DOI:
10.1039/C6CS00340K
(Tutorial Review)
Chem. Soc. Rev., 2016,
45, 5200-5215
Preparation of polyphosphazenes: a tutorial review
Received
27th April 2016
First published on 17th June 2016
Abstract
Poly(organo)phosphazenes are a family of inorganic molecular hybrid polymers with very diverse properties due to the vast array of organic substituents possible. This tutorial review aims to introduce the basics of the synthetic chemistry of polyphosphazenes, detailing for readers outside the field the essential knowledge required to design and prepare polyphosphazenes with desired properties. A particular focus is given to some of the recent advances in their chemical synthesis which allows not only the preparation of polyphosphazenes with controlled molecular weights and polydispersities, but also novel branched architectures and block copolymers. We also discuss the preparation of supramolecular structures, bioconjugates and in situ forming gels from this diverse family of functional materials. This tutorial review aims to equip the reader to prepare defined polyphosphazenes with unique property combinations and in doing so we hope to stimulate further research and yet more innovative applications for these highly interesting multifaceted materials.
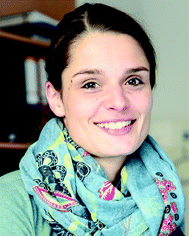
Sandra Rothemund
| Sandra Rothemund studied chemistry at the University of Bayreuth (Germany) and graduated with a MSc in 2010. She received a PhD in polymer chemistry from the Johannes Kepler University Linz (Austria) in 2014 for her work on the synthesis of functional and biodegradable polyphosphazenes for biomedical applications under the guidance of Ian Teasdale and Oliver Brüggemann. Currently, she is working on functional in vitro systems as a postdoc in the research group of James J. Hickman at the NanoScience Technology Center at the University of Central Florida (USA). |
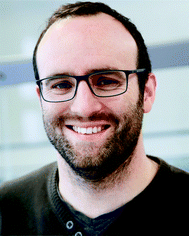
Ian Teasdale
| Ian Teasdale studied chemistry at the University of Sheffield (UK) and Heidelberg University (Germany), graduating with an MChem in 2004. In 2008 he received a PhD in chemistry from the University of Manchester (UK) for his work on the synthesis of high performance polyaryletherketones under the guidance of Michael L. Turner. A postdoc position working on functional polymers with Oliver Brüggemann took him to the Institute of Polymer Chemistry at the Johannes Kepler University Linz (Austria) where he subsequently received an assistant professorship. In 2015 he completed a habilitation in polymer chemistry. A promotion to associate professor followed with an ongoing research focus on polyphosphazene synthesis and their use as degradable polymers. |
Key learning points
(1) Poly(organo)phosphazenes are a family of inorganic molecular hybrid polymers based on a phosphorus–nitrogen backbone substituted with organic side groups which show very differing properties due to the vast array of organic substituents possible.
(2) Although the breadth of properties observed can be bewildering, the main motivation for synthesising polyphosphazenes is a small number of unique combinations of properties which make polyphosphazenes extremely interesting materials.
(3) The basic synthesis, often thought to be comparatively tricky, has for a long time hindered development, herein we aim to equip the reader with the tools to design and prepare polyphosphazenes with desired properties.
(4) In this review we also describe how recently improved chemistry allows for controlled synthesis of functional polyphosphazenes with well-defined architectures such as block copolymers and branched architectures.
|
1. Introduction
1.1 Background
Polyphosphazenes are macromolecules with a phosphorus–nitrogen backbone, substituted by two (commonly organic) side groups on the phosphorus atoms to give poly(organo)phosphazenes (Fig. 1). Polyphosphazenes themselves have a long history with cross-linked elastomeric materials (‘inorganic rubber’) consisting of phosphorus and nitrogen being reported as early as the 1890's. The isolation of soluble poly(dichloro)phosphazene [NPCl2]n, which had formed the basis of such ‘inorganic rubbers’, was first achieved in the 1960's by H. R. Allcock and coworkers.1 Key to this development was the insightful observation that the cross-linking of ‘inorganic rubber’ was caused by reaction of [NPCl2]n with trace amounts of H2O and thus, in the absolute absence of atmospheric moisture, non-cross-linked and soluble macromolecules of [NPCl2]n could be prepared. As was appreciated by H. R. Allcock et al., the reaction of H2O with [NPCl2]n was via nucleophilic attack and therefore the chlorine atoms of [NPCl2]n can also be readily replaced by organic nucleophiles to give stable poly(organo)phosphazenes, which can be processed and formed into materials with a wide variety of properties.1 Added to the fact that mixed substitution is also possible, i.e. the substitution of two or more organic substituents on the same macromolecule, this gives ready access to extensive libraries of polymers with varied properties. Indeed, small changes in the substituents can lead to considerable changes in the polymer properties, e.g. glass transition temperatures, which can vary from −100 °C to above room temperature (up to +300 °C are reported), with very minor structural changes (Fig. 2).
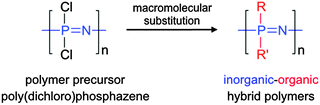 |
| Fig. 1 Generic structure of poly(organo)phosphazenes, a family of molecular hybrid polymers, most-commonly prepared from the precursor poly(dichloro)phosphazene [NPCl2]n. | |
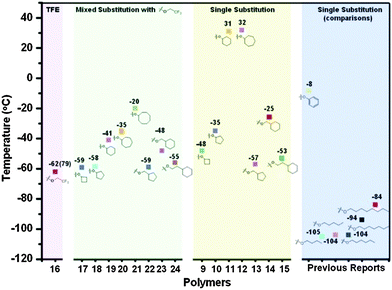 |
| Fig. 2 The effect of different substituents on the glass transition temperatures of poly(organo)phosphazenes, exemplifying how small structural changes can be used to tailor the physical properties of the resulting polyphosphazene. Reprinted with permission from Z. Tian et al., Macromolecules, 2015, 48, 4301. Copyright 2015 American Chemical Society.2 | |
This ease of tunability of poly(organo)phosphazenes, combined with the distinctive inorganic backbone, has led to significant academic interest and the development of materials with very differing characteristics, ranging from superhydrophobic to water soluble and from cationic to anionic. Such materials have been investigated for applications as far afield as vaccine delivery3 and fuel cell membranes.4 Currently, there is significant ongoing development of polyphosphazenes for potential use in the biomedical field, including as matrices for tissue engineering5 as well as drug and gene delivery,6 primarily due to their inherent backbone degradability and biocompatibility.7 Polyphosphazenes are further used in a variety of functional materials, for example as high performance elastomers,8 due to their combination of chain flexibility and high temperature stability, or as flame retardants9 due to their side chain functionality and high limiting oxygen indices. For a detailed overview on the application of polyphosphazenes as soft materials, the reader is referred to a review article recently published elsewhere.8 Furthermore, a detailed and highly comprehensive review of the field, including a summary of the majority of the poly(organo)phosphazenes produced up to 2003, has been published by H. R. Allcock1 and thus the reader is referred here for further information on the historical works. The focus of this review is laid on more recent developments and above all in synthetic methods for the preparation of polyphosphazene based materials.
1.2 Molecular level hybrids
Poly(organo)phosphazenes can be thought of as ‘molecular level hybrids’ with the effects of the organic substituents dictating the properties of the inorganic backbone and vice versa. The organic component has a defining role in terms of the chemical properties and thus the solubility and functionality of the resulting polymer, as well as hydrolytic stability (hence degradability). The inorganic backbone itself offers unique properties and is clearly more than just an inert scaffold, determining the size, architecture and conformation of any hybrid polymer formed. It is important to note that the P–N backbone has an unusual form of bonding, almost unique in chemistry and a detailed understanding of the bonding remains elusive.10 Due to its unique skeletal-bonding structure, it is not unsaturated, quite different from electron rich organic systems, nor is it delocalised. Indeed, far from being conjugated, the free rotation around the P–N bonds is extremely low which translates into the experimental observations that the linear phosphazene backbone can be quite flexible (clearly depending on the nature of the organic substituents).
2. Synthesis of polyphosphazenes
2.1 Macromolecular substitution of [NPCl2]n
Although not exclusive, by far the most widely used route to poly(organo)phosphazenes is via the precursor poly(dichloro)phosphazene [NPCl2]n (Fig. 3). Due to its high reactivity, this intermediate can only be isolated under anhydrous conditions and must be carefully stored or immediately reacted further. Recently, however, significant progress has been made in this regard, with the discovery that [NPCl2]n can be readily stored in solution in the presence of diglyme.11 Although hitherto unclear how, the remarkable stabilisation effect enables storage of [NPCl2]n in solution for several years, whereby previously hydrolysis and/or cross-linking would have been expected within hours.
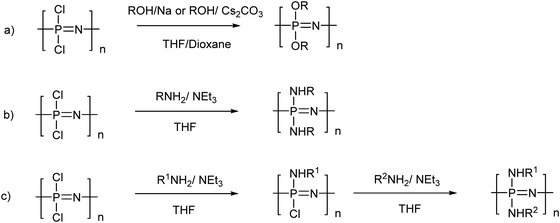 |
| Fig. 3 The most common routes to poly(organo)phosphazenes via macromolecular substitution of the reactive precursor poly(dichloro)phosphazene using alkoxides or aryloxides (a) and primary amines (b). Scheme (c) depicts a mixed substitution using different nucleophiles which allows fine-tuning the properties of the polymer. | |
In the absence of H2O, the high reactivity of [NPCl2]n in turn allows complete post-polymerisation with mono-functional nucleophiles, assuming functional group tolerance (e.g. no free acid groups). This facile post-polymerisation substitution of [NPCl2]n to produce the final poly(organo)phosphazenes has been coined “macromolecular substitution”1 and although enabling the simple preparation of a wide range of polymers from a single precursor, such a macromolecular reaction should not be underestimated since large numbers of simultaneous reactions on a single macromolecule are required and reaction conditions and times can greatly vary depending on the nucleophilicity and steric hindrance of the substituent.2 Furthermore, it is important to ensure complete replacement of Cl atoms, not only for the sake of purity, but also due to the high susceptibility of the P–Cl bond to hydrolysis and the subsequent uncontrolled cross-linking and/or degradation that may occur should any unreacted Cl groups be left remaining on the final polymer. 31P{1H} NMR spectroscopy allows relatively simple tracking of the macromolecular substitution reaction (Fig. 4b and c), whereby a single peak corresponding to [NPCl2]n at −18 ppm should completely shift to the substituted polymer (although caution should be exercised due to the limits in sensitivity of NMR spectroscopy and the possibility of impurities in which the backbone phosphorus is monosubstituted).2
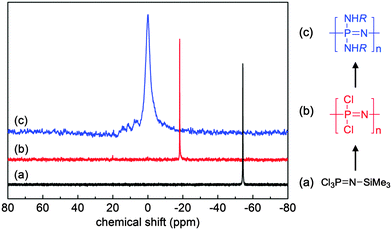 |
| Fig. 4 Sample 31P{1H} NMR spectra of the monomer trichlorophosphoranimine Cl3PNSiMe3 (a), polydichlorophosphazene [NPCl2]n (b) and a macrosubstituted poly(organo)phosphazene [NP(NHR)2]n (c) demonstrating the simple tracking of the reaction progression. Adapted from H. Henke et al., J. Polym. Sci., Part A: Polym. Chem., 2013, 51, 4467. Copyright 2013 Wiley Periodicals, Inc.12 | |
Mixed substitution, usually via stepwise addition of different nucleophiles, gives simple access to an even broader range of materials, what are essentially random copolymers (Fig. 3c). When conducting such substitution reactions, one should be aware that substituent exchange reactions can also occur, thus R1 may be displaced by R2 to some extent, depending on the nucleophilicity and steric hindrance of the reagents.13
Poly(alkyl/aryl)phosphazenes [NPR2]n with the organic substituent directly attached via a P–C bond from [NPCl2]n can be synthesised via substitution with suitable organic nucleophiles such as RMgX or RLi. However, such reactions are often tricky due to the low functional group tolerance and thus direct methods are preferred (see Section 2.3).
2.2 Polymerisation methods of [NPCl2]n
2.2.1 Ring-opening polymerisation of [NPCl2]3.
Several approaches are described in the literature to synthesise poly(organo)phosphazenes, however, there are two main routes most commonly used to obtain the polymeric precursor [NPCl2]n. The classical route to prepare linear, high molecular weight [NPCl2]n is via the ring-opening polymerisation of hexachlorocyclotriphosphazene [NPCl2]3 (Fig. 5). This reaction is usually carried out under vacuum in a sealed glass tube at high temperatures; typically 250 °C for several hours (see pages 146–153 in ref. 1). At temperatures around 250 °C, chlorine atoms can be cleaved from [NPCl2]3 to give a cationic phosphazenium species which initiates the ring opening of a second ring, thus propagating the polymerisation (Fig. 5). This reaction can be carried out on relatively large scales, indeed small scale <10 g reactions are often plagued with low reproducibility, presumably due to the relative effect of contaminants at lower scales or activity from the vessel surface. Efficient temperature control is essential for successful polymerisation since Cl cleavage is minimal at temperatures far below 250 °C and thus polymerisation rates are infinitively slow. Meanwhile, at temperatures above 250 °C, significant cross-linking occurs. The reaction temperature can be moderately lowered to around 200 °C by the addition of catalytic amounts of Lewis acids, most commonly anhydrous AlCl3. A potentially more convenient solution state method is also possible using trichlorobenzene as a solvent at 214 °C, with CaSO4·2H2O as a promoter and HSO3(NH2) as a catalyst.14 Furthermore, a direct synthesis from the [NPCl2]3 precursors phosphorus pentachloride (PCl5) and ammonium chloride has been developed,14 although the sublimation of PCl5 can become problematic at the high temperatures required for the ring-opening.
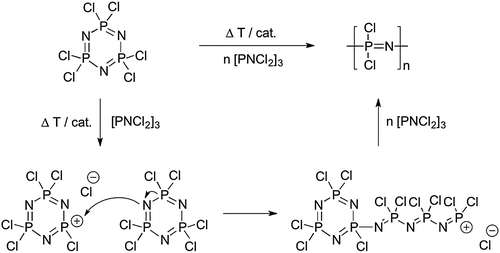 |
| Fig. 5 Thermally and/or catalytically induced ring opening polymerisation representing the classical route to synthesise poly(dichloro)phosphazene [NPCl2]n and its commonly accepted mechanism. | |
The molecular weights of the ring opening routes are usually high and difficult to control and the polydispersities are broad, primarily due to the underlying initiation mechanism (Fig. 5) in which Cl cleavage in [NPCl2]3 (and thus initiation of new polymer chains) can occur throughout the polymerisation. Furthermore, uncontrolled chain branching (especially at higher conversions) is an inherent feature of this polymerisation,11 due to Cl cleavage from the growing [NPCl2]n chains, thus producing initiation sites along the backbone. Since this leads to cross-linked species at higher conversions, the reaction must be terminated before gelation occurs (a time point often difficult to define), leading to low yields (ca. 40%). Recent work showed that full conversion could be achieved via a catalysed room temperature ring opening polymerisation of [NPCl2]3 in 1,2-dichlorobenzene.15 The key to this was the use of silylium ions (SiEt3+) to form the required phosphazene cations in combination with a weakly coordinating carborane anion. Complete conversion of [NPCl2]3 to [NPCl2]n was observed, and if optimised, this proof of principle would be an extremely useful and mild route to high molecular weight [NPCl2]n.
2.2.2 Living cationic polymerisation.
Controlled polymerisations have become of utmost importance in polymer chemistry, allowing access to block copolymers and more complex molecular structures and thus forming the basis of macromolecular engineering and modern polymer nanotechnology. For this purpose, defined molecular weights, narrow molecular weight distributions (Mw/Mn) and control over the precise nature of the polymer end groups are required, in order to be able to adapt functionality and/or to carry out further modifications. Controlled polymerisation of [NPCl2]n is possible via a living cationic polymerisation of trichlorophosphoranimine (Cl3PNSiMe3). This can be carried out in solution at room temperature via reaction of Cl3PNSiMe3 with two equivalents of PCl5 giving a cationic species [Cl3PNPCl3]+ with PCl6− as the counterion (Fig. 6a).15 This species can initiate the polymerisation upon addition of further equivalents of Cl3PNSiMe3 leading to polymer chains with a “living” cationic end group.16 One equivalent of ClSiMe3 is formed as a side product with every monomer molecule added to the polymer, making this polymerisation a rare example of a polycondensation reaction which occurs via a chain growth mechanism (compared to most polycondensation reactions having step-growth mechanisms). The living chain growth mechanism, with one cationic initiator per propagating chain, allows not only control of molecular weight via the feed monomer to initiator ratio, but also leads to poly(dichloro)phosphazenes with narrow polydispersities. This process can be readily followed by 31P NMR spectroscopy (Fig. 4a and b), confirming the consumption of Cl3PNSiMe3 and its incorporation into the polymer chain. Reaction times may vary depending on the desired polymer chain length, the monomer concentration and the nature of the counter ion,16 but Cl3PNSiMe3 is observed to be consumed completely relatively quickly within a few hours in the preferred solvent dichloromethane.17
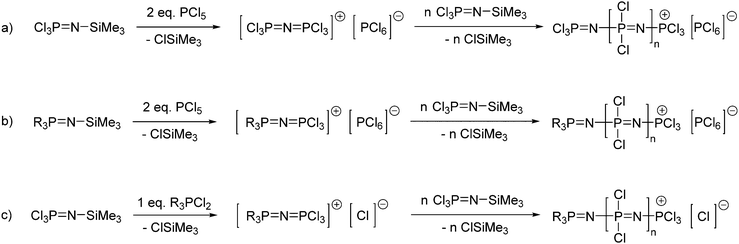 |
| Fig. 6 Synthesis of poly(dichloro)phosphazene [NPCl2]n by living cationic polymerisation of trichlorophosphoranimine (Cl3PNSiMe3) via initiation with PCl5 (a), incorporation of alkyl or aryl substituted phosphoranimines for monodirectional growth (b) and phosphine-mediated polymerisation (c). | |
The living cationic polymerisation requires large amounts of the monomer Cl3PNSiMe3 of high purity, usually prepared via reaction of PCl3 with LiN(SiMe3)2 to form Cl2PN(SiMe3)2, followed by oxidation with the chlorinating agent SO2Cl2 to give Cl3PNSiMe3. An optimized high yield synthesis route from the Manners group allows a two-step but one-pot preparation in scales of 50–100 g in yields of >80%.18 However, the purification of Cl3PNSiMe3via vacuum distillation can still be problematic on larger, more industrial scales, and thus to circumvent this, a one-pot method to produce [NPCl2]n directly via in situ preparation and polymerisation of Cl3PNSiMe3 has been suggested.19 This alternative route could facilitate upscaling and the industrial preparation of polyphosphazenes, as it avoids the vacuum distillation of the monomer. However, there is some loss in molecular weight control and thus the obtained polyphosphazenes show slightly broader polydispersities as compared to the living cationic polymerisation.
When PCl5 is used to form the cationic initiator for the living polymerisation of Cl3PNSiMe3 (Fig. 6a), some bidirectional growth can be observed due to the ability of the cationic propagating site to migrate.20 Furthermore, both end groups are identical after macrosubstitution of the chlorine atoms, thus limiting the options in terms of the preparation of polymers with higher architectures. However, monodirectional growth can be achieved via the use of R3PNSiMe3 type moieties (Fig. 6b).21 Reaction of such phosphoranimines with two equivalents of PCl5 gives a cationic species capable of initiating the polymerisation of Cl3PNSiMe3via an identical mechanism. The R groups, typically phenyl groups, effectively block one end of the initiating species, forcing the polymerisation to proceed in only one direction and more importantly resulting in polymers with defined chain ends.
Another alternative route to polyphosphazenes with defined chain ends is provided by the initiation with chlorinated tertiary phosphines R3PCl2 (Fig. 6c). Tertiary phosphines, for example Ph3PCl2, are known to exist completely in an ionized form [Ph3PCl]+ [Cl]− in polar solvents such as dichloromethane. This cationic species can be used to initiate the living cationic polymerisation of Cl3PNSiMe317,22 thus enabling monodirectional growth of the polymer and leading to controlled chain growth and narrow polydispersities. Furthermore, it has been shown that the synthesis of well-defined polyphosphazenes with end group functionalities is also possible through the use of monofunctionalised tertiary phosphines, i.e. bearing a single functional group on one of the organic moieties.17 Such monofunctionalised phosphine compounds are often commercially available due to their widespread use as ligands in inorganic catalysis and thus the spectrum of functional end groups readily available is manifold.
The cationic polymerisation routes show excellent control of the molecular weight for shorter polymer chain lengths (up to approximately 50 repeat units) and narrow molecular weight distributions (Mw/Mn) are observed. Thereafter control decreases at higher degrees of polymerisation (DP), presumably due to the prevalence of side reactions.17 Furthermore, the DP's obtained via the cationic polymerisation routes are significantly lower than via the ring opening polymerisation of [NPCl2]3, whereby DP's are commonly reported to be >10
000, and as such the ring-opening method remains of importance for applications where higher molecular weights are required, but control of molecular structure is of lesser importance.
2.3 Direct synthesis routes to poly(organo)phosphazenes
It is also possible to directly synthesise poly(organo)phosphazenes, without the need for the [NPCl2]n precursor. An example here includes the anionic polymerisation of N-silylphosphoranimines with fluoride ion initiators in the presence of N-methylimidazole (Fig. 7). Without quite achieving the control of the cationic routes described above, this polymerisation has living character and enables the preparation of polymers with polydispersities between 1.3 and 2.3 at 125 °C.23 Recently Steinke et al.24 showed that the polymerisation of similar N-silylphosphoranimines can be initiated by H2O with a catalytic amount of N-methylimidazole with living polymerisation kinetics (n ≈ 100 and dispersity ≤1.15). This method was proven to be robust for the preparation of poly(bistrifluoroethoxy phosphazene) and thus, if extended to a wider range of monomers, this could be a valuable route to prepare poly(organo)phosphazenes with excellent control of molecular weight and polydispersity.
 |
| Fig. 7 Direct synthesis route to N-silylphosphoranimines via anionic polymerisation. | |
Poly(alkyl/aryl)phosphazenes, [NPR2]n, whereby the organic substituent is directly attached via a P–C bond, can also be prepared via direct polymerisation routes. Initially developed by thermal (100–180 °C) condensation of (CF3CH2O)R2P
NSiMe3 type monomers,25 this has since been expanded to cationic initiators and with monomers of the type BrR2P
NSiMe3.21 Recently, the polymerisation of halo(alkyl/aryl)phosphoranimines (XR2PNSiMe3, X = Br or Cl) initiated by organic phosphites ((MeO)3P) has also been shown to be an effective route to poly(alkyl/aryl)phosphazenes at ambient temperature (Fig. 8).15 Furthermore, such phosphoranimines can be combined with the cationic polymerisation of [NPCl2]n (Fig. 9), thus allowing the preparation of block copolymers as will be discussed in the next section.
 |
| Fig. 8 Direct synthesis route to poly(alkyl/aryl)phosphazenes, with R and R′ = alkyl, aryl, typically R = Ph and R′ = Me, X = Br or Cl. | |
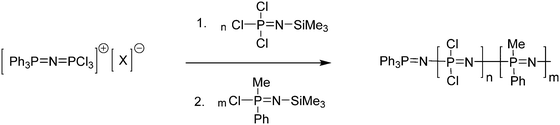 |
| Fig. 9 Exemplary synthesis pathway for the preparation of block copolymers with poly(dichloro)phosphazene and poly(alkyl/aryl)phosphazene. | |
2.4 Preparation of block copolymers
Although the macromolecular substitution of [NPCl2]n gives ready access to copolymers, they are exclusively random copolymers because the nucleophilic substitution of the chlorine atoms along the polyphosphazene backbone is non-selective. Block copolymers, however, have widespread use and have proven essential for a wide number of applications, thus a considerable effort has been made in order to be able to also prepare block copolymers of polyphosphazenes. Due to living nature of the cationic polymerisation routes to [NPCl2]n, described above (Fig. 6), the polymer chain ends remain active and enable further polymerisation upon addition of a second monomer. Clearly, due to the macrosubstitution method, all repeat units are however then substituted to yield the same poly(organo)phosphazene. One option to circumvent this is the combination of the cationic polymerisation of Cl3PNSiMe3 (Fig. 9) with a second block using a phosphoranimine of the type ClR2P
N-SiMe3, resulting in one “tunable” block of [NPCl2]n, with its wide array of possible substituents, followed by a polyalky/arylphosphazene block.26
Block copolymers combining polyphosphazene blocks with blocks from a second polymer can also be synthesised, for example, the organometallic–inorganic block copolymer poly(ferrocenylsilane-b-polyphosphazene),22 prepared via end group functionalisation of PFS with diphenylphosphine groups which could then act as a macroinitiator for the cationic polymerisation of Cl3PNSiMe3. Furthermore, a number of block copolymers with organic polymers have been reported using phosphoranimines as capping agents to modify an organic polymer which can then undergo macromolecular coupling with a “living” poly(dichloro)phosphazene (Fig. 10). An example includes the capping of amine functionalised polylactides with bromophosphoranimine,27 however, the same synthesis principle can and has been applied to a wide range of organic polymers.
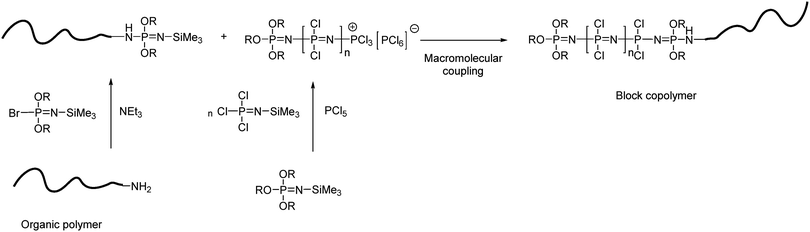 |
| Fig. 10 Representative synthetic route to polyphosphazene block copolymers with organic polymers. | |
3. Polyphosphazenes with advanced architectures
3.1 Graft polymers with organic side-chains
The use of controlled polymerisation techniques allows the synthesis of polymers with defined chain length, composition and more complex architectures based on polyphosphazenes. In this regard, the living condensation method described in the previous section can also be used to end-cap polyphosphazenes with polymerizable groups that can then be used to grow organic polymer chains, also described as telechelic polymers. An example here includes the capping with norbornenyl functionalised phosphoranimines which can undergo ring-opening metathesis polymerizations28 in essence polynorbornenes with grafted phosphosphazene pendent side arms.
The opposite procedure, i.e. grafting of organic polymers onto [NPCl2]n, with its two functional groups per repeat unit, allows relatively simple access to highly dense molecular brush-type polymers.12 Alternatively, it is possible to functionalise the backbone with macroinitiators for a ‘grafting from’ approach, as exemplified by the functionalisation with alkyl bromides capable of initiating vinyl polymerisations emanating from the inorganic backbone via atom transfer radical polymerisation (ATRP) (Fig. 11). This method was used to prepare a series of densely grafted molecular brushes composed of polystyrene, poly(tert-butyl acrylate), and poly(N-isopropylacrylamide).29 Conversely, the cationic polymerisation route allows the grafting of polyphosphazenes onto multifunctional polymers, as was illustrated by the grafting of [PN(OCH2CF3)2]n onto phosphoranimine-terminated PAMAM dendrimers, to give dendrimers consisting of an organic core and polyphosphazenes grafted onto the end of each arm.8
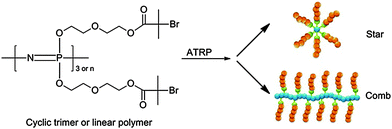 |
| Fig. 11 Synthesis of grafted star-like and comb-shaped molecular brush hybrid poly(organo)phosphazenes via ATRP. Reprinted with permission from X. Liu et al., Macromolecules, 2012, 45(3), 1417. Copyright 2015 American Chemical Society.29 | |
3.2 Polyphosphazenes with a branched polyphosphazene backbone
Previously reported examples of ‘branched or dendritic’ polyphosphazenes are in fact based on organic polymers, with polyphosphazenes grafted to or from the branched unit. However, a synthetic route to prepare polymers with a polyphosphazene-based branched structure has also recently been developed (Fig. 12).30 This method utilises star-like polyphosphazenes (generation 1) substituted with diphenylphosphine moieties. These can act as macroinitiators for the simultaneous polymerisation of a second generation of many arms to produce highly branched polyphosphazenes (dendritic molecular brushes) with high degrees of polymerisation but relatively narrow molecular weight distributions.
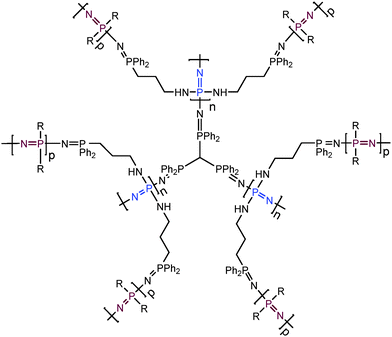 |
| Fig. 12 Chemical structure of a polyphosphazene-based dendritic molecular brush, consisting of a star polyphosphazene (generation 1, ‘n’, shown in blue) which was used as a macroinitiator to grow polyphosphazene arms (‘graft from’, generation 2, ‘p’, shown in purple). | |
3.3 Supramolecular structures from polyphosphazenes
The formation of supramolecular structures, for example, polymer micelles and polymersomes, have generated significant interest in recent years. Although commonly made from amphiphilic block copolymers, it is possible to prepare micelles and polymersomes from randomly substituted poly(organo)phosphazenes.31 The ability of poly(organo)phosphazenes to form such stable aggregates is presumably due to the conformational flexibility of the polyphosphazene backbone, allowing aggregation of the hydrophobic moieties (Fig. 13). The high functionality of the poly(organo)phosphazenes leads inherently to a wide scope of supramolecular structures with tailored surface properties. One recent example includes poly(organo)phosphazenes with alkene/alkyne organic substituents for the subsequent preparation of highly functionalised polymers via thiol–ene/yne addition chemistry.32 In this example, the polymers were then decorated with glycosyl moieties to synthesise amphiphilic poly(organo)phosphazenes, which upon self-assembly could undergo specific interactions via their surface ligands.
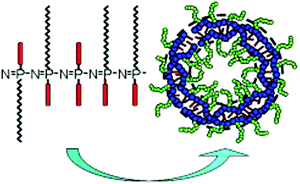 |
| Fig. 13 Schematic illustration of amphiphilic poly(organo)phosphazenes with the ability to form micellar and polymersome-like aggregates. Reproduced with permission from C. Zheng et al., Polymer, 2009, 50(5), 1173. Copyright 2009 Elsevier.31 | |
The recently developed methods for the controlled growth of polyphosphazene chains in one-direction opens many opportunities in terms of the preparation of tailored advanced nanomaterials. A prime example here is reported by Soto et al.33 who utilize the crystallization-driven living self-assembly of poly(ferrocenylsilane) (PFS) in combination with flexible polyphosphazenes, leading to the formation of stable pointed-oval shaped micelles.33 The idealized graphic representation (Fig. 14) shows polyphosphazene coronas in red and the body of the PFS oval in yellow. The centre consists of cylindrical micelles of PFS34–P2VP272 (P2VP = poly(2-vinylpyridine)) that were used as seed micelles to initiate the self-assembly into this unusual hierarchical structure.
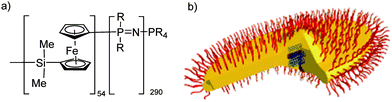 |
| Fig. 14 Chemical structure of the block copolymer consisting of poly(ferrocenylsilane) and polyphosphazene (R = OCH2CF3) blocks (a) and an idealized graphic representation of the pointed-oval-shaped micelles after crystallization-driven living self-assembly (b). Adapted with permission from A. Presa Soto et al., Angew. Chem., Int. Ed., 2010, 49, 8220. Copyright 2010 Wiley-VCH.33 | |
The ability to grow block copolymers from polyphosphazenes clearly opens many avenues for macromolecular engineering. Another interesting example here includes the preparation of polyphosphazene block copolymers which can self-assemble to supramolecular aggregates via host–guest inclusion complexes with cyclodextrin (Fig. 15).34 In this example [NPCl2]n chains were grown on an amine-capped polystyrene, which upon substitution of the [NPCl2]n with adamantyl groups, gave a hydrophobic diblock copolymer. The adamantyl group is known to undergo efficient host–guest complexation with cyclodextrin (β-CD) and it was shown that this can also be achieved on the polyphosphazene backbone. Upon mixing with aqueous solutions of β-CD, the block copolymers could be solubilized to micelles at relatively low critical micelle concentrations due to complexation of the adamantyl groups increasing the hydrophilicity of the polyphosphazene block and effectively forming an amphiphilic block. Although themselves not biodegradable, this system represents a proof of principle towards the development of fully degradable micelles if it can be extended to degradable polyphosphazene blocks and replacement of the polystyrene with a bioerodible polymer.
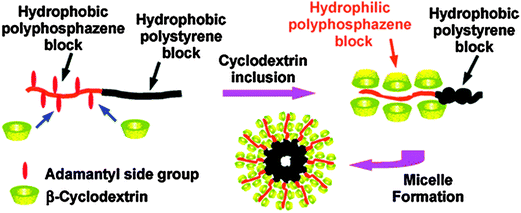 |
| Fig. 15
In situ formation of amphiphilic polystyrene–polyphosphazene diblock copolymers via host–guest inclusion complexes with β-cyclodextrin can be used to prepare supramolecular aggregates. Reprinted with permission from S. Y. Cho and H. R. Allcock, Macromolecules, 2009, 42, 4484. Copyright 2009, American Chemical Society.34 | |
3.4 Helical structures with polyphosphazenes
Helical architectures are well-known and essential structures in nature, such as in DNA and α-helical motives in proteins, hence it is unsurprising that there is much interest in recreating helical structures in synthetic polymers. For this purpose, high molecular weight, spirocyclic polyphosphazenes have been synthesised via macromolecular substitution of [NPCl2]n with binaphthoxy moieties to yield the chiral poly(2,2′-dioxy-1,1′-binaphthyl)phosphazene (Fig. 16)35 showing a preferred helical sense in solution and solid state. This polymer is itself intriguing, as the enforced geometry allows complete chlorine replacement of the macromolecular precursor without cross-linking that might be expected from the reaction of [NPCl2]n with a diol. The glass transition temperature Tg (∼330 °C) is extraordinarily high for a polyphosphazene which is attributed to the hindered rotation conferred from the binaphthyl substituents. This hindered bond rotation, or atropisomerism, results in polymers which, although behaving as random coils, can form helix-type sequences and short helices in sections of the polymer chain. Through the preparation of block copolymers with [NPMePh]n, it has also been shown that the helical conformation of the chiral block can induce a preferential helical conformation in the non-chiral block35 and that chirality of the helical centre can be transferred upon self-assembly to prepare nanostructures with helical morphologies.26
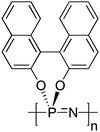 |
| Fig. 16 Chemical structure of the chiral poly(2,2′-dioxy-1,1′-binaphthyl)phosphazenes forming a helical conformation. | |
Poly[di(4-carboxyphenoxy)phosphazene] (PCPP) (Fig. 17) is a flexible achiral polymer and is as such optically inactive. Remarkably, however, PCPP has been shown to have a large optical rotation upon complexation with optically active (R)- or (S)-1-phenylethylamine, transforming into a prevailing one-handed helical conformation (see ref. 36 and references therein), thus acting as dynamic helical polymers. The feature of the dynamic helicity is presumably a function of the high flexibility of the unique polyphosphazene backbone facilitating conformational alignment. These polymers are proposed for numerous applications as chiral materials, for example the use as “chiral filters”36 for enantioselective adsorption of racemic amines (Fig. 17).
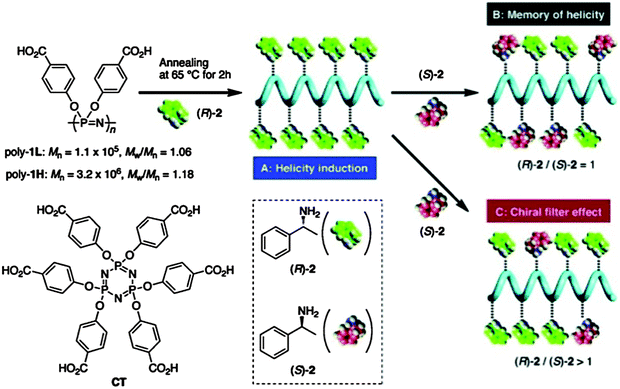 |
| Fig. 17 Dynamic helicity of poly[di(4-carboxyphenoxy)phosphazene] and its application as a chirality filter. Note: the polymer referred to as Poly1L here is essentially PCPP, a well-known polyphosphazene investigated for use in medical applications. Reprinted with permission from K. Maeda et al., Macromolecules, 2011, 44, 2457. Copyright 2015 American Chemical Society.36 | |
4. Hexachlorocyclotriphosphazene as a building block
A significant amount of literature is dedicated to the preparation of novel materials based on the cyclic phosphazene trimer hexachlorocyclotriphosphazene [NPCl2]3 (sometimes referred to as HCCP) and the preparation of high molecular weight materials through coupling the rings with multifunctional organic nucleophiles. The ease of accessibility of the cyclic [NPCl2]3 makes this a particularly attractive preparation route to include the phosphazene unit into polymers. Covalent linkage of the cyclic [NPCl2]3 can be used to prepare cyclolinear or cyclomatrix, as well as dendritic structures. Cyclolinear structures require only two reactive sites from the six possible in the cyclic [NPCl2]3 unit, which can be a synthetic challenge, in order to avoid cross-linking but nevertheless numerous examples have been prepared.1 Reaction of more than two sites leads to cross-linked, so called cyclomatrix materials, which have shown some recent progress and are thus highlighted in the following section.
4.1 Cyclomatrix polyphosphazenes
Precipitation polycondensation, that is the in situ formation of an insoluble polymer matrix, can be readily carried out with the cyclic [PNCl2]3 through nucleophilic substitution with multifunctional organic nucleophiles (Fig. 18). This gives a range of unique functional materials termed ‘cyclomatrix’ polyphosphazenes. Such an approach utilizes the high reactivity of the P–Cl bonds toward nucleophilic substitution, as well as the high number of functional groups. Upon substitution, some chlorine moieties will be left, depending on the reaction conditions. In situ processing of the final product is clearly required for such highly cross-linked materials and attention must be paid to the removal of by-products.
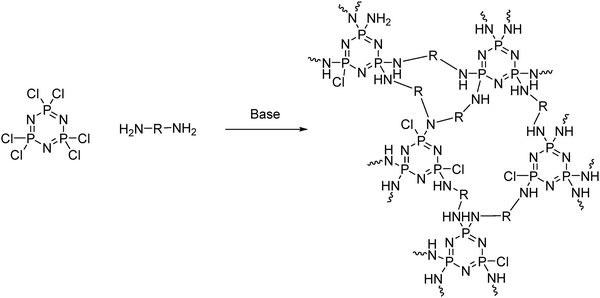 |
| Fig. 18 An example synthesis route to cyclomatrix polyphosphazenes using hexachlorocyclotriphosphazene [NPCl2]3 as a building block. | |
In recent years, clever engineering has evolved the precipitation polycondensation method to prepare cyclomatrix polyphosphazenes with uniform advanced structures such as nano/microspheres and nanotubes.37 Both the substitution reaction and the precipitation process influence the chemical structure as well as the size and shape of the products. Most of the chemistry has been developed with aromatic organic moieties whose rigid structures have the required mechanical properties to hold the scaffold together. More recently the approach has also been extended to flexible aliphatic monomers, for example with multifunctional branched polyethylenimines, to prepare more flexible, less rigid materials with further increased functionality.38 This brings several advantages including higher functionality and higher degrees of swelling. Recently, Huang et al. were able to prepare amino acid ester based phosphazene particles via such precipitation condensation chemistry.39 The prepolymer/oligomer formation involves the reaction of [PNCl2]3 with the diamine cystine in acetonitrile (good solvent) followed by particle formation (and curing) upon addition of H2O as a poor solvent for the system.39 This “water triggered self-assembly” leads to precisely controlled uniform spheres with sizes ranging from 250 nm to 2 μm by adjusting the monomer concentration (Fig. 19).
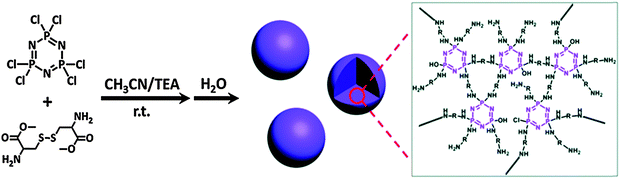 |
| Fig. 19 An example of a precipitation condensation reaction to give cyclomatrix polyphosphazenes using [PNCl2]3 as a building block. Adapted from Z. Huang et al., Chem. Commun., 2015, 51, 8373 with permission from The Royal Society of Chemistry.39 | |
4.2 Dendrimers from hexachlorocyclotriphosphazene
The multiplicity and reactivity of hexachlorocyclotriphosphazene [PNCl2]3 has also been explored for its effective use as a synthon in dendrimer design, exerting a multiplying effect to the dendrimer due to its six easily functionalized groups. The original approach here was to react [PNCl2]3 with six diamines, followed by six [PNCl2]3 molecules and so forth. However, due to the problems of cross-linking and/or high excess of reagents, more elegant approaches have been developed, involving substituted cyclic phosphazenes with orthogonal functionalities. Although many combinations are possible, the synthetically easiest to reach in a controlled manner are the substitution of one chloride with a given functionality, followed by five orthogonal substituents (or vice versa) giving AB5 type units. These AB5 type units give simple access to synthons each offering five branching sites and thus a significant multiplying effect compared to most organic units (which commonly have 2–4 branching sites).41 An example here is shown in Fig. 20,40 in which two AB5 type substituted [PNCl2]3 moieties are prepared having azide/aldehyde functionalities capable of coupling to a amine/phosphine functionalised AB5 monomer in an orthogonal manner. This elegant route allows the preparation of a third generation (i.e. three distinct steps) dendrimer with 750 functional end groups. Similar chemistry and the potential of this multiplying effect has been put to great use to prepare a range of well-defined dendritic structures with some properties quite unique from many comparable organic dendrimers. The properties and applications of such phosphazene-based dendrimers have been reviewed recently.40
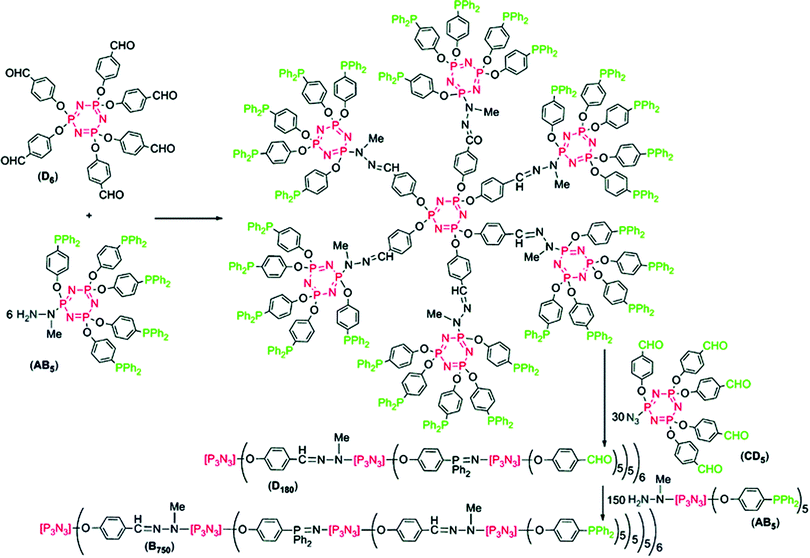 |
| Fig. 20 An example of a highly branched dendrimer exploiting the cyclic phosphazene unit to insert a multiplying effect on the branching structure with an AB5 type phosphazene based synthon on a hexachlorocyclotriphosphazene core. Reproduced from A.-M. Caminade et al., Dalton Trans., 2016, 45, 1810 with permission from The Royal Society of Chemistry.40 | |
5. Formation of bioconjugates with polyphosphazenes
One of the most outstanding properties of poly(organo)phosphazenes is the ability of its polyacid/polyanionic analogues to bind to biomacromolecules, a property that has wide-ranging potential in biomedicine. A variety of polyphosphazene based polyacids can be prepared. Direct substitution of [NPCl2]n with the free carboxylic acids is not possible, due to the reaction with the backbone, so alkyl esters are used followed by deprotection under basic conditions. The sodium salt of the polyacids undergo strong non-covalent interactions with biomacromolecules (Fig. 21). Their ability to bind to antigenic proteins has been shown to be far superior to similar anionic polymers based on carbon backbones, due to the high density of binding sites, the higher flexibility of the backbone and the high conformational adaptability this infers.42 In works pioneered by A. K. Andrianov and co-workers, the lead compound PCPP poly[di(carboxylatophenoxy)phosphazene] has undergone exhaustive testing into its production,11 hydrolytic degradation43 and toxicology (p. 54 in ref. 44) and it has been tested with a diverse range of antigens both in vitro and in vivo in mice, large animals and indeed human clinical trials.44 Standard adjuvant formulations based on anionic aluminium salts suffer some disadvantages which could be overcome by using polymer-based adjuvants, not least, for example, for the formation of mechanically stable films. In this regard, it has been shown that PCPP can be formulated into microspheres for drug loading or polymer-coated microneedles for the preparation of skin-patches for facile vaccine administration (Fig. 22).3
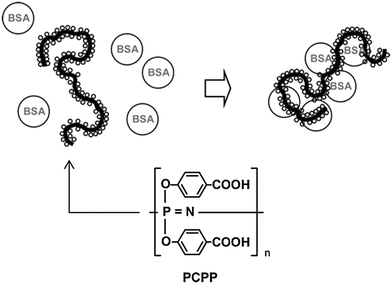 |
| Fig. 21 A schematic representation of PCPP, a polyphosphazene-based polyacid, and the proposed conjugation with BSA (bovine serum albumin). Reprinted from A. K. Andrianov et al., Biomacromolecules, 2005, 6, 1375. Copyright 2005 American Chemical Society.42 | |
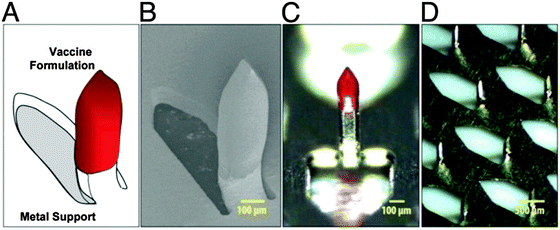 |
| Fig. 22 Polyphosphazene coated microneedles for intradermal adjuvant administration: schematic representation (A), scanning electron microscopy image (B), optical microscopy images (C and D). Reproduced with permission from A. K. Andrianov et al., Proc. Natl. Acad. Sci. U. S. A., 2009, 106(45), 18936.3 | |
In a similar vein, cationic polyphosphazenes, usually substituted with quaternized tertiary amino groups, can bind to anionic biomacromolecules45 (also reviewed in ref. 6). Investigations of various positively charged polyphosphazenes for gene delivery due to their ability to bind to negatively charged DNA forming polyplexes (sometimes referred to as “nanoparticles” in the literature on the basis of them inherently falling under the ‘nano’ size range of around 100 nm). Interestingly, poly(methylamino)phosphazene is observed to be cationic at physiological pH and its bioconjugation to DNA has been studied in detail.45
6.
In situ gelling polyphosphazenes
As discussed earlier (Section 2.4), the macrosubstitution route allows the facile preparation of random block copolymers. One way this synthetic approach can be put to effective use is to prepare amphiphilic polymers which possess a lower critical solution temperature (LCST) transition in aqueous environments. This phenomenon leads to collapse of the polymer chains above the LCST and thus a temperature-triggered agglomeration, followed by precipitation and/or gelation. If the LCST is around or just below body temperature, such triggered phase transitions can be of particular interest for biomedical applications, including drug delivery and tissue engineering. This can be achieved for polyphosphazenes by simple macrosubstitution of the polyphosphazene backbone with organic groups known for their thermoresponsive behaviour, for example, PNIPAm oligomers. Alternatively, it is possible to prepare thermosensitive poly(organo)phosphazenes by co-substitution of the inorganic backbone with hydrophobic and hydrophilic groups. This has been reported in various guises using hydrophobic amino acid esters (most commonly L-isoleucine ethyl ester, co-substituted with hydrophilic PEG oligomers as shown in Fig. 23) in which hydrophobic interactions of the amino acid esters lead to expulsion of H2O and thus precipitation and/or gelation above the LCST (Fig. 24).
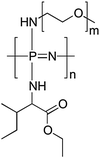 |
| Fig. 23 Representative structure of a typical thermosensitive poly(organo)phosphazene. The ratio of the hydrophobic isoleucine ester versus the hydrophilic polyethylene glycol units can be used to tailor the solution properties of the polymers. Further co-substitution is used to tune the mechanical and degradation properties. | |
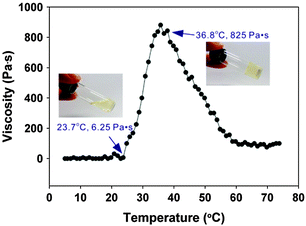 |
| Fig. 24 Temperature-dependent sol–gel transition and viscosity change of a suitably functionalised poly(organo)phosphazene. Adapted with permission from Y.-M. Kim et al., ACS Nano, 2012, 6, 5757. Copyright 2015 American Chemical Society.46 | |
A large amount of literature has been produced in the last 15 years (mainly by S.-C. Song and co-workers) focussing on the modification and optimization of the mechanical properties and degradation rates of such amphiphilic, thermosensitive poly(organo)phosphazenes, as well as their medical application, in particular the use as injectable hydrogels for drug delivery and theranostics with a wide range of drugs and therapeutic applications being investigated (see ref. 46 and 47 and references therein). Based on variations of the PEG/isoleucine ethyl ester substituted poly(organo)phosphazenes (Fig. 23), further co-substitution of the multivalent [PNCl2]n can be carried out to tailor the degradation rate and to improve the mechanical stability of such hydrogels, for example, by the addition of photocrosslinkable groups or to covalently bind drugs or biologically active agents. The tunability of the materials, combined with the proven biocompatibility and degradability in combination with the ability to gel rapidly in situ at physiological temperatures makes them excellent materials for the use as injectable hydrogels.
Supramolecular assembly can also be used to prepare in situ gelling materials, for example, polyethylene glycols are well known to form polyrotaxanes with cyclodextrins which tend to undergo physical cross-linking and thus gelation via hydrogen-bond interactions. Attaching many short chain, polyethylene glycol monomethyl ether oligomers onto a polyphosphazene backbone recreates this effect (Fig. 25)48 and has the advantage to be able to adjust degradation rates thus making them viable materials for biomedical applications, for example in injectable drug delivery.
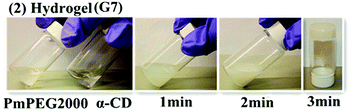 |
| Fig. 25 The formation of hydrogels upon mixing through the complexation of poly(organo)phosphazenes containing side oligo(ethylene glycol) methyl ethers (mPEGs) (called here PmPEG2000) with α-cyclodextrins (α-CD). Combination of the polymer with (α-CD) results in rapid hydrogel formation via reversible supramolecular assembly. Adapted with permission from Z. Tian et al., Macromolecules, 2013, 46, 2715. Copyright 2015 American Chemical Society.48 | |
7. Preparation of degradable poly(organo)phosphazenes
Much research and many proposed applications of polyphosphazenes, in particular in the biomedical field, are based on their degradability. As with many chemical properties of poly(organo)phosphazenes, the rates of degradation are determined by the nature of the organic substituents and can occupy a full spectrum from biostable, i.e. showing no signs of degradation over many years, as is the case for polyphosphazenes with fluoroalkoxy substituents, ranging to, for example, glyceryl derivatives that show complete degradation within several days.7 Thus when preparing new poly(organo)phosphazenes it is important to consider and/or where possible to predict what effect the substitution will have on the subsequent degradation rates. Degradation of poly(organo)phosphazenes occurs via substitution of the organic side groups with H2O, leading to the instable hydroxyphosphazene and phosphazane species, which subsequently leads to chain cleavage and degradation (shown for [PN(NHR)2]n in Fig. 26). The final degradation products are phosphates and ammonia, both of which can be readily detected by analytical methods,49 alongside the given organic substituent. Importantly, the degradation mixture of phosphates and ammonia is widely reported to be benign7,49 thus when care is taken with the choice of the organic component, it is possible to prepare materials with non-toxic degradation products. For some biomedical applications, a key advantage of polyphosphazene-based materials is indeed the reported near-neutral, pH-buffered degradation mixture of phosphates and ammonia,5 in contrast to the acidic products of the widely used poly(α-esters),50 that can be problematic for many uses.
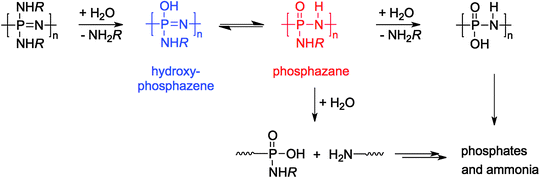 |
| Fig. 26 The proposed mechanism for the hydrolytic degradation of poly(organo)phosphazenes. | |
Rules governing the degradation rates of poly(organo)phosphazenes are sometimes complicated by chemical functionality in the organic side groups, but in general it can be said that hydrolysis is observed to be faster for most organic substituents with P–NH–R attachments than P–O–R, due to the strength of the P–O bond. Furthermore, hydrophobic substituents and bulky groups, which can sterically hinder H2O attack of the P atom, will tend to deter hydrolysis. Most frequently amino acid esters and dipeptide substituents have been used to prepare degradable poly(organo)phosphazenes,7 owing to their biocompatibility and wide availability making it relatively simple to prepare libraries of compounds with varying rates of hydrolysis. The rate of degradation of amino acid ester substituted polyphosphazenes tends to be deferred by larger, hydrophobic groups in the α-position of the amino acid ethyl ester, which can sterically hinder H2O attack.
Protonation of the backbone enhances the nucleophilic attack of H2O, thus lower pH values (<pH 7) increase degradation rates (Fig. 27c), an observation made for many different poly(organo)phosphazenes,43,49 whilst slightly basic conditions are generally observed to have little effect. In this context, poly[di(4-carboxyphenoxy)phosphazene] (PCPP) represents an interesting polymer and is well investigated due to its usefulness in drug delivery. Despite the phenoxy linkage (which would generally be expected to be quite hydrolysis resistant), PCPP can degrade in aqueous environments in medically relevant time-frames due to intramolecular acid catalysis from the carboxyl group.43
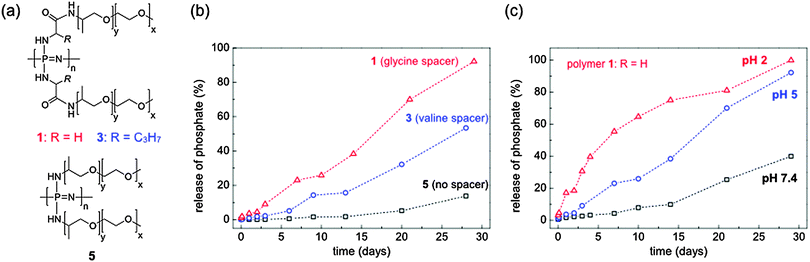 |
| Fig. 27 Structures (a) and degradation profiles (phosphate assay) of some exemplary water-soluble, degradable poly(organo)phosphazenes, exemplifying the accelerating effect of hydrolysis by subtle changes in chemical structure (b) and at differing pH values (c). Relative degradation rates are measured by determination of the phosphate backbone degradation product. Adapted from S. Wilfert et al., J. Polym. Sci., Part A: Polym. Chem., 2014, 52, 287. Copyright retained by authors.49 | |
Although macrosubstitution with amino acid esters and peptides enhances the rate of hydrolytic degradation, the mechanical properties of such polyphosphazenes are insufficient for some applications. Mixed post-polymerisation macrosubstitution of [NPCl2]n offers the opportunity here to balance out the properties required in terms of degradation rate and mechanical properties. Poly[(glycine ethyl glycinato)(phenyl phenoxy)phosphazene], a prevalent example here, is shown in Fig. 28, in which the phenyl phenoxy groups deter the rate of hydrolysis but increase the chain rigidity and thus mechanical modulus and Tg of the polymer. A balance is thus sought between the two functional groups to give the required mechanical properties whilst maintaining suitable degradation rates. This material was designed as a polyphosphazene scaffold for tissue regeneration, that is a biocompatible polymeric scaffold which can support and guide cell growth. Whilst particular mechanical properties are required to function as a scaffold, bearing significant weights to hold the growing tissue, the polymer must also be degradable (to non-toxic products) under physiological conditions in a suitable time-frame so that the material can be replaced by the growing cells.50 The synthetic flexibility of polyphosphazenes makes them an ideal platform for tuning the mechanical, chemical and biological properties of tissue engineering scaffolds and has been recently reviewed.5
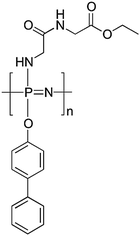 |
| Fig. 28 Chemical structure of poly[(glycine ethyl glycinato)(phenyl phenoxy)phosphazene] designed for use as a degradable scaffold for tissue engineering. | |
Whilst amino acid esters lead to hydrophobic polymers, water soluble and hydrolytically degradable polymers are also of importance for many biomedical applications due to concerns of the effects of dissolved biopersistent polymers in the body and in the environment. One simple way to achieve this, as demonstrated by Wilfert et al.,49 is the insertion of amino acid moieties between the backbone and a water-soluble organic substituent Jeffamine (an amine-capped polyalkylene oxide copolymer). These Jeffamine functionalised polyphosphazenes bearing either glycine or valine linkers showed enhanced degradation rates compared to the polyphosphazene without amino acid units (Fig. 27a), with the choice of amino acid ester determining the rate of degradation in aqueous media (Fig. 27b). Such polymers, which can degrade in a controlled manner in clinically relevant time-frames under physiological conditions, are of growing importance for biomedical materials science and in pharmaceutical applications (see ref. 50 for a comprehensive review of the positioning of polyphosphazenes in this rapidly expanding field).
8. Conclusions
Overall, poly(organo)phosphazenes represent an extremely versatile class of hybrid inorganic–organic polymers with very differing chemical and physical properties thus leading to their wide range of applications. Although the breadth of properties observed can be bewildering, it is a small number of unique combinations of properties which make polyphosphazenes extremely interesting materials. For instance, degradability for polymers which can be synthesised in a controlled manner is often not met, the combination of chirality and flexibility allowing dynamic helicity, or degradable polymers showing thermosensitive behaviour, to name a few examples encountered in this review. It would appear that it is these unique property combinations, not present in organic polymers, where polyphosphazene-based materials could prove most valuable. The methods and tools to design and synthesise polyphosphazenes with defined properties, as described herein, should enable the preparation of yet further innovative functional materials. Furthermore, recent developments in polyphosphazene synthesis have paved the way for the preparation of advanced architectures and supramolecular structures opening the window to yet more exciting, novel applications.
Acknowledgements
The authors would like to thank O. Brüggemann for his support of the polyphosphazene research programme at JKU Linz, as well as financial support of the Austrian Science Fund (FWF), project P 27410-N28.
References
-
H. R. Allcock, Chemistry and Applications of Polyphosphazenes, Wiley, Hoboken, USA, 2003 Search PubMed
.
- Z. Tian, A. Hess, C. R. Fellin, H. Nulwala and H. R. Allcock, Macromolecules, 2015, 48, 4301 CrossRef CAS
.
- A. K. Andrianov, D. P. DeCollibus, H. A. Gillis, H. H. Kha, A. Marin, M. R. Prausnitz, L. A. Babiuk, H. Townsend and G. Mutwiri, Proc. Natl. Acad. Sci. U. S. A., 2009, 106, 18936 CrossRef CAS PubMed
.
- S. Jankowsky, M. M. Hiller and H. D. Wiemhöfer, J. Power Sources, 2014, 253, 256 CrossRef CAS
.
- M. Deng, S. G. Kumbar, Y. Wan, U. S. Toti, H. R. Allcock and C. T. Laurencin, Soft Matter, 2010, 6, 3119 RSC
.
- I. Teasdale and O. Brueggemann, Polymers, 2013, 5, 161 CrossRef PubMed
.
- H. R. Allcock and N. L. Morozowich, Polym. Chem., 2012, 3, 578 RSC
.
- H. R. Allcock, Soft Matter, 2012, 8, 7521 RSC
.
- T. Mayer-Gall, D. Knittel, J. S. Gutmann and K. Opwis, ACS Appl. Mater. Interfaces, 2015, 7, 9349 Search PubMed
.
- A. B. Chaplin, J. A. Harrison and P. J. Dyson, Inorg. Chem., 2005, 44, 8407 CrossRef CAS PubMed
.
- A. K. Andrianov, J. Chen and M. P. LeGolvan, Macromolecules, 2004, 37, 414 CrossRef CAS
.
- H. Henke, S. Wilfert, A. Iturmendi, O. Brüggemann and I. Teasdale, J. Polym. Sci., Part A: Polym. Chem., 2013, 51, 4467 CrossRef CAS PubMed
.
- X. Liu, J. P. Breon, C. Chen and H. R. Allcock, Macromolecules, 2012, 45, 9100 CrossRef CAS
.
- G. A. Carriedo, F. L. G. Alonso, P. Gomez-Elipe, J. I. Fidalgo, J. L. G. Alvarez and A. Presa Soto, Chem. – Eur. J., 2003, 9, 3833 CrossRef CAS PubMed
.
- V. Blackstone, A. Presa Soto and I. Manners, Dalton Trans., 2008, 4363 RSC
.
- V. Blackstone, S. Pfirrmann, H. Helten, A. Staubitz, A. Presa Soto, G. R. Whittell and I. Manners, J. Am. Chem. Soc., 2012, 134, 15293 CrossRef CAS PubMed
.
- S. Wilfert, H. Henke, W. Schoefberger, O. Brüggemann and I. Teasdale, Macromol. Rapid Commun., 2014, 35, 1135 CrossRef CAS PubMed
.
- B. Wang, E. Rivard and I. Manners, Inorg. Chem., 2002, 41, 1690 CrossRef CAS PubMed
.
- B. Wang, Macromolecules, 2005, 38, 643 CrossRef CAS
.
- V. Blackstone, A. J. Lough, M. Murray and I. Manners, J. Am. Chem. Soc., 2009, 131, 3658 CrossRef CAS PubMed
.
- S. Suárez Suárez, D. Presa Soto, G. A. Carriedo, A. Presa Soto and A. Staubitz, Organometallics, 2012, 31, 2571 CrossRef
.
- A. Presa Soto and I. Manners, Macromolecules, 2009, 42, 40 CrossRef
.
- K. Matyjaszewski, M. K. Moore and M. L. White, Macromolecules, 1993, 26, 6741 CrossRef CAS
.
- J. H. G. Steinke, B. W. Greenland, S. Johns, M. P. Parker, R. C. J. Atkinson, I. A. Cade, P. Golding and S. J. Trussell, ACS Macro Lett., 2014, 3, 548 CrossRef CAS
.
-
P. Wisian-Neilson, Polyphosphazenes for Biomedical Applications, John Wiley & Sons, Inc., 2008, p. 155 Search PubMed
.
- S. Suárez-Suárez, G. A. Carriedo and A. Presa Soto, Chem. – Eur. J., 2015, 21, 14129 CrossRef PubMed
.
- A. L. Weikel, S. Y. Cho, N. L. Morozowich, L. S. Nair, C. T. Laurencin and H. R. Allcock, Polym. Chem., 2010, 1, 1459 RSC
.
- H. R. Allcock, C. R. de Denus, R. Prange and W. R. Laredo, Macromolecules, 2001, 34, 2757 CrossRef CAS
.
- X. Liu, Z. Tian, C. Chen and H. R. Allcock, Macromolecules, 2012, 45, 1417 CrossRef CAS
.
- H. Henke, S. Posch, O. Brüggemann and I. Teasdale, Macromol. Rapid Commun., 2016, 37, 769 CrossRef CAS PubMed
.
- C. Zheng, L. Y. Qiu and K. J. Zhu, Polymer, 2009, 50, 1173 CrossRef CAS
.
- C. Chen, H. Xu, Y.-C. Qian and X.-J. Huang, RSC Adv., 2015, 5, 15909 RSC
.
- A. Presa Soto, J. B. Gilroy, M. A. Winnik and I. Manners, Angew. Chem., Int. Ed., 2010, 49, 8220 CrossRef CAS PubMed
.
- S. Y. Cho and H. R. Allcock, Macromolecules, 2009, 42, 4484 CrossRef CAS
.
- S. Suárez-Suárez, G. A. Carriedo, M. P. Tarazona and A. Presa Soto, Chem. – Eur. J., 2013, 19, 5644 CrossRef PubMed
.
- K. Maeda, K. Kuroyanagi, S.-i. Sakurai, T. Yamanaka and E. Yashima, Macromolecules, 2011, 44, 2457 CrossRef CAS
.
- L. Zhu, Y. Xu, W. Yuan, J. Xi, X. Huang, X. Tang and S. Zheng, Adv. Mater., 2006, 18, 2997 CrossRef CAS
.
- J. Köhler, S. Kühl, H. Keul, M. Möller and A. Pich, J. Polym. Sci., Part A: Polym. Chem., 2014, 52, 527 CrossRef
.
- Z. Huang, S. Chen, X. Lu and Q. Lu, Chem. Commun., 2015, 51, 8373 RSC
.
- A.-M. Caminade, A. Hameau and J.-P. Majoral, Dalton Trans., 2016, 45, 1810 RSC
.
- V. Maraval, A.-M. Caminade, J.-P. Majoral and J.-C. Blais, Angew. Chem., Int. Ed., 2003, 42, 1822 CrossRef CAS PubMed
.
- A. K. Andrianov, A. Marin and B. E. Roberts, Biomacromolecules, 2005, 6, 1375 CrossRef CAS PubMed
.
- D. P. DeCollibus, A. Marin and A. K. Andrianov, Biomacromolecules, 2010, 11, 2033 CrossRef CAS PubMed
.
-
Polyphosphazenes for Biomedical Applications, ed. A. K. Andrianov, Wiley, Hoboken, USA, 2009 Search PubMed
.
- T. V. Burova, N. V. Grinberg, D. R. Tur, V. S. Papkov, A. S. Dubovik, V. Y. Grinberg and A. R. Khokhlov, Langmuir, 2011, 27, 11582 CrossRef CAS PubMed
.
- Y.-M. Kim, M.-R. Park and S.-C. Song, ACS Nano, 2012, 6, 5757 CrossRef CAS PubMed
.
- J. I. Kim, B. S. Lee, C. Chun, J.-K. Cho, S.-Y. Kim and S.-C. Song, Biomaterials, 2012, 33, 2251 CrossRef CAS PubMed
.
- Z. Tian, C. Chen and H. R. Allcock, Macromolecules, 2013, 46, 2715 CrossRef CAS
.
- S. Wilfert, A. Iturmendi, W. Schoefberger, K. Kryeziu, P. Heffeter, W. Berger, O. Brüggemann and I. Teasdale, J. Polym. Sci., Part A: Polym. Chem., 2014, 52, 287 CrossRef CAS PubMed
.
- B. D. Ulery, L. S. Nair and C. T. Laurencin, J. Polym. Sci., Part B: Polym. Phys., 2011, 49, 832 CrossRef CAS PubMed
.
Footnote |
† This author was formerly S. Wilfert. |
|
This journal is © The Royal Society of Chemistry 2016 |
Click here to see how this site uses Cookies. View our privacy policy here.