DOI:
10.1039/C5AY02230D
(Paper)
Anal. Methods, 2016,
8, 214-221
Near infrared fluorescence-magnetic resonance dual-modal imaging with Cy5-labeled, Gd–Al co-doped mesoporous silica nanoparticles†
Received
24th August 2015
, Accepted 7th November 2015
First published on 17th November 2015
Abstract
To obtain comprehensive information and to compensate the limits of individual modalities in molecular imaging, the research on dual-modal imaging probes (DMIPs) attracts much interest. Here, we report a reliable and facile procedure to prepare near infrared fluorescence (NIRF)-magnetic resonance (MR) DMIPs using Cy5-labeled, Gd–Al co-doped mesoporous silica nanoparticles (MSNs). Amine-modified, Gd–Al co-doped MSNs were first synthesized through one-step co-condensation. A Cy5-NHS ester was then coupled to the amino groups to produce the DMIPs, which were denoted as Gd–Al@MSNs-Cy5. High intensity NIRF and a relaxation rate (17.7 mM−1 s−1) were observed. Featuring the lower background and the deeper penetration depth, the cutting-edge NIRF imaging was achieved. Moreover, the larger surface area of MSNs could be modified with more NIRF probes, Cy5, for improved NIRF. Following the Solomon–Bloembergen–Morgan theory, enhanced MR performance was illustrated by the relaxation rate because MSNs have a porous structure that enables access of water into the interior, and co-doping with Al in the MSNs promotes the doped amount of Gd. The exchange rate of water is much more accelerated through doping Gd and Al since a disordered pore arrangement is found in Gd–Al co-doped MSNs. The DMIPs in saline were injected into mice through the tail vein for imaging and basic pharmacokinetics studies. They were excreted by the stomach and intestine in 3 h, a circulation time long enough for imaging. Thus, Gd–Al@MSNs-Cy5 are attractive for clinical NIRF-MR dual-modal imaging by integrating the biocompatibility of MSNs, the NIRF of Cy5, and the high MR response of Gd.
Introduction
Molecular imaging reveals the generation, development, and metastasis of tumor, allowing precise delivery of medicines and dynamic monitoring of personalized treatment.1,2 To obtain comprehensive information and to compensate the limits of individual modalities in clinical diagnostics, dual-modal imaging probes (DMIPs) have been rapidly developed.3 Fluorescence imaging and magnetic resonance (MR) imaging are considered to be a complementary pair. Fluorescence imaging shows high sensitivity but poor spatial resolution with a relatively moderate tissue penetration depth, while MR imaging is unlimited in the penetration depth and prominent in spatial resolution yet lacks sensitivity.4 The first fluorescence-MR DMIPs were introduced by Huber et al. in 1998, where rhodamine derivatives and Gd lay the foundations for fluorescence and MR imaging, respectively.5 However, several issues impede the real bio-medical applications of the fluorescence-MR DMIPs, including but not restricted to (1) a high background and rather limited penetration depth of fluorescence, (2) an insufficient MR imaging contrast agent payload, (3) inadequate surface areas to be modified or to contact with water in nanoparticle-based DMIPs, and (4) complex design and laborious preparation of DMIPs.
Traditional fluorescence imaging is subjected to auto-fluorescence and the low penetration depth of the tissue, leading to a high level of background and inaccuracy, whereas longer wavelength light, such as near infrared fluorescence (NIRF) that ranges from 650 to 1350 nm in wavelength, is less absorbed or scattered by tissues.6 Featuring the lower background, deeper penetration depth, and higher spatial resolution,7,8 the cutting-edge NIRF imaging overmatches the other optical techniques. Currently developed NIRF imaging probes can be classified into two categories: Stokes type and anti-Stokes type (up-conversion). The latter refers to precious rare earth element doped nanoparticles,9 while the former is more common and mainly includes fluorescent proteins,10 organic dyes,11 metallorganics,12 and nanomaterials, e.g., gold clusters,13 silver clusters,14 quantum dots, etc.15 For biodegradability, non-toxicity, and convenient labeling concerns, organic NIRF dyes have been widely used, like Cy5,16 Cy5.5,17 and indocyanine green.18
Unlike many other modalities, 3D magnetic resonance (MR) imaging is both non-radiative and non-invasive. It is especially valuable for visualizing soft tissues. MR images are created by recording the demagnetization (relaxation) rates of magnetized protons of water in different chemical environments, and then converting them into grayscale images.19 Since the chemical environments in different tissues are often dissimilar, the contrast is formed between adjacent tissues. The contrast agent will further enhance the inherent contrast by shortening the relaxation course of nearby protons. Examples are paramagnetic metal complexes or species containing Gd20 or Mn21 and superparamagnetic iron oxide.22 In particular, Gd-containing species are intensely studied because Gd3+ has up to seven unpaired electrons; a large magnetic moment is therefore expected. According to Solomon–Bloembergen–Morgan theory, the relaxation time decreases with increasing hydration number of Gd, accelerating the exchange rate of the inner shell and outer shell water, and increasing the molecular weight of Gd compounds.23 Besides, multiple Gd centers in one molecular unit help quick relaxation.24 Only one water binding site is left in two approved contrast agents in clinical MR imaging, Gd-DOTA and Gd-DTPA. New ligands with more than one site need careful design and synthesis.25 An alternative strategy to design improved Gd-containing contrast agents is loading them on nanoparticle carriers, increasing the molecular weight and the payload of Gd complexes simultaneously.26,27
New methodologies enable the synthesis of functional nanoparticles with a controllable morphology and structure.28 Modifying the surface alters their physical properties and expands their functions, which is the basis of theranostics.29 DMIPs are preferentially built on nanoparticles with respect to the enhanced permeability and retention effect that makes the tumor passive-targetable, thus precise imaging and drug-delivery become practical.30 Nevertheless, post-linking of Gd complexes on solid nanoparticles results in limited outer surface labelling, while doping Gd complexes in the inner affects the exchange rate of water. We therefore explore nanoparticles with substantial Gd-doping, without perturbing the exchange rate of water simultaneously.31 The biocompatible mesoporous silica nanoparticles (MSNs) are the best, as their porous structure enables access of water into the interior, along with a larger surface area that can be modified. Previous literature studies proved that co-doping with Al in the MSNs promotes the doped amount of Gd.32,33 Because Al3+ is similar to Si4+ in size, the negative charge is produced when Al3+ is incorporated into the MSNs during their formation. Gd3+ as a counter-ion is loaded by ion-exchange to balance the charge. Importantly, the exchange rate of water is much more accelerated through doping Gd and Al, since a disordered pore arrangement is found in Gd–Al doped MSNs, where the interconnection of pores facilitates the free diffusion of water.33,34 In our previous work, Ru(bpy)32+ was integrated into Gd–Al doped MSNs via an ion-exchange procedure as fluorescence-MR DMIPs.33 However, Ru(bpy)32+ suffers from low fluorescence efficiency and the ion-exchanged Ru(bpy)32+ may be leaked.
Here, we report a reliable and facile procedure to prepare NIRF-MR DMIPs using Cy5-labeled, Gd–Al co-doped MSNs (Gd–Al@MSNs-Cy5). Different to the simple MSNs reported in our previous work,33 amine-modified, Gd–Al co-doped MSNs (Gd–Al@MSNs-NH2) were first synthesized through one-step co-condensation. Cy5-NHS ester was then coupled to the amino groups to produce the DMIPs. Different to the previous DMIPs,33,35,36 Gd–Al@MSNs-Cy5 address the aforementioned four issues well. Highly intensive NIRF from Cy5 enjoys the low background and high penetration depth. While co-doped Al increases the loading of Gd ions, the mesoporous structure enhances the exchange of water molecules, so a high relaxation rate and contrast efficiency were observed. Importantly, mesoporous silica provides a biocompatible platform to integrate the signal sources, Gd ions and Cy5, by a simple preparation procedure with high yield. All of the properties were validated by the imaging and basic pharmacokinetics study with mice as the model through tail vein injection.
Experimental
Chemicals and apparatus
Cetyltrimethyl ammonium bromide (CTAB), diethanolamine (DEA), and (3-aminopropyl)triethoxysilane (APTES) were purchased from J&K Scientific. Tetraethoxysilane (TEOS) was from Concord, Tianjin, China. Aluminum chloride (AlCl3) and gadolinium oxide (Gd2O3) were from Aladdin Industrial. Absolute anhydrous N,N-dimethylformamide (DMF) was from Heowns, Tianjin, China. Cyanine5 NHS ester (Cy5-NHS) was obtained from Fanbo, Beijing, China. GdCl3 was produced by the reaction of Gd2O3 with hydrochloric acid. All other reagents were of analytical grade. Ultrapure water was used throughout the experiment.
Fourier transform infrared (FTIR) spectra were recorded by using a Nicolet Magna 560 IR spectrometer. Transmission electron microscopy (TEM) images were captured by using an FEI Tecnai G2 transmission electron microscope. N2 adsorption–desorption outcomes were analyzed with a Micromeritics TriStar 3000 surface area and pore size analyzer. Elemental contents were determined by Thermo IRIS Intrepid II XSP inductively coupled plasma atomic emission spectroscopy (ICP-AES). Fluorescence was recorded using a Hitachi FL-4500 fluorescence spectrophotometer, and NIRF imaging was performed by using a Kodak in vivo imaging system FX. The relaxation time measurement and MR imaging were executed with a Huantong (Shanghai, China) HT-MRSI60-60 KY MR imaging scanner.
Synthesis of Gd–Al@MSNs-NH2
The synthesis of Gd–Al@MSNs-NH2 resembles the previous studies.37–40 After dissolving 0.29 g CTAB (0.79 mmol) in a mixture of 6.4 mL water (0.36 mol) and 0.92 mL ethanol (0.02 mol) by sonication for 10 min, 0.05 g DEA (0.48 mol) was added and heated to 60 °C. TEOS was added drop-wise under vigorous stirring for 10 min (ESI, Table S1†). Then, 0.20 mL of a GdCl3 (0.13 mmol) and AlCl3 (0.06 mmol) solution was added, and the reaction proceeded for 1 h. An equal volume of TEOS and APTES was added together and allowed to react for another 2 h period to integrate amine groups into the exterior and interior surface. Subsequently, the reaction system was allowed to cool, and the nanoparticles, Gd–Al@MSNs-NH2, were washed with ethanol three times. The collected nanoparticles were refluxed in acidified ethanol for 6 h to remove CTAB, and finally dispersed in 10 mL ethanol after washing.
Labelling Cy5 on Gd–Al@MSNs-NH2
25 mg of dry Gd–Al@MSNs-NH2 was dispersed in 8 mL anhydrous DMF containing 1 mg Cy5-NHS and 80 μL tripropylamine. The mixture was stirred in the dark at room temperature for 24 h. Then the nanoparticles, Gd–Al@MSNs-Cy5, were washed with ethanol five times and dispersed in 5 mL water.
Toxicity of Gd–Al@MSNs-Cy5
The cell viability was recorded after HepG2 cells were incubated with Gd–Al@MSNs-Cy5 at different concentrations using a standard MTT [3-(4,5-dimethylthiazol-2-yl)-2,5-diphenyltetrazolium bromide] assay to test the toxicity of Gd–Al@MSNs-Cy5. The cells were incubated in 96-well culture plates at a density of 5 × 103 cells per well in culture medium. Gd–Al@MSNs-Cy5 and Gd3+ ions at concentrations from 0 to 20 mg L−1 (based on Gd3+) were introduced into the medium and incubated HepG2 cells for 24 h. MTT solution (20 mL, 0.1 mg) was added to each well and the cells were incubated for another 4 h. N,N′-Dimethyl sulfoxide (150 μL) was used to completely liberate the formazan crystals. The absorbance at 490 nm was measured for the calculation of the cell viability.
Fluorescence measurements and NIRF imaging
Steady-state fluorescence was excited with 630 nm wavelength at room temperature using a Hitachi FL-4500 fluorescence spectrophotometer. The excitation source is a 450 W xenon lamp coupled to a monochromator with a 1200 groves per mm grating. During in vivo and ex vivo imaging, female nude mice (6 weeks old, BALB/c-nu) were initially anesthetized with 4% chloral hydrate in a dosage of 8.25 μL g−1, and further anesthetized with 2% chloral hydrate. Then, 200 μL of 10 mg mL−1 Gd–Al@MSNs-Cy5 saline was injected through the tail vein of the mice. The fluorescence was excited at 625 nm wavelength and collected at 690 nm. The black and white fluorescence images were superimposed for better visualization. Finally, the mice were sacrificed and dissected to image the individual organs. The mice were obtained from the Institute of Hematology & Hospital of Blood Diseases, Chinese Academy of Medical Sciences & Peking Union Medical College with the license no. SCXK-2004-001, Tianjin, China. The mice had free access to solid rodent chow and water. All experimental protocols using animals were also approved by the Institutional Animal Care Committee of Nankai University.
Relaxation time measurements and MR imaging
Relaxation time was measured on an HT-MRSI60-60KY MR imaging scanner (1.2 T, 30 °C) using the inversion-recovery method. In vitro T1-weighted phantom imaging and in vivo MR imaging (using female Kunming mice, 8 weeks old, outbred stock) were based on a spin-echo method. 300 μL of 10 ng mL−1 saline solution of Gd–Al@MSNs-Cy5 was injected into the mice through the tail vein. MR imaging was conducted using a T1-weighted sequence with TR/TE = 200/14 ms, FOV = 75 mm × 75 mm, flip angle = 60°, and number of excitation = 3.
Results and discussion
Synthesis of the DMIPs
MSNs were prepared to load with Cy5 and Gd3+ for the synthesis of the NIRF-MR DMIPs. Hydrolyzation and co-condensation of TEOS and APTES are extensively reported to directly produce NH2-functional MSNs by a simple procedure with high yield.35,36 Precipitation is avoided and amine groups are homogeneously distributed, forming Gd–Al@MSNs-NH2 in one step.40 The impact of the amount of both TEOS and APTES on the surface charge of the Gd–Al@MSNs-NH2 is summarized in Table S1.† More APTES, more positive charges, which means more amino groups are formed on the Gd–Al@MSNs-NH2. The increase of the absolute value of zeta-potential favors the dispersion and stability of the Gd–Al@MSNs-NH2. The composition and ratio of Sample 4 in Table 1 are optimal, considering the stability, the Gd-loading amount, the relaxation rate, and the number of amine groups that coupled with Cy5. The zeta potential of the Gd–Al@MSNs-Cy5 was tested and −7.8 mV, which is lower than that of mesoporous silica nanoparticles because of the existence of amino groups. The amine group-modified mesoporous structure provides a platform to integrate Gd ions and Cy5 for MR and NIRF dual-response.
Table 1 The amount of Gd and Al in various Gd–Al@MSNs-NH2 samples and their respective relaxation data
Sample # |
Gd (wt%) |
Al (wt%) |
Gd (mmol g−1) |
Al (mmol g−1) |
Al/Gd (m m−1) |
r
1 (mM−1 s−1) |
r
2/r1 |
The optimal Gd–Al@MSNs-NH2 and their respective relaxation data.
|
1 |
4.52 |
0.943 |
0.287 |
0.350 |
1.22 |
14.2 |
1.3 |
2 |
4.46 |
0.869 |
0.284 |
0.330 |
1.17 |
12.6 |
2.0 |
3 |
4.03 |
0.801 |
0.256 |
0.297 |
1.16 |
13.9 |
1.7 |
4
|
4.27
|
0.806
|
0.272
|
0.299
|
1.10
|
17.3
|
1.1
|
5 |
3.78 |
0.664 |
0.240 |
0.246 |
1.02 |
16.5 |
1.2 |
Morphology and structure of the DMIPs
The morphology and microstructure of the DMIPs were characterized. All nanoparticles appeared to be uniform spheres as shown in the TEM images in Fig. 1. The average diameters of Gd–Al@MSNs-NH2 and Gd–Al@MSNs-Cy5 were ca. 57 nm. In addition, the mesoporous structure of the intragranular network of the nanoparticles was observed clearly (Fig. 1B). Since post-grafting of Cy5 to the surfaces and pores would not influence the dispersion and the structure of the nanoparticles, the exchange rate of water between the surroundings and the internals of nanoparticles was not interfered, thus maintaining high relaxivity.
 |
| Fig. 1 TEM images of (A) Gd–Al@MSNs-NH2 and (B) Gd–Al@MSNs-Cy5. The inset shows the same sample at high resolution with a 20 nm scale. (C) Illustration of NIRF-MR DMIPs of the mesoporous structure with the co-doped Gd and Al as well as the labeled Cy5. | |
The mesoporous structure of Gd–Al@MSNs-NH2 was further investigated by XRD and N2 adsorption–desorption tests. Doping with Al3+ decreases the size of the MSNs as a result of the pH decrease of the reaction system after addition of Al3+.33,38 Moreover, the Al3+ co-dopant could greatly reduce the structural stress within the silicate matrix, induced by the charge and size mismatch of the trivalent Gd3+, which therefore disrupted the order of the MSN structure.41 Consequently, the XRD peaks of Gd–Al@MSNs-NH2 became broader and some of them disappeared. Long-range order MSNs like hexagonal MCM-41 are typically characterized to exhibit three sharp Bragg peaks (100), (110), and (200) at two-theta angles less than 5°, while only one broad, weak peak at low angles indexed to (100) diffractions of Gd–Al@MSNs-NH2 was found, as shown in Fig. S1A (ESI†). N2 adsorption–desorption of Gd–Al@MSNs-NH2 reflects their textural properties (Fig. S1B†). A type IV isotherm with an evident hysteresis loop at high pressures accounts for the mesoporous nature and the small size of Gd–Al@MSNs-NH2. Using the Brunauer–Emmett–Teller test, the surface area is 882.7 m2 g−1, and total pore volume is 1.2 cm3 g−1. The maximum pore size is 2.5 nm (inset) based on the Barrett–Joyner–Halenda (BJH) test. The BJH model is based on the Kelvin equation: ln
p/p0 = −2σVL
cos
θ/RTrk. The rk obtained by using the Kelvin equation plus the thickness of the liquid film is the pore diameter. The small size and large pores facilitate the loading of a substantial amount of Cy5 and the exchange of water to maintain the high relaxivity. Based on the results above, Fig. 1C illustrates the mesoporous structure with the co-doped Gd and Al as well as the labeled Cy5.
The conjugation of Cy5 with Gd–Al@MSNs-NH2 was confirmed by using the FTIR spectra. As shown in Fig. S2a (ESI†), the band between 3500 and 3200 cm−1 is assigned to the N–H stretching vibrations of primary amine; the peaks near 1640 and 1560 cm−1 are the bending vibrations of primary amine, which prove the existence of the amine group on Gd–Al@MSNs-NH2. Amide characteristic stretching vibrations appear at 1450 cm−1 (Fig. S2b†), and there are two sharp peaks at 1180 and 1130 cm−1 related to stretching vibration of aromatic sulfonic acid groups on Cy5, so Cy5 is successfully linked to Gd–Al@MSNs-NH2. The loading content of Cy 5 was 4.17 × 10−5 mol g−1, which was calculated based on the fluorescence intensity from the probe with the emission of Cy5 solution as the standard.
Fluorescence properties of the DMIPs
The optical properties of the Gd–Al@MSNs-Cy5 were investigated. While Gd–Al@MSNs-Cy5 solution was cyan (Fig. 2A), NIRF emission was observed when illuminated by using 365 nm UV light (Fig. 2B). As shown in Fig. 2C, the maximum emission wavelengths are 682 and 684 nm for Cy5 and Gd–Al@MSNs-Cy5, respectively, under 630 nm excitation. The nearly identical wavelengths indicate that the luminescence properties of Cy5 remained even when Cy5 was linked to the MSNs. Strong NIRF from Cy5 can increase the signal-to-noise ratio during in vivo fluorescence imaging of the probe.
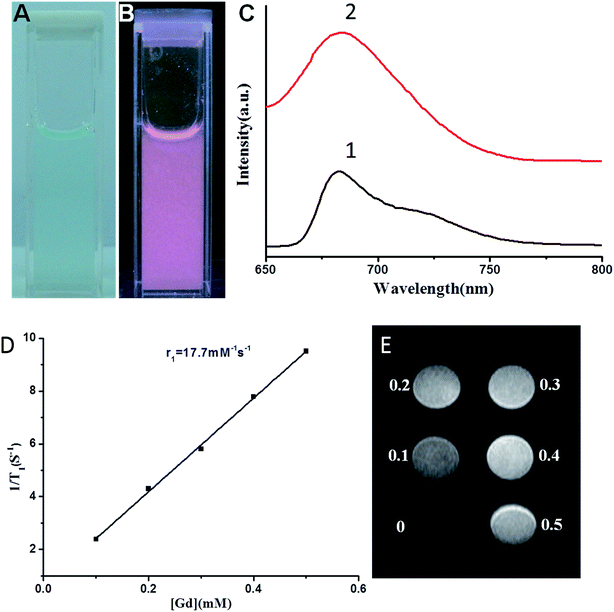 |
| Fig. 2 Gd–Al@MSNs-Cy5 at a concentration of 1 mg mL−1 under (A) natural and (B) UV light. (C) The fluorescence spectrum of (1) Cy5 and (2) Gd–Al@MSNs-Cy5 at an excitation of 630 nm wavelength. (D) The r1 relaxation curve of Gd–Al@MSNs-Cy5. (E) T1-weighted phantom images of different concentrations of Gd–Al@MSNs-Cy5. | |
Relaxation properties of the DMIPs
The content of Gd is a critical parameter of Gd-related contrast agents. When we calculated the longitudinal and transverse relaxation rate, r1 and r2, the precise concentration of Gd is necessarily acquired. After Gd–Al@MSNs-Cy5 were dissolved in a nitric acid/hydrofluoric acid mixture, they were subsequently analyzed by ICP-AES to quantify the amount of Gd and Al. Table 1 summarizes the amount of Gd and Al, and the relaxation rate of different Gd–Al@MSNs-NH2. It can be seen from Table S1† that the amount of Gd and Al decreases with increasing APTES. The negative charge is produced when Al is incorporated into the MSNs, and Gd, as a counter-ion, is loaded into the MSNs by ion-exchange to balance the charge.42 Simultaneously, the positive charge formed during APTES hydrolysis, which balances part of the negative charge generated by TEOS hydrolysis. Because APTES could lower the negative charge in the reaction, the amount of Gd and Al in the MSNs is decreased. Sample 4 in Table 1 shows the highest relaxation properties, with 17.3 mM−1 s−1 of longitudinal relaxation rate, 4.1-fold higher than that of Gd-DTPA.33 The probe's r2/r1 is ca 1.1, which is suitable as a positive contrast agent. In general, r2/r1 less than 2 is common for T1 contrast agents.25 Therefore, DMIPs can be established using near-infrared dye linked Sample 4 in Table S1.†
The content of Gd and Al in Gd–Al@MSNs-Cy5 is 259.0 μmol g−1 and 263.2 μmol g−1, respectively, while the molar ratio of Al and Gd is 1.02, with r1 of 17.7 mM−1 s−1 and 1.1 of r2/r1 ratio. Compared with Sample 4, little influence was observed when coupling Cy5 on the surface of the pores and nanoparticles, indicating that Cy5 cannot hinder the diffusion and exchange rate of water. The results in Fig. 2D and E illustrated that the as-prepared DMIPs are applicable for in vivo MR applications.
Stability of the DMIPs
The leakage of metal ions from 0.5 mg mL−1 Gd–Al@MSNs-Cy5 in phosphate buffer saline (PBS, pH 7.4) is measured by ICP-AES to illustrate the stability of DMIPs. The DMIPs contained 2036 ppm Gd and 355 ppm Al (the limit of detection for Gd and Al is 0.0154 ppm and 0.0174 ppm, respectively). No Gd or Al in the supernatant after centrifugation was detected after one day, one week, and even one month. Any leakage of Gd and Al from the DMIPs is avoided.
Gd3+ ions are toxic and often used in the form of complexes to reduce their toxicity. We tested the cell viability after being incubated with the Gd–Al@MSNs-Cy5 probe and Gd ions with HepG2 cells as the model. As shown in Fig. S3,† while the cell viability was almost the same around 100% after incubation with the Gd–Al@MSNs-Cy5 probe at different concentrations, Gd3+ ions led to the gradually decreased cell viability with the increased concentration. Only 30% cell viability was observed at 20 mg L−1 of Gd3+ ions. The above results confirmed the high biocompatibility of MSNs and less leakage of Gd3+ ions from the probe.
The fluorescence of pure PBS and the supernatant after centrifugation of Gd–Al@MSNs-Cy5 in PBS is measured, after one day, one week, and one month. The ratio of the intensity is 1
:
1.03
:
1.03
:
1.02, so little Cy5 is leaked, which contributes to the covalent coupling on the nanoparticles. The stability of fluorescence intensity and relaxation rate was also examined. The Gd–Al@MSNs-Cy5 were kept in the suspension and stored away from light at 4 °C during the test. As illustrated in Fig. S4 (ESI†), the fluorescence intensity and relaxation rate remained the same after 10 days.
NIRF imaging using the DMIPs
In vivo NIRF and MR imaging of mice using Gd–Al@MSNs-Cy5 was performed to show their bio-effects and imaging application. The BALB/c mice were injected with 200 μL colloidal saline of DMIPs at a concentration of 10 mg mL−1 through the tail vein. Cy5-labeling is better than fluorescein or rhodamine-labeling, because its emission could only be weakly absorbed by the tissue.43 As shown in Fig. 3, the NIRF image of the mice verifies that the bright fluorescence could penetrate the body. We monitored the mice after injection in the period between 0.5 and 3 h (Fig. 3A). Strong emission was observed throughout the body after injection, which is different to the low fluorescence efficiency of Ru(bpy)32+ used in our previous work.33 By systematic circulation, the DMIPs gradually accumulated in the mononuclear phagocyte system like liver and spleen. Finally, most of the DMIPs were excreted by the stomach and intestines. 3 h later, mice were sacrificed and dissected to confirm the bio-distribution of the DMIPs. As shown in Fig. 3B, a high intensity of fluorescence was observed in the stomach and the intestines, while no fluorescence was detected in the heart and the lung. The DMIPs could be cleared from the mice, since little were found in liver, spleen, and kidneys. The above results reveal that Gd–Al@MSNs-Cy5 can be used in in vivo NIRF imaging. The large surface area of MSNs can load more Cy5 that emits strong fluorescence, and the NIRF from Cy5 shows deep tissue penetration capability.
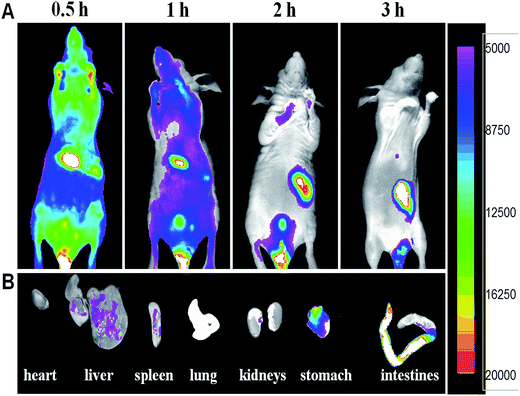 |
| Fig. 3 (A) In vivo fluorescent images of mice with intravenous injection of Gd–Al@MSNs-Cy5. (B) Ex vivo images of resected organs at 3 h after injection. | |
MR imaging using the DMIPs
The results of MR imaging were in accordance with those of the NIRF imaging (Fig. 4). The 30 g female Kunming mice were injected with 300 μL colloidal saline of Gd–Al@MSNs-Cy5 (25.6 μmol kg−1 of Gd), and the T1-weighted MR images at pre-injection through the tail-vein (blank), post-injection at 0.5, 1, 2, and 3 h, were recorded. The MR signal was first enhanced at the liver as it was the main uptake organ of the DMIPs. During the next 3 h, an evident contrast appeared at the intestines. Hence, it is speculated that after blood circulation, the DMIPs will accumulate in the liver, which are then excreted by the kidneys or enter the stomach and then reach the intestines. Similarly, no signal enhancement was spotted in heart and lung. The above results reveal that Gd–Al@MSNs-Cy5 can be used in in vivo MR imaging. Our method for synthesizing the DMIPs allows a sufficient Gd payload, and the inherent porous structure of Gd and Al co-doped MSNs further facilitates water diffusion into the inner of the DMIPs, both of which are beneficial for Gd–Al@MSNs-Cy5 in in vivo MR imaging.
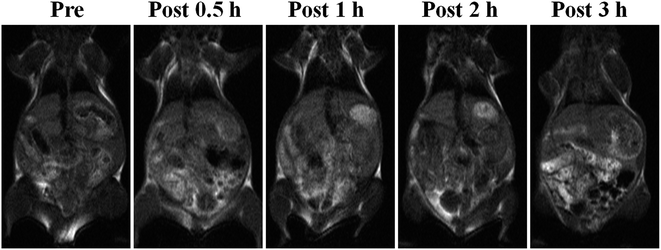 |
| Fig. 4
In vivo MR images of mice with intravenous injection of Gd–Al@MSNs-Cy5. | |
Expansibility of the DMIPs
The DMIPs presented herein overcome several limitations in nanoparticle-based dual-modal imaging. They are simple and reliable in design and preparation. In addition, their functions can be further expanded. Antibodies44 and nucleic acid aptamers 45 that recognize the cancer-related biomarkers have been identified. On another aspect, diverse therapeutic methods, including photodynamic therapy, photothermal therapy, chemotherapy, radiotherapy, etc., originate with corresponding molecules or nanoparticles.46 Smart theranostic agents can be synthesized based on our work herein, integrating the abilities of (1) active and selective multi-valency targeting of tumor by coupling various antibodies and/or aptamers on the large surface of Gd–Al@MSNs-Cy5 and (2) cooperative treatment of cancer by linking and/or entrapping therapeutic-effective molecules or nanoparticles to Gd–Al@MSNs-Cy5.
Conclusions
In this paper, NIRF-MR DMIPs using Cy5-labeled, Gd–Al co-doped MSNs were constructed reliably and facilely. Detailed design and characterization of the excellent properties of NIRF and MR were demonstrated both in vitro and in vivo, suggesting that Gd–Al@MSNs-Cy5 were an attractive candidate for clinical NIRF-MR imaging. Furthermore, it is possible to expand the functions of Gd–Al@MSNs-Cy5. Overcoming several issues common in dual-modal imaging based on functional nanoparticles, our work herein gives solutions to effectively enhance the fluorescence and MR signal intensities, and simplifies the design and preparation procedure simultaneously.
Acknowledgements
This work was supported by the National Natural Science Foundation of China (No. 21375064 and 21435001), 973 projects (No. 2015CB932001), Tianjin Natural Science Foundation (No. 15ZCZDSF00060), and Research Fund for the Doctoral Program of Higher Education (No. 20130031110016).
References
- T. Florio and F. Barbieri, Drug Discovery Today, 2012, 17, 1103–1110 CrossRef CAS PubMed.
- Z. Jia, D. Geng, T. Xie, J. Zhang and Y. Liu, J. Clin. Neurosci., 2012, 19, 820–823 CrossRef PubMed.
- S. S. Kelkar and T. M. Reineke, Bioconjugate Chem., 2011, 22, 1879–1903 CrossRef CAS PubMed.
- B. A. Smith and B. D. Smith, Bioconjugate Chem., 2012, 23, 1989–2006 CrossRef CAS PubMed.
- M. M. Huber, A. B. Staubli, K. Kustedjo, M. H. B. Gray, J. Shih, S. E. Fraser, R. E. Jacobs and T. J. Meade, Bioconjugate Chem., 1998, 9, 242–249 CrossRef CAS PubMed.
- H. Kobayashi, M. Ogawa, R. Alford, P. L. Choyke and Y. Urano, Chem. Rev., 2010, 110, 2620–2640 CrossRef CAS PubMed.
- T. Furuta, M. Nakada, K. Misaki, Y. Sato, Y. Hayashi, Y. Nakanuma and J.-I. Hamada, Brain Tumor Pathol., 2014, 31, 32–39 CrossRef CAS PubMed.
- A. D. Norden, G. S. Young, K. Setayesh, A. Muzikansky, R. Klufas, G. L. Ross, A. S. Ciampa, L. G. Ebbeling, B. Levy, J. Drappatz, S. Kesari and P. Y. Wen, Neurology, 2008, 70, 779–787 CrossRef CAS PubMed.
- J. Zhou, N. Shirahata, H.-T. Sun, B. Ghosh, M. Ogawara, Y. Teng, S. Zhou, R. G. S. Chu, M. Fujii and J. Qiu, J. Phys. Chem. Lett., 2013, 4, 402–408 CrossRef CAS PubMed.
- D. Yu, W. C. Gustafson, C. Han, C. Lafaye, M. Noirclerc-Savoye, W.-P. Ge, D. A. Thayer, H. Huang, T. B. Kornberg, A. Royant, L. Y. Jan, Y. N. Jan, W. A. Weiss and X. Shu, Nat. Commun., 2014, 5, 3626 CAS.
- C.-S. Yeh, C.-H. Su, W.-Y. Ho, C.-C. Huang, J.-C. Chang, Y.-H. Chien, S.-T. Hung, M.-C. Liau and H.-Y. Ho, Biomaterials, 2013, 34, 5677–5688 CrossRef CAS PubMed.
- H. Ke, H. Wang, W.-K. Wong, N.-K. Mak, D. W. J. Kwong, K.-L. Wong and H.-L. Tam, Chem. Commun., 2010, 46, 6678–6680 RSC.
- H. Hu, P. Huang, O. J. Weiss, X. Yan, X. Yue, M. G. Zhang, Y. Tang, L. Nie, Y. Ma, G. Niu, K. Wu and X. Chen, Biomaterials, 2014, 35, 9868–9876 CrossRef CAS PubMed.
- C. Wang, L. Xu, X. Xu, H. Cheng, H. Sun, Q. Lin and C. Zhang, J. Colloid Interface Sci., 2014, 416, 274–279 CrossRef CAS PubMed.
- I. Hocaoglu, F. Demir, O. Birer, A. Kiraz, C. Sevrin, C. Grandfils and H. Y. Acar, Nanoscale, 2014, 6, 11921–11931 RSC.
- X. He, J. Chen, K. Wang, D. Qin and W. Tan, Talanta, 2007, 72, 1519–1526 CrossRef CAS PubMed.
- L. Jiang, Q. Zhou, K. Mu, H. Xie, Y. Zhu, W. Zhu, Y. Zhao, H. Xu and X. Yang, Biomaterials, 2013, 34, 7418–7428 CrossRef CAS PubMed.
- M. A. Jarzabek, P. C. Huszthy, K. O. Skaftnesmo, E. McCormack, P. Dicker, J. H. M. Prehn, R. Bjerkvig and A. T. Byrne, Mol. Imaging, 2013, 12, 161–172 CAS.
-
P. Reimer, P. M. Parizel, J. F. M. Meaney and F. A. Stichnoth, Clinical MR Imaging: a Practical Approach, Springer, 3rd edn, 2010 Search PubMed.
- J. Tang, Y. Sheng, H. Hu and Y. Shen, Prog. Polym. Sci., 2013, 38, 462–502 CrossRef CAS.
- M. Kueny-Stotz, A. Garofalo and D. Felder-Flesch, Eur. J. Inorg. Chem., 2012, 1987–2005 CrossRef CAS.
- N. Lee and T. Hyeon, Chem. Soc. Rev., 2012, 41, 2575–2589 RSC.
- A. J. L. Villaraza, A. Bumb and M. W. Brechbiel, Chem. Rev., 2010, 110, 2921–2959 CrossRef CAS PubMed.
- C. Shen and E. J. New, Curr. Opin. Chem. Biol., 2013, 17, 158–166 CrossRef CAS PubMed.
- P. Caravan, J. J. Ellison, T. J. McMurry and R. B. Lauffer, Chem. Rev., 1999, 99, 2293–2352 CrossRef CAS PubMed.
- F. Chen, W. Bu, S. Zhang, J. Liu, W. Fan, L. Zhou, W. Peng and J. Shi, Adv. Funct. Mater., 2013, 23, 298–307 CrossRef CAS.
- Y. Liu and N. Zhang, Biomaterials, 2012, 33, 5363–5375 CrossRef CAS PubMed.
- L. H. Tan, H. Xing and Y. Lu, Acc. Chem. Res., 2014, 47, 1881–1890 CrossRef CAS PubMed.
- Z. Cheng, A. Al Zaki, J. Z. Hui, V. R. Muzykantov and A. Tsourkas, Science, 2012, 338, 903–910 CrossRef CAS PubMed.
- H. Maeda, J. Controlled Release, 2012, 164, 138–144 CrossRef CAS PubMed.
- Z. Cheng, D. L. J. Thorek and A. Tsourkas, Angew. Chem., Int. Ed., 2010, 49, 346–350 CrossRef CAS PubMed.
- A. J. Silversmith, N. T. T. Nguyen, B. W. Sullivan, D. M. Boye, C. Ortiz and K. R. Hoffman, J. Lumin., 2008, 128, 931–933 CrossRef CAS.
- D. Zhang, A. Gao, Y. Xu, X.-B. Yin, X.-W. He and Y.-K. Zhang, Analyst, 2014, 139, 4613–4619 RSC.
- N. M. K. Tse, D. F. Kennedy, N. Kirby, B. A. Moffat, T. M. Hinton, B. W. Muir, R. A. Caruso and C. J. Drummond, J. Mater. Chem. B, 2013, 1, 1219–1222 RSC.
- L. Tang, X. Yang, L. W. Dobrucki, I. Chaudhury, Q. Yin, C. Yao, S. Lezmi, W. G. Helferich, T. M. Fan and J. Cheng, Angew. Chem., Int. Ed., 2012, 51, 12721–12726 CrossRef CAS PubMed.
- D. W. Hwang, H. Y. Ko, J. H. Lee, H. Kang, S. H. Ryu, I. C. Song, D. S. Lee and S. Kim, J. Nucl. Med., 2010, 51, 98–105 CrossRef CAS PubMed.
- J. Kobler, K. Moeller and T. Bein, ACS Nano, 2008, 2, 791–799 CrossRef CAS PubMed.
- Z.-A. Qiao, L. Zhang, M. Guo, Y. Liu and Q. Huo, Chem. Mater., 2009, 21, 3823–3829 CrossRef CAS.
- W.-Y. Huang, G.-L. Davies and J. J. Davis, Chem. Commun., 2013, 49, 60–62 RSC.
- J. J. Davis, W.-Y. Huang and G.-L. Davies, J. Mater. Chem., 2012, 22, 22848–22850 RSC.
- N. Portney and M. Ozkan, Anal. Bioanal. Chem., 2006, 384, 620–630 CrossRef CAS PubMed.
- M. Norek, I. C. Neves and J. A. Peters, Inorg. Chem., 2007, 46, 6190–6196 CrossRef CAS PubMed.
- J.-L. Bridot, A.-C. Faure, S. Laurent, C. Riviere, C. Billotey, B. Hiba, M. Janier, V. Josserand, J.-L. Coll, L. Vander Elst, R. Muller, S. Roux, P. Perriat and O. Tillement, J. Am. Chem. Soc., 2007, 129, 5076–5084 CrossRef CAS PubMed.
- J. J. Day, B. V. Marquez, H. E. Beck, T. A. Aweda, P. D. Gawande and C. F. Meares, Curr. Opin. Chem. Biol., 2010, 14, 803–809 CrossRef CAS PubMed.
- G. Zhu, M. Ye, M. J. Donovan, E. Song, Z. Zhao and W. Tan, Chem. Commun., 2012, 48, 10472–10480 RSC.
- Z. Ali, A. Z. Abbasi, F. Zhang, P. Arosio, A. Lascialfari, M. F. Casula, A. Wenk, W. Kreyling, R. Plapper, M. Seidel, R. Niessner, J. Knoell, A. Seubert and W. J. Parak, Anal. Chem., 2011, 83, 2877–2882 CrossRef CAS PubMed.
Footnote |
† Electronic supplementary information (ESI) available. See DOI: 10.1039/c5ay02230d |
|
This journal is © The Royal Society of Chemistry 2016 |
Click here to see how this site uses Cookies. View our privacy policy here.