
In summary, a sensitive and selective electrochemical approach for cholesterol sensing based on a competitive host–guest interaction between CX6 and signal probe/target molecules using a CX6–Gra-modified electrode was developed. Due to the good electron transfer property of Gra and the excellent host–guest recognition of CX6, the developed CX6–Gra/GCE displays excellent analytical performance for the electrochemical detection of cholesterol: the linear response range is 0.50–50.00 μM and the LOD is 0.20 μM (S/N = 3). In addition, the developed electrochemical sensing platform is important as it does not use any enzyme or antibody for the detection of cholesterol efficiently and selectively over common interfering species. Molecular modeling calculations revealed that the complexation of cholesterol and CX6 could reduce the energy of the system and the complex of a 1
:
1 host–guest stoichiometry had the lowest ΔG value of −8.01 kcal mol−1. The molecular docking studies suggested that hydrogen bonding, electrostatic interactions, and hydrophobic interactions should be the predominant driving forces for the formation of the inclusion complex.
Acknowledgements
This work was financially supported by the National Natural Science Foundation of China (21565029, 31160334) and the Natural Science Foundation of Yunnan Province (2014RA022, 2012FB112), People's Republic of China.
Notes and references
- F. Biedermann, V. D. Uzunova, O. A. Scherman, W. M. Nau and A. D. Simone, J. Am. Chem. Soc., 2012, 134, 15318–15323 CrossRef CAS PubMed.
- F. Biedermann, M. Vendruscolo, O. A. Scherman, A. D. Simone and W. M. Nau, J. Am. Chem. Soc., 2013, 135, 14879–14888 CrossRef CAS PubMed.
- G. Ghale and W. M. Nau, Acc. Chem. Res., 2014, 47, 2150–2159 CrossRef CAS PubMed.
- F. Biedermann and W. M. Nau, Angew. Chem., Int. Ed., 2014, 53, 5694–5699 CrossRef CAS PubMed.
- C. F. Li, J. X. Feng and H. X. Ju, Analyst, 2015, 140, 230–235 RSC.
- A. Praetorius, D. M. Bailey, T. Schwarzlose and W. M. Nau, Org. Lett., 2008, 10, 4089–4092 CrossRef CAS PubMed.
- G. Ghale, V. Ramalingam, A. R. Urbach and W. M. Nau, J. Am. Chem. Soc., 2011, 133, 7528–7535 CrossRef CAS PubMed.
- J. M. Chinai, A. B. Taylor, L. M. Ryno, N. D. Hargreaves, C. A. Morris, P. John Hart and A. R. Urbach, J. Am. Chem. Soc., 2011, 133, 8810–8813 CrossRef CAS PubMed.
- A. Mondal and N. R. Jana, Chem. Commun., 2012, 48, 7316–7318 RSC.
- X. Mao, D. Tian and H. Li, Chem. Commun., 2012, 48, 4851–4853 RSC.
- G. B. Zhu, L. Wu, X. Zhang, W. Liu, X. H. Zhang and J. H. Chen, Chem. – Eur. J., 2013, 19, 6368–6373 CrossRef CAS PubMed.
- N. Agnihotri, A. D. Chowdhury and A. De, Biosens. Bioelectron., 2015, 63, 212–217 CrossRef CAS PubMed.
- X. Zhang, L. Wu, J. W. Zhou, X. H. Zhang and J. H. Chen, J. Electroanal. Chem., 2015, 742, 97–103 CrossRef CAS.
- L. Mutihac, J. H. Lee, J. S. Kim and J. Vicens, Chem. Soc. Rev., 2011, 40, 2777–2796 RSC.
- R. N. Dsouza, U. Pischel and W. M. Nau, Chem. Rev., 2011, 111, 7941–7980 CrossRef CAS PubMed.
- J. Zhou, M. Chen and G. W. Diao, ACS Appl. Mater. Interfaces, 2013, 5, 828–836 CAS.
- D. Chen, L. H. Tang and J. H. Li, Chem. Soc. Rev., 2010, 39, 3157–3180 RSC.
- D. R. Dreyer, S. Park, W. C. Bielawski and R. S. Ruoff, Chem. Soc. Rev., 2010, 39, 228–240 RSC.
- X. J. Chen, R. A. Boulos, P. K. Eggers and C. L. Raston, Chem. Commun., 2012, 48, 11407–11409 RSC.
- E. Eroglu, W. Z. Zang, P. K. Eggers, X. J. Chen, R. A. Boulos, M. Haniff Wahid, S. M. Smith and C. L. Raston, Chem. Commun., 2013, 49, 8172–8174 RSC.
- X. J. Chen, C. T. Gibson, J. Britton, P. K. Eggers, M. Haniff Wahid and C. L. Raston, Chem. Commun., 2015, 51, 2399–2402 RSC.
- N. Batra, M. Tomar and V. Gupta, Biosens. Bioelectron., 2015, 67, 263–271 CrossRef CAS PubMed.
- J. Motonaka and L. R. Faulkner, Anal. Chem., 1993, 65, 3258–3261 CrossRef CAS PubMed.
- Y. Guo, S. Guo, J. Ren, Y. Zhai, S. Dong and E. Wang, ACS Nano, 2010, 4, 4001–4010 CrossRef CAS PubMed.
- C. Z. Zhu, S. J. Guo, Y. X. Fang and S. J. Dong, ACS Nano, 2010, 4, 2429–2437 CrossRef CAS PubMed.
-
A. J. Bard and L. R. Faulkner, Electrochemical Methods: Fundamentals and Applications, Wiley, New York, 2nd edn, 2001 Search PubMed.
- L. Zhu, L. Xu, L. Tan, H. Tan, S. Yang and S. Yao, Talanta, 2013, 106, 192–199 CrossRef CAS PubMed.
- C. Z. Zhao, L. Wan, L. Jiang, Q. Wang and K. Jiao, Anal. Biochem., 2008, 383, 25–30 CrossRef CAS PubMed.
- N. Ruecha, R. Rangkupan, N. Rodthongkum and O. Chailapakul, Biosens. Bioelectron., 2014, 52, 13–19 CrossRef CAS PubMed.
- A. Ahmadalinezhad and A. C. Chen, Biosens. Bioelectron., 2011, 26, 4508–4513 CrossRef CAS PubMed.
- A. K. Giri, A. Sinhamahapatra, S. Prakash, J. Chaudhari, V. K. Shahi and A. B. Panda, J. Mater. Chem. A, 2013, 1, 814–822 CAS.
- A. Umar, R. Ahmad, S. W. Hwang, S. H. Kim, A. Al-Hajry and Y. B. Hahn, Electrochim. Acta, 2014, 135, 396–403 CrossRef CAS.
- U. Saxena, M. Chakraborty and P. Goswami, Biosens. Bioelectron., 2011, 26, 3037–3043 CrossRef CAS PubMed.
- M. J. Monerris, F. J. Arévalo, H. Fernández, M. A. Zon and P. G. Molina, Sens. Actuators, B, 2015, 208, 525–531 CrossRef CAS.
- T.-B. Xin, H. Chen, Z. Lin, S.-X. Liang and J.-M. Lin, Talanta, 2010, 82, 1472–1477 CrossRef CAS PubMed.
Footnotes |
† Electronic supplementary information (ESI) available. See DOI: 10.1039/c5an01843a |
‡ These authors contributed equally to this work. |
|
This journal is © The Royal Society of Chemistry 2016 |
Click here to see how this site uses Cookies. View our privacy policy here.