DOI:
10.1039/C5FO00464K
(Paper)
Food Funct., 2016,
7, 475-482
Hibiscus sabdariffa polyphenols prevent palmitate-induced renal epithelial mesenchymal transition by alleviating dipeptidyl peptidase-4-mediated insulin resistance†
Received
28th April 2015
, Accepted 14th October 2015
First published on 30th October 2015
Abstract
Diabetic nephropathy has a significant socioeconomic impact, but its mechanism is unclear and needs to be examined. Hibiscus sabdariffa polyphenols (HPE) inhibited high glucose-induced angiotensin II receptor-1 (AT-1), thus attenuating renal epithelial mesenchymal transition (EMT). Recently, we reported HPE inhibited dipeptidyl-peptidase-4 (DPP-4, the enzyme degrades type 1 glucagon-like peptide (GLP-1)), which mediated insulin resistance signals leading to EMT. Since free fatty acids can realistically bring about insulin resistance, using the palmitate-stimulated cell model in contrast with type 2 diabetic rats, in this study we examined if insulin resistance causes renal EMT, and the preventive effect of HPE. Our findings reveal that palmitate hindered 30% of glucose uptake. Treatment with 1 mg mL−1 of HPE and the DPP-4 inhibitor linagliptin completely recovered insulin sensitivity and palmitate-induced signal cascades. HPE inhibited DPP-4 activity without altering the levels of DPP-4 and the GLP-1 receptor (GLP-1R). HPE decreased palmitate-induced phosphorylation of Ser307 of insulin receptor substrate-1 (pIRS-1 (S307)), AT-1 and vimentin, while increasing phosphorylation of phosphatidylinositol 3-kinase (pPI3K). IRS-1 knockdown revealed its essential role in mediating downstream AT-1 and EMT. In type 2 diabetic rats, it suggests that HPE concomitantly decreased the protein levels of DPP-4, AT-1, vimentin, and fibronectin, but reversed the in vivo compensation of GLP-1R. In conclusion, HPE improves insulin sensitivity by attenuating DPP-4 and the downstream signals, thus decreasing AT-1-mediated tubular-interstitial EMT. HPE could be an adjuvant to prevent diabetic nephropathy.
1. Introduction
Among diabetic complications, nephropathy is perhaps the one with the greatest socioeconomic impact.1–3 Hyperglycemia and hypertension are generally considered crucial in diabetic nephropathy. However, despite careful treatment, renal damage continues to worsen in most patients. The mechanism inducing and leading to diabetic nephropathy remains active and unmodified by the present therapy.4 Once renal function begins to deteriorate, there is concern about the dosage of most antidiabetic drugs, because the metabolic and pharmacological controls become more difficult and complicated. Hence, new treatment paradigms are needed urgently.5
Type 2 diabetes, accompanied by the impact of obesity and characterized by insulin resistance, is indeed the most prevalent type of diabetes. Insulin action involves a series of signaling cascades initiated by a binding to its receptor, eliciting tyrosine phosphorylation of insulin receptor substrates (IRS), and leading to the activation of phosphatidylinositol 3-kinase (PI3K) and the downstream mediators.6 However, an increase in the phosphorylation of Ser307 of IRS-1 (pIRS-1 (S307)) hinders the response signals and glucose utilization.7
Recently, dipeptidyl peptidase-4 (DPP-4, a serine protease) inhibitors have emerged as useful tools for the treatment of type 2 diabetes mellitus. This mode of action is based on inhibiting the degradation of type 1 glucagon-like peptide (GLP-1), an incretin with a short half-life that stimulates glucose-dependent insulin secretion of β cells and suppresses glucagon release of α cells. In addition to its action on the pancreas, GLP-1 has direct effects on various organs including the heart, blood vessels and kidneys via the GLP-1 receptor (GLP-1R).8 Among the approved DPP-4 inhibitors, linagliptin lowers albuminuria in patients with type 2 diabetes and renal dysfunction, thus it could be beneficial for diabetic patients suffering from renal impairment.9
Fibrosis is a common feature found in chronic kidney disease.10 Although the expanded interstitial fibroblasts result in fibrosis, emerging evidence suggests that epithelial-mesenchymal transition (EMT) of renal tubular epithelial cells, with increasing vimentin, plays a critical role in renal fibrosis.11 It was reported that high glucose increased the synthesis of fibronectin in rat renal proximal tubular cells, while treatment with the angiotensin II receptor-1 (AT-1) antagonist reduced the signs of EMT.12
Our previous research suggested that the polyphenol extracts of Hibiscus sabdariffa L. calyx (HPE) have the potential to prevent diabetic renal damage. In type 2 diabetic rats exhibiting insulin resistance,13 HPE inhibited albuminuria and hyperfiltration, and prevented collagen accumulation in kidneys.14 HPE inhibited high glucose-induced AT-1, thus attenuating EMT in human tubular cells.15 Recently, we examined high glucose activated DPP-4, which mediates the downstream cascades of IRS-1 (S307) and vimentin. In vivo evidence showed that HPE reversed the expressions of IRS-1 (S307) and vimentin simultaneously in diabetic kidneys (shown in ESI S1†),16 leading to the occurrence of EMT. However, whether AT-1 is cross-talked with DPP-4 remains unclear. Besides, a few studies indicate that free fatty acids, but not high glucose, are much likely to induce insulin resistance and glucose uptake dysfunction.17–19
In the present study, we use palmitatestimulated HK-2 cells, in contrast with the kidneys of type 2 diabetic rats. We aim to investigate whether palmitate induces renal EMT via the putative mechanism of insulin sensitivity and signal cascades, and the preventive effect of HPE.
2. Methods and materials
2.1 Preparation of HPE
Hibiscus sabdariffa L. calyx was purchased from the Taitung Area Farmer's Association, Taiwan. HPE was prepared as in our previous report,20 and its chemical composition was analyzed by liquid chromatography–tandem mass spectrometry. Phenolic compounds including gallic acid, galloyl ester, protocatechuic acid, caffeic acid, caffeoyl quinic acid, chlorogenic acid, and quercetin derivative were found in HPE.20
2.2 Cell culture
HK-2 cells purchased from the Food Industry Research and Development Institute were cultured in keratinocyte serum free medium (Gibco BRL) with 5 ng mL−1 epidermal growth factor and 50 μg mL−1 bovine pituitary extract (both were also from Gibco BRL). The cells were grown in a humidified incubator at 37 °C under an atmosphere of 5% CO2 and 95% air. HK-2 cells were inoculated into a 6 cm dish at a density of 5 × 105 cells for 24 h. With PBS washing, the non-adherent cells were removed, and the attached cells were treated with various concentrations of palmitate with or without various concentrations (0.1–1 mg mL−1) of HPE for 48 h. The harvested cells were then prepared for western blot analysis.
2.3 Cytotoxicity assay
HK-2 cells were seeded at a density of 1 × 106 cells per mL in a 24-well plate for 24 h. With PBS washing, the non-adherent cells were removed, and the attached cells were incubated with palmitate at various concentrations (50–200 μM) for 48 h. Following this, the medium was changed and the cells were incubated with 3-(4,5-dimethylthiazol-2-yl)-2,5-diphenyltetrazolium bromide (MTT, 0.5 mg mL−1) for 4 h. The viable cells were directly proportional to the production of formazan. Following dissolution in isopropanol, the result was read at 563 nm with a spectrophotometer (Hitachi, U-3210). Treatments of HPE and linagliptin (a gift from Boehringer Ingelheim) have been tested by a cytotoxicity assay in our previous work, and proved to be nontoxic for HK-2 in the dose range.15,16 One mg mL−1 of HPE or 1 μM of linagliptin is the maximum cells can tolerate and exhibit a significant change. Linagliptin was dissolved with distilled water. The stock solutions of palmitate and HPE were prepared with 50% ethanol (100 mg ml−1 and 10 mM, respectively). Since the final concentration would be increased simultaneously with ethanol, the analysis of vehicle-only had been preliminarily tested.
2.4 Glucose uptake
Cells were seeded in 96-wells as 1.5 × 103 cells per well for 24 h. With PBS washing, the attached cells were tested for the effect of 1 mg mL−1 of HPE or 1 μM of linagliptin, with or without palmitate treatment for 48 h, in the presence of 1 μM of insulin. After being washed with PBS, the cells were starved overnight with 100 μL keratinocyte serum free medium (no BPE and EGF) per well, re-washed with PBS, and then starved for glucose by preincubating with 100 μl of Krebs-Ringer-Phosphate-HEPES (KRPH) buffer containing 2% BSA for 40 min. The cells were stimulated or not with 1 μM insulin for 20 min, then combined with 10 μl of 10 mM 2-deoxyglucose (2-DG) for 20 min. Glucose uptake was measured by the Glucose Uptake Colorimetric Assay Kit (Catalog # K676-100, BioVision) following the manufacturer's instructions, based on the generation of 2-DG6P and the amount of NADPH. Analysis of vehicle-only had also been tested.
2.5 Western blot analysis
Cells were harvested into a lysis buffer containing 50 mM Tris-HCl (pH 6.8), 10% glycerol, 2% SDS, and 5% mercaptoethanol and then lysed by sonication. Cell lysate was centrifuged at 9300g for 20 min at 4 °C, and the supernatant was collected as the protein sample. After quantifying, equal amounts of protein samples (50 μg) were subjected to 10% SDS–polyacrylamide gel electrophoresis and transferred to nitrocellulose membranes (Millipore, Bedford, MA). Membranes were blocked with 5% nonfat milk powder with 0.1% Tween-20 in TBS and then incubated with the primary antibody at 4 °C overnight against the following targets: IRS-1 (1
:
1000), pIRS-1 (S307) (1
:
500), PI3K (1
:
1000), pPI3K (p85, Tyr 458) (1
:
500), DPP4 (1
:
1000), GLP-1R (1
:
1000), AT-1 (1
:
1000), vimentin (1
:
1000), and fibronectin (1
:
1000). Antibodies of GLP-1R and DPP4 were from Abcam (Cambridge, U.K.). Antibodies of IRS-1, PI3K and vimentin were purchased from Santa Cruz, CA, and pIRS-1 (S307) and pPI3K were from Cell Signaling (Danvers, MA). Antibodies of AT-1 and fibronectin were from Epitomics and Novus Biological, respectively. Afterward, membranes were washed three times with 0.1% Tween-20 in TBS and incubated with the secondary antibody (1
:
5000) conjugated to horseradish peroxidase (GE Healthcare, Little Chalfont, Buckinghamshire, U.K.). Band detection was thereafter revealed by enhanced chemiluminescence using ECL Western blotting detection reagents and exposed in FUJFILM Las-3000 (Tokyo, Japan). Protein quantity was determined by densitometry using FUJFILM-Multi Gauge V2.2 software.
2.6 Assay for DPP-4 activity
Cells were seeded on a 96-well plate (1 × 106 cells per well) for 24 h, and then washed with PBS. After treatment with 50 μM of palmitate and different doses of HPE for 48 h, the cells of each well were lysed with 100 μL of NP-40 lysis buffer (containing 10 mM HEPES (pH 7.5), 142.5 mM KCl, 5 mM MgCl2, 1 mM EGTA, and 0.2% NP-40) and centrifuged at 9300g for 20 min at 4 °C. The supernatants were collected, and the protein concentrations were determined with the Bradford assay. DPP4 activity was measured by the DPP4/CD26 Assay Kit for Biological Samples (Enzo Life Sciences). Briefly, H-Gly-Pro-pNA, a chromogenic substrate of DPP-4, was hydrolyzed into the dipeptide Gly-Pro and the product 4-nitroaniline, whose rate of appearance was measured spectrophotometrically at 405 nm. The activity was normalized with protein concentration, and then in proportion to the control. Linagliptin, a gift from Boehringer Ingelheim, was used as a DPP4 inhibitor in the experiment.
2.7 siRNA transfection
HK-2 cells were seeded at 1 × 105 cells in each 6 cm Petri dish and incubated for 24 h, and then washed with PBS. Transfection of siRNA (IRS-1 of human origin) was performed by the Lipofectamine 2000 Reagent protocol. The final concentration of siRNA (SR302452A: rGrGrArUrArGrGrArCrArArArUrUrCrArGrUrArArArCrAGG) in the transfection medium (a keratinocyte serum-free medium without bovine pituitary extract and EGF) was 45 nM; incubation was carried out for 6 h. The transfection medium was subsequently replaced with a normal cultured medium and incubated for another 12 h. After that, the cells were treated with or without 50 μM of palmitate for 24 h. Cell proteins were then extracted and prepared for western blotting.
2.8 Animal experiment
The animal experimental project was approved by the Animal Model Experimental Ethics Committee of Chung-Shan Medical University based on the European Community guidelines (IACUC approval No: 1007). A type 2 diabetic model was carried out according to our previous publication.13,20 Briefly, male Sprague-Dawley rats (weight 250 ± 20 g) obtained from LuxBiotech Co., Taiwan, were divided into several groups (n = 8 for each group), including the control (normal diet), FAT + STZ (high fat diet and streptozotocin injection), FAT + STZ + low HPE (with 100 mg kg−1 HPE tube-fed), and FAT + STZ + high HPE (with 200 mg kg−1 HPE tube-fed) groups. The animals’ diets were prepared as previously suggested.20 Seven weeks later, when the average body weight was measured to be 425 ± 15 g, the FAT groups were injected with a dose of STZ 35 mg per kg bw. About two weeks later, when the hyperglycemic status was confirmed, rats were tube-fed with or without different doses of HPE. The metabolic features, histological findings, and biomolecule expressions of this model have been shown in our previous reports.14,20 Safety evaluations revealed that HPE did no harm to the liver, kidney, or cardiovascular tissue. After the rats were sacrificed, the kidney chops were added to a radioimmunoprecipitation assay (RIPA) buffer and protein inhibitors and homogenized at 4 °C. The tissue homogenates were centrifuged at 9300g for 20 min at 4 °C, and the resulting supernatants (whole-tissue extracts) were used for western blot analysis of GLP-1R, DPP-4, AT-1, vimentin and fibronectin.
2.9 Statistical analysis
The statistical software SPSS v.12.0 was used to analyze the data. One-way ANOVA was performed (p < 0.05), while Bonferroni's multiple comparison was used for post-test.
3. Results
3.1 Palmitate is tested for nontoxic dose range
The test of the cytotoxicity assay revealed that palmitate is nontoxic to HK-2 under 75 μM (Fig. 1). Accordingly, 50 μM of palmitate was the dose chosen for the subsequent experiment. Analysis of the vehicle-only was shown to be non-toxic (shown in ESI S2(A)†).
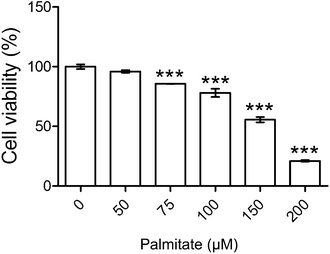 |
| Fig. 1 Cytotoxicity test of palmitate. HK-2 cells were incubated for 48 h with or without different concentrations of palmitate. Cell viability was calculated as a percentage compared with the control group. Data are presented as means ± SD (n = 3) and analyzed with ANOVA. ***p < 0.001, compared with the control. | |
3.2 HPE and linagliptin reversed glucose uptake in palmitate-stimulated insulin-resistant cells
In the presence of 1 μM of insulin, palmitate decreased 30% of the glucose uptake in HK-2 cells. HPE (at 1 mg ml−1) and DPP-4 inhibitor linagliptin (1 μM) significantly recovered the glucose uptake hindered by palmitate (Fig. 2). As shown in ESI S2(B),† treatment with both 1 mg ml−1 of HPE and 50 μM of palmitate was non-toxic to the cells. Besides, glucose uptake was not affected by the vehicle-only (shown in S3†). This result implies that DPP-4 alone, in the absence of GLP-1, could interfere with insulin sensitivity and its function. Therefore, in future experiments, we will focus on the role of DPP-4 in the signal cascades, and investigate the mechanism of HPE.
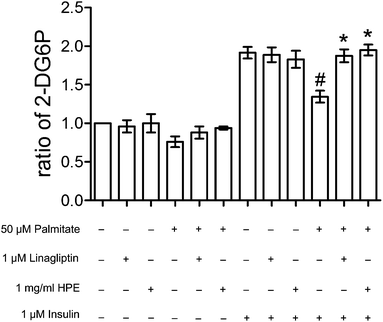 |
| Fig. 2 Effect of HPE and DPP-4 inhibitors on glucose uptake. HK-2 cells were incubated for 48 h with or without 50 μM of palmitate (50 μL palmitate stock solution added with 9950 μL medium; vehicle concentration 0.25%), 1 mg mL−1 of HPE (100 μL HPE stock solution added with 9900 μL medium; vehicle concentration: 0. 5%), 1 μM of linagliptin, and 1 μM of insulin. Cells were analyzed with an uptake of 2-DG, and the longitudinal axis indicates ratios compared with the control. #p < 0.05, compared with the group treated with insulin alone. *p < 0.05, compared with the group treated with insulin and palmitate. | |
3.3 HPE inhibits DPP-4 activity induced by palmitate
Fig. 3A shows that the protein expression of GLP-1R and DPP-4 was neither altered by palmitate nor HPE. However, by measuring DPP-4 activity, the alteration was significant. Palmitate increased the activity of DPP-4 almost 2-fold. Although a dose of 0.1 mg mL−1 could not suppress the activation, 0.5 and 1 mg mL−1 of HPE dose-dependently inhibit DPP-4 activity. Especially at 1 mg mL−1, the activation was completely alleviated (Fig. 3B). This result implies that at least partly by regulating the activity of DPP-4, HPE might rescue the palmitate-induced insulin resistance and signal cascades.
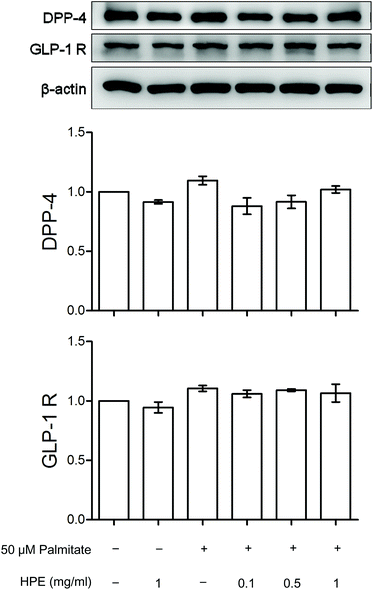 |
| Fig. 3 Effect of HPE on DPP-4 and GLP-1R. HK-2 cells were incubated for 48 h with or without 50 μM of palmitate and different concentrations of HPE. (A) Cell proteins were extracted and analyzed with western blot. Protein levels of DPP-4 and GLP-1R were represented as folds compared with the control. (B) DPP-4 activity was analyzed, and calculated as fold compared with the control. Data are presented as means ± SD (n = 3) and analyzed with ANOVA. #p < 0.05, compared with the control. *p < 0.05, compared with the palmitate-treated group. | |
3.4 DPP-4 mediates the regulation of vimentin, AT-1, and phosphorylation of IRS-1 (S307)
Compared with the control, palmitate increased vimentin more than 2-fold and AT-1 nearly 3-fold. Treatment with 1 mg mL−1 of HPE inhibited the expression of AT-1 and vimentin even below the control. In addition, the phosphorylation of IRS-1 (S307) was increased 2.5-fold by palmitate, and HPE inhibited the phosphorylation effectively (Fig. 4). In contrast, PI3K, a normal response signal transmitting insulin sensitivity, was decreased by about 50% by palmitate while recovered by either linagliptin or HPE. These results suggest that there might be a cascade downstream of DPP-4 involving vimentin, AT-1, and IRS-1 (S307).
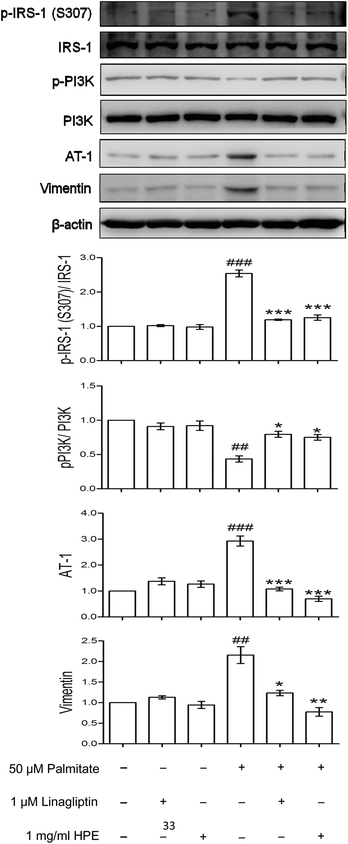 |
| Fig. 4 Effect of HPE on IRS-1 (S307), p-PI3K, AT-1 and vimentin. HK-2 cells were incubated for 48 h with or without 50 μM of palmitate and different concentrations of HPE. Cell proteins were extracted and analyzed with western blot. The ratios of IRS-1 (S307)/IRS-1, p-PI3K/PI3K, and the levels of AT-1 and vimentin were represented as folds compared with the control. Data are presented as means ± SD (n = 3) and analyzed with ANOVA. #p < 0.05, ##p < 0.01 compared with the control. *p < 0.05, **p < 0.01, ***p < 0.001, compared with the palmitate-treated group. | |
3.5 IRS-1 plays a critical role in mediating the downstream expression of AT-1 and vimentin
For illustrating whether AT-1 and vimentin elevation could be downstream of IRS-1, si-RNA of IRS-1 was transfected into the palmitate-incubated tubular cells. Fig. 5A shows that the knockdown blocked most of the IRS-1 expression and reduced about 70% of the IRS-1 protein. Palmitate-induced AT-1 and vimentin were significantly attenuated by the si-RNA (Fig. 5B), indicating that IRS-1 and its phosphorylation could be an essential mediator of downstream AT-1 and a marker of EMT.
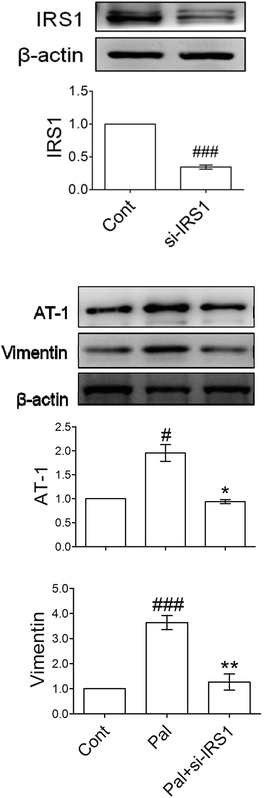 |
| Fig. 5 Signal cascades of IRS-1, AT-1 and vimentin. HK-2 cells were transfected with siRNA, and then incubated under different conditions. Cell proteins were extracted and analyzed with western blot. Protein levels were represented as folds compared with the control. Data are presented as means ± SD (n = 3) and analyzed with ANOVA. #p < 0.05, ### p < 0.001, compared with the control. *p < 0.05, **p < 0.01, compared with the palmitate-treated group. | |
3.6 HPE alleviates both increases of DPP-4/GLP-1R and AT-1/EMT in diabetic kidneys
The expressions of GLP-1R, DPP-4, AT-1, fibronectin and vimentin in the kidneys of type 2 diabetic rats were detected by western blot. The in vivo data showed that in diabetic kidneys, protein levels of DPP-4 and GLP-1R increased 3-fold and 4-fold, respectively. Treatment with high HPE (200 mg kg−1) significantly reduced the protein levels of DPP-4 and GLP-1R (Fig. 6). A similar result was also found in the expression of AT-1. Compared with the data of Fig. 3A, there exist discrepancies between the in vitro and in vivo expressions of both GLP-1R and DPP-4.
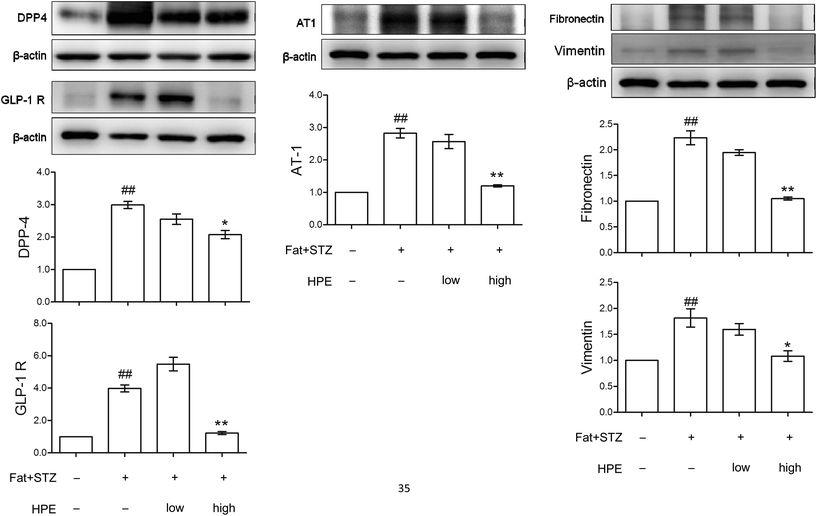 |
| Fig. 6 Effect of HPE on diabetic kidneys. The kidney proteins DPP-4, GLP-1R, AT-1, fibronectin and vimentin in normal and type 2 diabetic rats with or without HPE treatment (100 mg kg−1 or 200 mg kg−1) were examined by western blot. Protein levels were represented as folds compared with the control. Data are presented as means ± SD (n = 3) and analyzed with ANOVA. ##p < 0.01 compared with the control. *p < 0.05, **p < 0.01, compared with the diabetic group (Diet formula for the experimental animals is shown in ESI S7†). | |
The expression of vimentin and fibronectin was elevated, implicating the occurrence of EMT in diabetic kidneys, while treatment with HPE significantly reduced the markers of EMT (Fig. 6). These results are in accordance with the change of renal fibrosis in our previous work (shown in ESI S4†).14 Our recent report demonstrated that pIRS-1 (S307) is also concomitantly expressed with vimentin in type 2 diabetic kidneys (shown in ESI S1†).16 As a result, the evidence showed that expressions of GLP-1R and DPP-4 correlate with insulin resistance and the cascades of renal EMT.
4. Discussion
In the present study, we demonstrate that DPP-4 plays a critical role in insulin resistance and diabetic nephropathy. Inhibiting the activity of DPP-4 recovers insulin sensitivity and glucose uptake hindered by palmitate. DPP-4 activation evokes the insulin resistance signals by increasing phosphorylation of IRS-1 (S307) and decreasing phosphorylation of PI3K, therefore mediating the expression of AT-1 and vimentin. In vitro and in vivo evidence suggests that HPE has a good ability to attenuate diabetic renal EMT, and has the added effect of regulating the cascades.
Noticeably, the protein expressions of GLP-1R and DPP-4 were not altered in vitro, but significantly changed in vivo. The levels of GLP-1R and DPP-4 substantially increased in the kidneys of type 2 diabetic rats, but decreased under 200 mg kg−1 of HPE treatment. During the long-term feeding, DPP-4 might be induced gradually, suggesting that much more DPP-4 would be activated, thereby mediating the signal cascades. The increase of GLP-1R, however, could be viewed as a feedback for compensating for a deficit of GLP-1 in this tissue. Similar results were found in the previous report. The expression of GLP-1R was increased in visceral adipose depots from morbidly obese patients with a high degree of insulin resistance. A prospective study carried out with patients who underwent biliopancreatic diversion surgery showed that subjects with a high level of GLP-1R expression were those whose insulin sensitivity improved after surgery.21
The DPP-4 inhibitor improved renal function parameters including albuminuria and creatinine clearance, or histological changes of tubular-interstitial volume and glomerular basement membrane thickness, while the effects appeared to be independent of blood glucose lowering.9,19 On top of an angiotensin receptor blocker, the DPP-4 inhibitor significantly reduced urinary albumin excretion and oxidative stress in diabetic eNOS knockout mice independent of blood glucose or systolic blood pressure.22 In the recent years, some studies have investigated the putative role of DPP-4 on insulin resistance. Treatment with the DPP-4 inhibitor significantly improved hyperglycemia and insulin resistance in SHR/NDmcr-cp rats, as evidenced by an oral glucose tolerance test and homeostasis model assessment for insulin resistance, respectively.23 DPP-4 has a higher release from visceral adipose tissue that is particularly pronounced in obese and insulin-resistant patients.24 In adults with a metabolic syndrome, a DPP-4 decrease was associated with insulin sensitivity following exercise training and weight loss.25 The DPP-4 inhibitor vildagliptin improves insulin sensitivity and beta-cell function in subjects with impaired fasting glucose.26 In a clinical trial, linagliptin lowered the albuminuria in type 2 diabetic patients, independent of the level of HbA1C.9 Our investigation revealed that HPE, as well as linagliptin, regulates insulin sensitivity and signal cascades via DPP-4, hence attenuating the renal damage. Furthermore, the usual dose of linagliptin (MW = 472.54) for type 2 DM is 2.5 mg daily per os which corresponds to 5.3 μmoles. Taking the serum volume to be approximately 4 L per capita for humans, the transient blood concentration could yield 1.325 μM. In the present study, 1.0 μM of linagliptin was used as a DPP-4 inhibitor for the experiment, which is indeed correct and feasible.
We have demonstrated that AT-1 mediates high glucose-induced renal EMT via up-regulating TGF-β1, thus promoting the generation of fibroblasts. Treatment with HPE inhibited the transduction of AT-1/TGF-β1 and recovered the increase of vimentin and fibronectin, thus avoiding the accompanying fibrosis.15 Treatment with the AT-1 antagonist attenuated high glucose-induced TGF-β1 elevation and ERK1/2 phosphorylation, resulting in reduced EMT.12 It has been reported that the hypoxia-inducible factor prolyl-hydroxylase-2 mediates TGF-β1-induced EMT in renal tubular cells.27 TGF-β induced α-SMA, collagen I, and fibronectin, and reduced E-cadherin of tubular cells via regulating response gene to complement 32.28 Therefore, in the process of palmitate-induced renal EMT, TGF-β1 and downstream signals could be responsible for mediating the action of AT-1. Furthermore, although AT-1 is effective as a signal mediating the cascades, its role in regulating blood pressure still cannot be excluded.
The maximum dose of HPE used in our in vitro experiments is 1 mg ml−1. This dose range is not toxic to the cells, and induces significant effects in HK-2 cells. However, this range of concentrations is likely too high to be physiologically relevant. The synergistic effects cannot be excluded since at least 18 phenolic compounds were found in HPE.20 We have tested some and estimated their ability to inhibit the palmitate-induced signals leading to renal EMT (shown in ESI S5 and S6†). Caffeic acid, protocatechuic acid, chlorogenic acid, and gallic acid all have the potential to inhibit the activation of DPP-4. A low dose of caffeic acid especially inhibits vimentin. Gallic acid and chlorogenic acid are fairly effective, whereas protocatechuic acid has no role in preventing palmitate-induced EMT. Therefore, caffeic acid, chlorogenic acid, and gallic acid could be responsible for the utility of HPE in preventing diabetic renal fibrosis. In the literature, the tested compounds were reported to be antidiabetic. Caffeic acid promotes glycolysis and glycogen synthesis, but inhibits gluconeogenesis in insulin-resistant hepatocytes.29 Caffeic acid prevents diabetic nephropathy by decreasing HbA1c, blood urine nitrogen, urine output, renal advanced glycation end product (AGE), and inflammatory cytokines, while improving clearance of creatinine.30 Chlorogenic acid lowers the fasting plasma glucose and lipid peroxides in diabetic rats.31 Gallic acid stimulates insulin secretagogue by regenerating β-islets of the pancreas.32 Galloyl ester decreases oxidative stress and AGEs, thus improving liver and kidney functions.33 Treatment with protocatechuic acid also reduces plasma HbA1c and renal receptors of AGEs.34
In conclusion, using the palmitate-stimulated cells and type 2 diabetic rats, we demonstrated the superior effect of HPE. By regulating the activity of DPP-4, HPE improves insulin sensitivity and alleviates insulin resistance signals, thus decreasing the AT-1-mediated tubular-interstitial EMT. HPE could be an adjuvant to prevent diabetic renal damage.
Acknowledgements
Funding for this research was provided by the National Science Council, Taiwan, under MOST 103-2320-B-241-002.-.
References
- Anon: CDC – National Diabetes Fact Sheet – Publications – Diabetes DDT, 2005.
- H. King, R. E. Aubert and W. H. Herman, Diabetes Care, 1988, 21, 1414–1431 CrossRef.
- H. W. Rodbard, L. Blonde, S. S. Braithwaite, E. M. Brett, R. H. Cobin, Y. Handelsman, R. Hellman, P. S. Jellinger, L. G. Jovanovic, P. Levy, J. I. Mechanick and F. Zangeneh, Endocr. Pract., 2007, 13, S1–S68 CrossRef PubMed.
- P. Balakumar, M. K. Arora, J. Reddy and M. B. Anand-Srivastava, J. Cardiovasc. Pharmacol., 2009, 54, 129–138 CrossRef CAS PubMed.
- L. Chaykovska, K. von Websky, J. Rahnenführer, M. Alter, S. Heiden, H. Fuchs, F. Runge, T. Klein and B. Hocher, PLoS One, 2011, 6, e27861 CAS.
- K. Choi and Y. B. Kim, Korean J. Intern. Med., 2010, 25, 119–129 CrossRef CAS PubMed.
- C. L. Lin and J. K. Lin, Mol. Nutr. Food Res., 2008, 52, 930–939 CAS.
- B. Hocher, C. Reichetzeder and M. L. Alter, Kidney Blood Pressure Res., 2012, 36, 65–84 CrossRef CAS PubMed.
- P. H. Groop, M. E. Cooper, V. Perkovic, A. Emser, H. J. Woerle and M. von Eynatten, Diabetes Care, 2013, 36, 3460–3468 CrossRef CAS PubMed.
- G. Remuzzi, A. Benigni and A. Remuzzi, J. Clin. Invest., 2006, 116, 288–296 CAS.
- J. Li, X. Qu and J. F. Bertram, Am. J. Pathol., 2009, 175, 1380–1388 CrossRef CAS PubMed.
- L. Zhou, H. Xue, P. Yuan, J. Ni, C. Yu, Y. Huang and L. M. Lu, Clin. Exp. Pharmacol. Physiol., 2010, 37, e152–e157 CrossRef CAS PubMed.
- C. H. Peng, Y. B. Ker, C. F. Weng, C. C. Peng, C. N. Huang, L. Y. Lin and R. Y. Peng, J. Agric. Food Chem., 2009, 57, 8812–8819 CrossRef CAS PubMed.
- Y. S. Yang, C. N. Huang, C. J. Wang, Y. J. Lee, M. L. Chen and C. H. Peng, J. Funct. Foods, 2013, 5, 810–819 CrossRef CAS.
- Y. S. Yang, C. J. Wang, C. N. Huang, M. L. Chen, M. J. Chen and C. H. Peng, J. Agric. Food Chem., 2013, 61, 7545–7551 CrossRef CAS PubMed.
- C. H. Peng, Y. S. Yang, K. C. Chan, C. J. Wang, M. L. Chen and C. N. Huang, J. Agric. Food Chem., 2014, 62, 9736–9743 CrossRef CAS PubMed.
- R. Ragheb, G. M. Shanab, A. M. Medhat, D. M. Seoudi, K. Adeli and I. G. Fantus, Biochem. Biophys. Res. Commun., 2009, 13, 211–216 CrossRef PubMed.
- W. Chai, J. Liu, L. A. Jahn, D. E. Fowler, E. J. Barrett and Z. Liu, Diabetes Care, 2011, 34, 1634–1638 CrossRef CAS PubMed.
- Y. Li, S. Zhao, W. Zhang, P. Zhao, B. He, N. Wu and P. Han, Diabetes Res. Clin. Pract., 2011, 93, 205–214 CrossRef CAS PubMed.
- C. H. Peng, C. C. Chyau, K. C. Chan, T. H. Chan, C. J. Wang and C. N. Huang, J. Agric. Food Chem., 2011, 59, 9901–9909 CrossRef CAS PubMed.
- J. Vendrell, R. El Bekay, B. Peral, F. E. Garcia, A. Megia, G. M. Macias, J. F. Real, G. Y. Jimenez, X. Escote, G. Pachon, R. Simo, D. M. Selva, M. M. Malagon and F. J. Tinahones, Endocrinology, 2011, 152, 4072–4079 CrossRef CAS PubMed.
- M. L. Alter, I. M. Ott, K. von Websky, O. Tsuprykov, Y. Sharkovska, K. Krause-Relle, J. Raila, A. Henze, T. Klein and B. Hocher, Kidney Blood Pressure Res., 2012, 36, 119–130 CrossRef CAS PubMed.
- H. Nakagami, Z. Pang, T. Shimosato, T. Moritani, H. Kurinami, H. Koriyama, A. Tenma, M. Shimamura and R. Morishita, Hypertens. Res., 2014, 37, 629–635 CrossRef CAS PubMed.
- H. Sell, M. Bluher, N. Kloting, R. Schlich, M. Willems, F. Ruppe, W. T. Knoefel, A. Dietrich, B. A. Fielding, P. Arner, K. N. Frayn and J. Eckel, Diabetes Care, 2013, 36, 4083–4090 CrossRef CAS PubMed.
- S. K. Malin, H. Huang, A. Mulya, S. R. Kashyap and J. P. Kirwan, Peptides, 2013, 47, 142–147 CrossRef CAS PubMed.
- K. M. Utzschneider, J. Tong, B. Montgomery, J. Udayasankar, F. Gerchman, S. M. Marcovina, C. E. Watson, M. A. Ligueros-Saylan, J. E. Foley, J. J. Holst, C. F. Deacon and S. E. Kahn, Diabetes Care, 2008, 31, 108–113 CrossRef CAS PubMed.
- W. Q. Han, Q. Zhu, J. Hu, P. L. Li, F. Zhang and N. Li, Biochim. Biophys. Acta, 2013, 1833, 1454–1462 CrossRef CAS PubMed.
- W. Y. Huang, Z. G. Li, H. Rus, X. Wang, P. A. Jose and S. Y. Chen, J. Biol. Chem., 2009, 284, 9426–9432 CrossRef CAS PubMed.
- D. W. Huang and S. C. Shen, J. Funct. Foods, 2012, 4, 358–366 CrossRef CAS.
- C. Y. Chao, M. C. Mong, K. C. Chan and M. C. Yin, Mol. Nutr. Food Res., 2010, 54, 388–395 CAS.
- K. Karthikesan, L. Pari and V. P. Menon, J. Funct. Foods, 2010, 2, 134–142 CrossRef CAS.
- R. C. Latha and P. Daisy, Chem. – Biol. Interact., 2011, 189, 112–118 CrossRef CAS PubMed.
- C. H. Park, J. S. Noh, N. Yamabe, K. S. Kang, T. Tanaka and T. Yokozawa, Eur. J. Pharmacol., 2010, 640, 233–242 CrossRef CAS PubMed.
- C. Y. Lin, S. J. Tsai, C. S. Huang and M. C. Yin, J. Agric. Food Chem., 2011, 59, 5117–5124 CrossRef CAS PubMed.
Footnote |
† Electronic supplementary information (ESI) available: S1, S2, S3, S4, S5, S6 and S7. See DOI: 10.1039/c5fo00464k |
|
This journal is © The Royal Society of Chemistry 2016 |
Click here to see how this site uses Cookies. View our privacy policy here.