DOI:
10.1039/C5FO00705D
(Paper)
Food Funct., 2016,
7, 445-454
Effects of post-suckling n-3 polyunsaturated fatty acids: prevention of dyslipidemia and liver steatosis induced in rats by a sucrose-rich diet during pre- and post-natal life
Received
11th June 2015
, Accepted 14th September 2015
First published on 17th September 2015
Abstract
The interaction between fetal programming and the post-natal environment suggests that the post-natal diet could amplify or attenuate programmed outcomes. We investigated whether dietary n-3 long-chain polyunsaturated fatty acids (n-3 PUFAs) at weaning resulted in an amelioration of dyslipidemia, adiposity and liver steatosis that was induced by a sucrose-rich diet (SRD; where the fat source is corn oil) from the onset of pregnancy up to adulthood. During pregnancy and lactation, dams were fed an SRD or the standard powdered rodent commercial diet (RD). At weaning and until 150 days of life, male offspring from SRD-dams were divided into two groups and fed an SRD or SRD-with-fish oil [where 6% of the corn oil was partially replaced by fish oil (FO) 5% and corn oil (CO) 1%], forming SRD–SRD or SRD–FO groups. Male offspring from RD-dams continued with RD up to the end of the experimental period, forming an RD–RD group. The presence of FO in the weaning diet showed the following: prevention of hypertriglyceridemia and liver steatosis, together with increased lipogenic enzyme activity caused by a maternal SRD; the complete normalization of CPT I activity and PPARα protein mass levels; a slight but not statistically significant accretion of visceral adiposity; and limited body fat content and reduced plasma free fatty acid levels. All of these results were observed even in the presence of a high-sucrose diet challenge after weaning. SRD-dams' breast milk showed a more saturated fatty acid composition. These results suggest the capacity of n-3 PUFAs to overcome some adverse outcomes induced by a maternal and post-weaning sucrose-rich diet.
Introduction
Different lines of evidence indicate that macronutrient status during the perinatal period could influence adult susceptibility to developing a number of abnormalities that are included within the metabolic syndrome.1 This phenomenon, called early nutritional programming of adult health, is strongly supported by animal experimental evidence. A variety of metabolic dysfunctions were produced when hypocaloric (variations in protein amount and/or quality) or hypercaloric (high-fat, ‘junk food’ or high-protein) diets were provided to rodents during pregnancy and lactation2–6 or when pups were exposed to overnutrition at birth.6
In the past two decades, the intake of simple sugars (sucrose/fructose/high-fructose corn syrup) has increased markedly all over the world. Although much research and discussion is ensuing about the effect of these nutrients on adult metabolism, there are few data concerning the impact and mechanisms of increased maternal intake of simple sugars on the mother, placenta, fetus and the disease risk of offspring.7 Experimental animal models using enriched fructose/sucrose diets during pregnancy have been reported to bring about increased liver lipogenesis and insulin resistance in mothers, in addition to adverse effects on placental and fetal development.8–12 Concerning the later-life impact via fetal metabolic programming, previous data have demonstrated an increased hepatic lipid content in adult offspring born from BHE (a carbohydrate-sensitive animal model for the study of non-insulin-dependent diabetes mellitus without obesity) pregnant rats fed a sucrose-rich diet (SRD).13 This fact was evidenced even though the progeny had been fed a control diet from weaning. Samuelsson et al.14 demonstrated that a sugar-rich diet in utero and during the suckling period led to hyperinsulinemia, increased adiposity and impaired glucose tolerance in mouse female offspring weaned on a control diet at 3 months of age. More recently – in normal Wistar rats – D'Alessandro et al.15 reported that maternal high-sucrose feeding during pregnancy and suckling is a crucial factor in determining the long-term programming of impaired glucose homeostasis and altered lipid metabolism regardless of the fact that the offspring were fed a control diet up to 100 days of age (young adult). Moreover, extending the post-natal period up to 150 days, sucrose-programmed derangements were more pronounced and when a sucrose-rich diet was also present after weaning, the possible predictive adaptive protection on adiposity, dyslipidemia and altered glucose homeostasis was not induced for sucrose-fed dams.16
Although the alterations resulting from nutrient-fetal programming have been considered as irreversible changes,17 some studies have addressed the capacity of the post-natal environment, including hyper- or hypo-caloric dietary modifications,18 to either exacerbate or attenuate programmed outcomes. Wyrwoll et al.19,20 demonstrated that post-natal, high n-3 fatty acid supplementation (5% fat diet with 38% n-3 fatty acids) form birth to six months of age rescued glucocorticoid-programmed hypertension, hyperlipidemia and the overall inflammatory state of adult offspring. More recently, Zulkafli et al.21 showed the same capacity of n-3 fatty acids in the glucocorticoid-programmed model even in the presence of a high-fat diet challenge. Moreover, Hou et al.22 – in Sprague-Dawley rats – showed that a 6% fish oil (FO) diet during the post-suckling period prevented the programmed excess of adipose accumulation and insulin resistance in the model of early post-natal overfed rats.
In this regard, a significant body of evidence indicates that the dietary intake of marine PUFA [fish oil rich in 20:5n-3 (EPA) and 22:6n-3 (DHA)], involved in many biological processes, appears to play an important role against the adverse effects of dyslipidemia, obesity, and insulin resistance, among others.23,24 Taken together, these observations led us to hypothesize that dietary n-3 PUFAs given after suckling may also provide a viable option for preventing and/or reducing adverse outcomes induced by a sucrose-rich diet during the fetal and post-natal periods. To test this hypothesis, we investigated the partial substitution of corn oil (rich in linoleic acid, 18:2n-6) by fish oil (rich in n-3 PUFAs) as a fat source in the sucrose-rich diet and its effects on the anthropometrical parameters, adiposity and hepatic lipid metabolism of adult offspring from SRD-dams.
Methods
Animal models and diets
Female Wistar rats (200–230 g) purchased from the National Institute of Pharmacology (Buenos Aires, Argentina) were housed in a colony room with a 12 h light/dark cycle and constant temperature (22 °C) and humidity. The experimental protocol was approved by the Human and Animal Research Committee of the School of Biochemistry, University of Litoral, Santa Fe, Argentina, and adequate procedures were taken to minimize the pain or discomfort of the rats. The oestrous cycle – by vaginal smears – was monitored by collecting material with the aid of a dropper containing 0.9% saline solution. Females in the proestrus phase that exhibited signals of sexual receptivity were mated with males of the same strain. The day on which spermatozoids appeared in vaginal smears was considered day 1 of gestation. Rats were fed a standard powdered rodent commercial diet (Cargill SA, Argentina) containing (% w/w) carbohydrate (corn, sorghum, wheat, oats, barley): 43; protein: 21.5; fat: 5.1; fiber: 6; minerals: 8; vitamins: 1.9; digestible energy 12.73 kJ g−1 as stated by the manufacturer before and during mating. Pregnant rats were transferred to individual cages. Through gestation and lactation, one group of rats (n = 12) was fed a home-made sucrose-rich diet (SRD) containing (% w/w) carbohydrate (sucrose): 62.5; protein (casein free of vitamins) 18; fat: (corn oil) 6; fiber (cellulose) 8.5; minerals (salt mix): 3.5; vitamins (vitamin mix) 1.0. Other components of the SRD (choline chloride and methionine) and energy are described in Table 1 and are in agreement with what was recommended by the final report of the American Institute of Nutrition).25 The preparation and handling of the SRD has been reported elsewhere.26 Another group of rats (n = 6) – considered as a reference group (RD) – was fed the standard powdered rodent commercial diet as described above.
Table 1 Composition of home-made experimental dietsa
Ingredients |
SRDb |
SRD-with-FOc |
% by weight |
% of energy |
% by weight |
% of energy |
Diets are based on the AIN-93M formula.
SRD: Sucrose-rich diet (source of fat = corn oil).
SRD–FO: SRD (source of fat = fish oil and corn oil).
Salt mix is based on salt mix AIN-93M (in g per kg of diet): calcium carbonate, 357.0; potassium phosphate (monobasic) 250.0; sodium chloride, 74.0; potassium sulfate, 46.6; potassium citrate, tri-potassium (monohydrate) 28.0; magnesium oxide, 24.0; ferric citrate, 6.06; zinc carbonate, 1.65; manganese carbonate, 0.63; cupric carbonate, 0.30; potassium iodate, 0.01; sodium selenate, 0.01025; ammonium paramolybdate, 0.00795; chromium potassium sulfate, 0.275.
Vitamin mix is based on vitamin mix AIN-93M (in g per kg of diet): niacin 3.00; calcium pantothenate, 1.60; pyridoxine HCl, 0.70; thiamin HCl, 0.60; riboflavin, 0.60; folic acid, 0.20; d-biotin, 0,02; vitamin B-12 (0.1% in mannitol) 2.5 Units; vitamin E (500 IU g−1), 15.00; vitamin A (500 000 IU g−1) 0.80; vitamin D3 (400 000 IU), 0.25; vitamin K, 0.075.
In the SRD without the addition of FO offered to offspring at weaning, cholesterol and vitamins D and A were added as follows (mg per kg of diet): cholesterol 30; vitamin A 42.5; vitamin D 4.25, in order to balance with the SRD-with-FO.
|
Casein free of vitamin |
18.0 |
19.49 |
18 |
19.49 |
Salt mixd |
3.5 |
|
3.5 |
|
Vitamin mixe,f |
1.0 |
|
1.0 |
|
Choline chloride |
0.2 |
|
0.2 |
|
Methionine |
0.3 |
|
0.3 |
|
Cellulose |
8.5 |
|
8.5 |
|
Sucrose |
62.5 |
66.49 |
62.5 |
66.49 |
Corn oil (CO) |
6.0 |
14.36 |
1.0 |
2.39 |
Fish oil (FO) |
|
|
5.0 |
11.97 |
Energy (kJ g−1) |
|
15.73 |
|
15.73 |
At birth, pups were weighed and the litter size was reduced to eight pups, with an equal number of male and female pups whenever possible. The pups were kept with their own mother until weaning (21 days of life). At this time, the male offspring of SRD-fed dams were weighed and assigned to either an SRD diet or an SRD diet where the source of fat [corn oil (CO) at 6% w/w] was partially replaced by fish oil [(FO) at 5 g per 100 g of cod liver oil, ICN Biomedical, Costa Mesa, CA, plus 1 g per 100 g of CO] (SRD-with-FO) (see Table 1). Offspring born to SRD dams fed either an SRD or SRD-with-FO diet after weaning formed the SRD–SRD (n = 24) and SRD–FO (n = 24) groups, respectively. The male offspring (n = 24) from RD-fed dams continued with RD after weaning, forming the reference group (RD–RD). All offspring were fed their respective diet until 150 days of age (5 months).
Corn oil in the SRD-with-FO was included to maintain the essential fatty acid proportion within the normal range. The amount of fish oil used in this study was in agreement with that previously described in adult animals27–29 or after weaning22 (7 and 6% by weight, respectively), and also slightly lower (5% by weight in the present study). The base mixture was stored at 4 °C until preparation of the diet. Fish oil was kept under an atmosphere of nitrogen during storage at −20 °C and was added every day to a fresh base mixture containing the other nutrients. The SRD without the addition of FO offered to the SRD offspring at weaning was balanced with cholesterol and vitamins D and A present in FO (Table 1).
The present study was conducted in male offspring to avoid the effects of different sexual hormones on the lipid metabolism. Throughout the experimental period, dams and offspring had free access to food and water and were kept under controlled room conditions, as described above. Food intake and body weights of the offspring were monitored once a week, starting at post-weaning until the end of the experimental period (150 days of life), although fresh food was provided every day as described above, to avoid the potential oxidation of n-3PUFAs.
At the end of the experimental period, food was removed at 0700 hours and experiments were performed between 0700 and 0900 hours. At least six rats from each dietary group were used in each experiment. Rats were anesthetized with sodium pentobarbital (60 mg kg−1 i.p.). Blood samples were obtained from the jugular vein, collected in tubes containing ethylenediaminetetraacetic (EDTA) sodium salt as anticoagulant (1 mg ml−1 of blood) and centrifuged at 2700g for 10 min at room temperature. The plasma obtained was either immediately assayed or stored at −20 °C until use. Immediately, the liver, heart and gastrocnemius muscle were removed, weighed, and frozen at −80 °C until use. The epididymal and retroperitoneal adipose tissues were also removed and weighed. Adiposity was calculated by the sum of epididymal and retroperitoneal fat weights divided by body weight × 100, and expressed as the adiposity percentage.30
Milk collection and lipid analysis of diets and milk
At weaning, milk samples were collected from dams. After separation from pups for 60 min, dams were anesthetized i.p. with sodium pentobarbital (60 mg kg−1 i.p.) and injected with 4 IU kg−1 of oxytocin (Sigma Chemical Comp. St Louis, MO) to stimulate milk flows. Milking was initiated 10 min after the oxytocin injection and collected by hand extraction. The milk was dispensed into micro vials under nitrogen atmosphere and frozen at −20 °C until a fatty acid compositional analysis was performed, as described below. Lipids were extracted from the different diets and milks according to the procedure reported by Folch et al.31 Samples were derivatized with boron trifluoride (BF3) at 64 °C for 3 h, and the fatty acid composition of total lipids was determined by gas liquid chromatography of their methyl esters, as previously described.32,33Table 2 shows the fatty acid composition of each diet and Table 3 shows the fatty acid composition of dam milk.
Table 2 Fatty acid composition of the experimental dietsa
Fatty acids |
RD |
SRD |
SRD-with-FO |
Data are expressed as % of total extracted fatty acids. RD: reference diet; SRD: sucrose-rich diet (source of fat = corn oil); SRD-with-FO: SRD (source of fat = fish oil and corn oil); ∑SFA: sum of saturated fatty acids, ∑MUFA: sum of monounsaturated fatty acids; ∑n-6: sum of polyunsaturated n-6 fatty acids; ∑n-3: sum of polyunsaturated n-3 fatty acids.
|
14:0 |
1.10 |
Traces |
4.10 |
16:0 |
19.80 |
10.46 |
11.40 |
18:0 |
19.79 |
2.62 |
3.13 |
20:0 |
0.10 |
0.40 |
0.90 |
∑SFA
|
40.88
|
13.48
|
19.53
|
|
16:1 n-7 |
2.50 |
|
6.70 |
18:1 n-9 |
18:1 n-9 |
44.90 |
32.30 |
27.49 |
18:2 n-6 |
20:1 n-9 |
0.10 |
1.60 |
12.29 |
∑MUFA
|
47.50
|
33.90
|
48.48
|
|
18:2 n-6 |
9.43 |
51.82 |
11.30 |
20:3 n-6 |
0.10 |
0.07 |
|
20:4 n-6 |
0.98 |
0.25 |
|
22:4 n-6 |
0.39 |
|
|
22:5 n-6 |
0.51 |
0.08 |
|
∑n-6
|
11.41
|
52.22
|
11.30
|
|
18:3 n-3 |
0.20 |
0.40 |
|
20:4 n-3 |
|
|
3.10 |
20:5 n-3 |
|
|
9.00 |
22:5 n-3 |
|
|
0.50 |
22:6 n-3 |
|
|
8.10 |
∑n-3
|
0.20
|
0.40
|
20.70
|
Table 3 Total breast milk fatty acid composition of dams fed a reference diet (RD) or sucrose-rich diet (SRD) during pregnancy and lactationa
Fatty acid |
Breast milk groups |
RD |
SRD |
Data are expressed as % of total extracted fatty acids. Values are expressed as mean ± SEM, n = 3 (assayed in duplicate); *p < 0.05 compared with RD. ∑SFA: sum of saturated fatty acids, ∑MUFA: sum of monounsaturated fatty acids; ∑n-6: sum of polyunsaturated n-6 fatty acids; ∑n-3: sum of polyunsaturated n-3 fatty acids.
|
C12:0 |
Traces |
4.5 ± 0.1* |
C14:0 |
1.5 ± 0.03 |
5.6 ± 0.11* |
C15:0 |
Traces |
Traces |
C16:0 |
14.0 ± 0.2 |
35.0 ± 1.5* |
C17:0 |
0.3 ± 0.03 |
0.2 ± 0.03 |
C18:0 |
9.0 ± 0.1 |
3.1 ± 0.1* |
C20:0 |
1.7 ± 0.1 |
0.3 ± 0.04* |
∑SFA
|
26.5
|
48.7
|
|
16:1n-7 |
1.5 ± 0.1 |
1.8 ± 0.2 |
18:1n-9 |
34.5 ± 2.6 |
23.9 ± 0.8* |
20:1n-9 |
0.4 ± 0.1 |
0.3 ± 0.04 |
∑MUFA
|
36.4
|
26.0
|
|
18:2n-6 |
30.7 ± 2.4 |
22.0 ± 0.7* |
18:3n-6 |
0.4 ± 0.1 |
0.4 ± 0.04 |
20:2n-6 |
1.0 ± 0.1 |
0.5 ± 0.1* |
20:4n-6 |
0.6 ± 0.1 |
0.3 ± 0.11* |
22:4n-6 |
2.1 ± 0.1 |
1.3 ± 0.1* |
22:5n-6 |
0.3 ± 0.02 |
0.1 ± 0.01* |
∑n-6
|
35.1
|
24.6
|
|
18:3n-3 |
0.4 ± 0.1 |
0.4 ± 0.04 |
20:5n-3 |
0.4 ± 0.02 |
0.2 ± 0.02* |
22:4n-3 |
Traces |
Traces |
22:5n-3 |
0.2 ± 0.01 |
Traces |
22:6n-3 |
1.0 ± 0.1 |
0.5 ± 0.04* |
∑n-3
|
2
|
1.1
|
Anthropometrical determinations
The abdominal circumference, thoracic circumference and body length (nose–anus length) were determined in all rats at 150 days of age.34 The measurements were made in anaesthetized rats (as previously described). The body weight and body length were used to determine the body mass index (BMI) = body weight (g)/length2 (cm2).
Analytical methods
A commercially available analytical kit was employed to determine the plasma triglyceride (TG) concentration (Wiener Lab., Rosario, Santa Fe, Argentina). Plasma free fatty acids (FFAs) were determined using an acyl-CoA oxidase-based colorimetric kit (Wako NEFA-C, Wako Chemicals, Neuss, Germany). The liver, heart and skeletal muscle TG content were determined by the method described by Laurell.35 Briefly, liver lipids were extracted using isopropyl ether
:
ethanol (95
:
5 v
:
v); phospholipids were removed by adsorption on silicic acid and glyceride esters were saponified to glycerol. Glycerol was determined by oxidation with periodate, using a colorimetric measurement of the product from the reaction of formaldehyde and chromotropic acid in sulfuric acid. A UV-Visible Hitachi U1500 instrument (Hitachi High Technology, Japan) was used for all spectrophotometric assays.
Carcass composition
Visceral organs were removed in pre-shaved anesthetized rats from each dietary group. The carcass was weighed and frozen at −20 °C until compositional evaluation.36 Total nitrogen – previously converted into ammonium sulfate – was measured in appropriate aliquots from the carcass homogenate by the Kjeldahl procedure (AOAC 1999 #954.01). Carcass protein contents were estimated by multiplying their nitrogen contents by 6.25. Dried samples of carcasses were used to measure the fat content. After extraction with petroleum ether (AOAC 1999 #920.39), the total lipid contents were gravimetrically estimated by solvent evaporation up to a constant weight. The water contents were determined by drying aliquots of the carcasses to a constant weight in a 60 °C oven (AOAC 1999 #934.01).
Liver enzymatic activity assays
Acetyl-CoA carboxylase (ACC) and glucose-6-phosphate dehydrogenase (G-6-PDH).
Frozen liver tissue samples were homogenized (motor-driven Teflon glass homogenizer, Thomas Scientific Swedesboro, NJ, USA) in an ice-cold buffer [9 mmol L−1 KH2PO4, 85 mmol L−1 K2HPO4, 1 mmol L−1 dithiothreitol (DTT), and 70 mmol L−1 KHCO3, (pH 7)] and centrifuged at 100
000g for 1 h at 4 °C (Beckman Coulter, LE80, Palo Alto, CA, USA). The cytosolic fractions were used for the assay of enzyme activities. ACC activity was measured using an NADH-linked assay, with the slight modifications described by Zimmermann et al.37 G-6-PDH was investigated following the increase of nicotinamide adenine dinucleotide (NADH) absorption at 340 nm, according to Cohen et al.38
Fatty acid synthase (FAS).
Frozen liver tissue samples were homogenized as described above in an ice-cold buffer [0.25 mmol L−1 sucrose, 1 mmol L−1 DTT and 1 mmol L−1 EDTA (pH 7.4)] and centrifuged at 100
000g for 1 h at 4 °C. FAS was immediately assayed in the cytosolic fraction (duplicate measurements) by determining malonyl-CoA-dependent oxidation of nicotinamide adenine dinucleotide phosphate (NADPH) at 37 °C.39
Carnitine-palmitoyltransferase I (CPT I).
CPT I activity was analyzed as described by Karlic et al.40 Briefly, pieces of frozen liver were homogenized in an ice-cold buffer (0.25 mmol L−1 sucrose, 1 mmol L−1 EDTA, 0.1% ethanol, 1 mg L−1 antipain, 2 mg L−1 aprotinin, 1 mg L−1 leupeptin, 0.7 mg L−1 pepstatin, 0.2 mol L−1 phenyl methyl-sulfonil fluoride) using an Ultra-Turrax Type X120 disperser (Ingenieurburo CATM Zipperer GmbH, Germany) at maximum speed for 30 s and then centrifuged at 300g at 4 °C for 10 min. The precleared supernatant was centrifuged at 12
000g at 4 °C for 5 min. CPT I activity was spectrophotometrically assayed in these supernatants following the release of CoA-SH from palmitoyl-CoA using the thiol reagent 5,5-dithiobis(2-nitrobenzoic acid) (DTNB).
Western blot analysis of liver protein mass levels of PPARα
Liver homogenates from frozen tissue were prepared for PPARα protein mass level analysis and the western blots were run under reduced-denaturated protein (Laemmli buffer) as described by Hein et al.27 The protein concentration of the extracts was determined using the Bradford assay. Protein samples were resolved on SDS-PAGE slab gels according to Laemmli. Before electrophoresis, all samples were boiled for 2 min in the presence of 1% SDS with 200 μM DTT. For immunoblotting, proteins in the SDS-PAGE gel were transferred to polyvinyldifluoride (PVDF) membranes. The membranes were probed with a specific antibody (rabbit anti-PPARα antibody, from Santa Cruz Biotechnology, Inc., Santa Cruz, CA, USA). The blots were then incubated with horseradish peroxidase-linked secondary antibody followed by chemiluminescence detection according to the manufacturer's instructions (Super Signal West Pico chemiluminescence detection, Pierce Biotechnology, Rockford, IL,USA). β-Actin was used as a loading control. The intensity of the bands was quantified by NIH imaging software. The relationship between the amount of sample subjected to immunoblotting and the signal intensity observed was linear under the conditions described above.
Statistical analysis
Sample sizes were calculated on the basis of measurements previously made with rats fed either an RD or an SRD12,15,16,25,32 considering 80% power. Results were expressed as the mean ± SEM. Statistical analyses were performed with SPSS version 17.0 (SPSS, Chicago, IL, USA). The data were subjected to a one-way ANOVA, followed by Tukey's post-hoc test or, when appropriate, the statistical significance of differences was determined using the Student's t-test. Means with p values <0.05 were considered statistically different.41
Results
Effect of SRD on dam weight, pup number and offspring weight gain during lactation
Confirming previous results,12 both female weight gain during pregnancy (Δ weight for 1–21 days of gestation) and the number of pups at birth were slightly but significantly decreased in the SRD group compared with the RD group. Values are expressed as the mean ± SEM: Δ weight (g) were 93.28 ± 5.16 in SRD (n = 12) vs. 110.33 ± 5.68 in RD (n = 6) (p < 0.05); number of pups per dam 9.00 ± 0.13 in SRD vs. 10.17 ± 0.33 in RD (p < 0.05). Fig. 1 shows male offspring body weight gain during lactation from the two groups of dams. SRD-pups had slightly but significantly reduced body weights (g) compared to RD offspring at birth (results were mean ± SEM): 5.80 ± 0.08 SRD (n = 48) vs. 6.15 ± 0.11 RD (n = 24), (p < 0.05). Similar results were obtained when only 8 pups were considered in each group (data not shown). Moreover, SRD neonates displayed a rapid catch-up in growth and were heavier than RD neonates at the time of weaning (day 21).
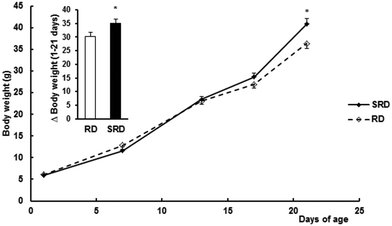 |
| Fig. 1 Mean weight and body weight gain from birth to weaning in offspring from dams fed a reference rodent chow (RD) or a sucrose-rich diet (SRD). Values are expressed as the mean ± SEM (n = 24); *p < 0.05 SRD vs. RD. | |
Effect of perinatal exposure to SRD on breast milk fatty acid composition
The breast milk fatty acid composition of female rats fed an RD or SRD diet during pregnancy and lactation is given in Table 3. Sucrose feeding during pregnancy and lactation significantly displaces the fatty acid profile to saturation including short chain fatty acids as C12:0 and C14:0. A remarkable accumulation of C16:0, but no C18:0, was also depicted in the SRD-breast milk. On the other hand, linoleic acid (18:2) – a crucial precursor of higher homologues of the n-6 series – is considerably decreased, as well as 20:2, 20:4, 22:4 and 22:5n-6 fatty acids. Fifty-percent decreases of eicosapentaenoic (EPA) and docosahexaenoic acid (DHA) were also detected compared to RD-breast milk.
Effects of diet on adult offspring weight, food and energy intake, and body composition
Food intake, energy intake, body weight, body mass, body length and BMI were similar between male offspring fed RD, SRD or SRD-with-FO throughout their post-weaning period (weaning up to 150 days of age) (Table 4). An increased adiposity was observed in offspring from SRD-fed dams weaned on the same diet up to 150 days of age. When FO was present in the SRD after weaning, the adiposity was slightly lower, although significant differences with the SRD-SRD and RD–RD groups were not seen. Moreover, the higher fat content of the carcass observed in the SRD–SRD group significantly decreased when FO was incorporated into the diet after weaning at the expense of increasing the carcass water content. The amounts of protein and ash were similar in all groups. No significant differences were observed in liver and heart weights. Results were (mean ± SEM; n = 6): liver total weight (g) 14.18 ± 0.32 RD–RD, 14.95 ± 0.45 SRD–SRD and 15.01 ± 0.50 SRD–FO; heart total weight (g) 1.22 ± 0.04 RD–RD, 1.21 ± 0.04 SRD–SRD and 1.16 ± 0.03 SRD–FO. Relative liver and heart weights (g per 100 g body weight) were also similar between groups (data not shown).
Table 4 Food and energy intake, anthropometric measurements, body mass index (BMI), adiposity and carcass composition of adult offspring at the end of the experimental period (150 days)a
Group |
RD–RD |
SRD–SRD |
SRD–FO |
RD–RD: Offspring born from RD-dams weaned on RD; SRD–SRD: offspring born from SRD-dams and weaned on the SRD; SRD–FO: offspring born from SRD-dams weaned on an SRD-with-FO. Values are expressed as the mean ± SEM. Six animals were included in each experimental group. Values in each line that do not share the same superscript letter were significantly different (p < 0.05) when one variable at a time was compared using Tukey's test.
|
Food intake (g per rat per day) |
19.80 ± 0.60 |
17.50 ± 0.70 |
17.70 ± 0.90 |
Energy intake (kJ per rat per day) |
254.43 ± 6.68 |
263.68 ± 11.30 |
275.70 ± 13.97 |
Final body weight (g) |
415.10 ± 12.05 |
400.25 ± 9.97 |
398.60 ± 11.48 |
Body length (cm) |
23.50 ± 0.20 |
23.26 ± 0.19 |
23.37 ± 0.19 |
Abdominal circumference (cm) |
19.30 ± 0.29 |
19.20 ± 0.30 |
19.30 ± 0.16 |
Thoracic circumference (cm) |
16.80 ± 0.50 |
18.18 ± 0.45 |
17.05 ± 0.30 |
BMI (g cm−2) |
0.74 ± 0.02 |
0.72 ± 0.02 |
0.73 ± 0.01 |
Adiposity (%) |
2.38 ± 0.12b |
3.39 ± 0.11a |
2.82 ± 0.07a,b |
Carcass weight (g) |
324.51 ± 8.10 |
306.40 ± 10.50 |
301.10 ± 10.9 |
Protein (% wet weight) |
21.80 ± 0.12 |
21.46 ± 0.24 |
21.12 ± 0.16 |
Fat (% wet weight) |
7.74 ± 0.26c |
10.86 ± 0.20a |
9.69 ± 0.23b |
Water (% wet weight) |
64.23 ± 0.09a |
62.55 ± 0.07c |
63.10 ± 0.29b |
Ash (% wet weight) |
4.77 ± 0.11 |
4.68 ± 0.30 |
4.87 ± 0.12 |
Effects of diets on adult offspring plasma lipid content and tissue triglyceride levels
Plasma lipid content and tissue triglyceride levels are depicted in Table 5. Plasma triglycerides and free fatty acid (FFA) levels as well as liver, heart and skeletal muscle triglyceride content were significantly higher in offspring from SRD-fed dams weaned on the same diet (SRD–SRD group), compared to age-matched RD–RD offspring. Concerning the SRD–FO group, Table 5 shows that plasma, liver, heart and muscle triglyceride concentrations were indistinguishable from those of RD–RD offspring. Although the plasma FFA content significantly decreased in the offspring group from SRD-dams where fish oil replaced corn oil from weaning, it remained above the values shown for the age-matched control RD-fed dams. Interestingly, Fig. 2 shows a positive correlation between plasma FFA levels and adiposity.
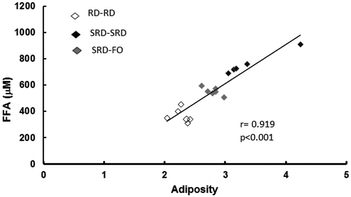 |
| Fig. 2 Correlation between plasma free fatty acids and adiposity of offspring at the end of the experimental period (150 days). RD–RD: offspring fed RD at weaning from dams fed RD; SRD–SRD: offspring fed SRD at weaning from dams fed SRD; SRD–FO: offspring fed SRD-with-FO at weaning from dams fed SRD; (n = 6 per dietary group), p < 0.05 SRD–SRD vs. RD–RD and SRD–FO offspring, p < 0.05 SRD–FO vs. RD–RD. | |
Table 5 Plasma free fatty acid (FFA) and triglyceride (TG) levels and liver, heart and muscle TG content of adult offspring at the end of the experimental period (150 days)a
Groups |
Metabolites |
Plasma FFA (μM) |
Plasma TG (mM) |
Liver TG |
Heart TG |
Gastrocnemius TG |
(μmol per g wet tissue) |
RD–RD: offspring born from RD-dams weaned on RD; SRD–SRD: offspring born from SRD-dams and weaned on SRD; SRD–FO: offspring born from SRD-dams weaned on SRD-with-FO. Values are expressed as the mean ± SEM. Six animals were included in each experimental group. Values in each column that do not share the same superscript letter are significantly different (p < 0.05) when one variable at a time was compared using Tukey's test.
|
RD–RD |
365 ± 27c |
0.71 ± 0.05b |
8.26 ± 0.44b |
3.55 ± 0.25b |
2.00 ± 0.36b |
SRD–SRD |
726 ± 52a |
1.18 ± 0.10a |
18.69 ± 1.43a |
5.95 ± 0.28a |
5.08 ± 0.62a |
SRD–FO |
573 ± 28b |
0.72 ± 0.05b |
10.13 ± 0.78b |
3.93 ± 0.25b |
3.22 ± 0.30b |
Effects of diets on adult offspring hepatic enzymes activities involved in lipid metabolism and PPARα protein mass level
Fig. 3A shows that when offspring are exposed to SRD during the entire experimental period, the activities of lipogenic enzymes (FAS, ACC; G-6-PDH) were significantly increased. On the other hand, the mitochondrial CPT I, which controls fatty acid entry into mitochondria and therefore fatty acid oxidation, was decreased (Fig. 3B). The effect of the n-3 PUFA-rich fish oil after weaning on offspring from SRD-fed dams reverses these effects. This was mostly accomplished by a decrease and normalization of the FAS, ACC and G-6-PDH and by enhancing fatty acid oxidation through an increase of CPT I activity. The results of the measurements of liver PPARα protein content – in offspring from SRD-fed dams weaned on the different diet compared with the RD–RD group – demonstrated that during the entire life period (pregnancy, lactation and post-weaning), the SRD had a depressing effect. The partial replacement of the source of fat (corn oil by fish oil) at weaning normalized the PPARα protein mass (Fig. 3C).
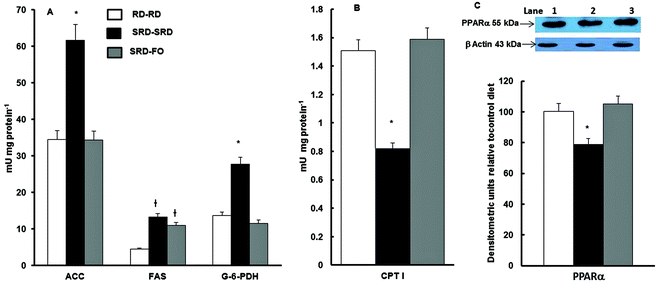 |
| Fig. 3 Activities of liver enzymes related to lipid metabolism and protein mass levels of PPARα of offspring at the end of the experimental period (150 days). RD–RD: offspring fed RD at weaning from dams fed RD; SRD–SRD: offspring fed SRD at weaning from dams fed SRD; SRD–FO: offspring fed SRD-with-FO at weaning from dams fed SRD. (A) Values for acetyl-CoA carboxylase (ACC), fatty acid synthase (FAS) and glucose-6-P-dehydrogenase (G-6-PDH) and (B) carnitine-palmitoyltransferase I (CPT I) activities are expressed as the mean ± (n = 6); *p < 0.05 vs. RD–RD and SRD–FO; p < 0.05 vs. RD–RD. (C) Upper part: immunoblots of PPARα from RD–RD (lane 1), SRD–SRD (lane 2) and SRD–FO (lane 3) with molecular weight marker showed on the right. Lower part: densitometric immunoblot analyses of PPARα protein mass in liver tissue. Values are expressed as the mean ± SEM (n = 6) and expressed as percentage to RD–RD. *p < 0.05 vs. RD–RD and SRD–FO. | |
Discussion
Diets enriched in carbohydrates during early development have been proved to influence the susceptibility of developing the metabolic alteration of glucose and lipid metabolism in adulthood.11,15,16 The present study shows the effect of post-weaning dietary n-3 polyunsaturated fatty acids on lipid disorders induced by a sucrose-rich diet from the onset of pregnancy until 150 days of life in offspring. The key findings of this work point to the fact that the incorporation of fish oil in the diet after weaning induces: (1) the prevention of hypertriglyceridemia, liver steatosis and the increased lipogenic enzyme activities caused by a maternal sucrose-rich diet; (2) a complete normalization of CPT I activity and PPARα protein mass levels in offspring born from SRD-fed dams; (3) a slight and not statistically significant accretion of visceral adiposity; and (4) limited body fat content and reduced plasma free fatty acid levels. All these results were observed even in the presence of a high-sucrose diet challenge after weaning. (5) Finally, expanding our previous research15,16 the present study provides information concerning the composition of breast milk fatty acids.
To date, few studies have investigated the effect of a sucrose-rich diet during pregnancy upon the fetus or newborn offspring.42 Experimental research in similar models has produced conflicting results mainly due to differences in the strain of rat used and/or the quantity of carbohydrate present in the diet.11,12,43 A previous report12 with the same strain of rats employed in our study and a home-made control diet (starch as a source of carbohydrate) reported that sucrose feeding during pregnancy induced a decreased conceptus weight, a small number of fetuses per litter, and a decreased mass of both fetuses and placental tissues. In the present study, the SRD-dams displayed the same behavior, although we analyzed newborn offspring instead of fetuses and the results were compared to those obtained with standard rodent chow-fed dams.
The rapid catch-up growth of offspring from SRD-dams could be linked to the lipid quality of the maternal diet during lactation. The type of dietary fat is known to determine the fatty acid (FA) composition of milk lipids both directly and indirectly by influencing the de novo synthesis of FA within the mammary gland or other tissues and also by mobilization of lipids from adipose tissue stores.44 Therefore, the maternal dietary intake through pregnancy and lactation has an impact on the profile of breast milk fatty acid.45 In this regard, as described in Table 2, although the sucrose-rich diet provides lower saturated fatty acids (13.48%) compared with the reference diet (40.88%), our results demonstrate that breast milk fatty acids are significantly affected by the SRD leading to an important increase in saturated (mainly C12:0, C14:0 and C16:0) fatty acids. The low unsaturation index – increased saturated/unsaturated fatty acid proportion – suggests a more lipogenic breast milk from sucrose-fed dams. Novak and Innis46 showed that diets enriched in carbohydrates promote the biosynthesis of medium-chain fatty acids in the gland. Milk composition was also altered in BALB/c mice fed a sucrose-rich diet associated with increased offspring body weight and body fat before the ingestion of solid food (12 days of age).47 Recently, Priego et al.48 observed a positive correlation between the relative content in milk of some saturated fatty acids, particularly medium chain fatty acids (C10:0, C12:0 and C14:0) and body weight gain of offspring. On the other hand, the relative levels of oleic acid in milk correlated negatively with body weight of offspring. Of course we cannot discard other possible mechanism(s) to explain our experimental observations, considering that we compared breast-milk from rats fed a home-made sucrose-rich diet with that from rats fed a commercial rodent chow.
Regarding the amount of C18:0 and C18:1 from SRD-breast milk fatty acids compared to RD-breast milk, a state of deficiency in the fatty acid elongation from their respective metabolic precursors C16:0 and C16:1 could be assumed. Moreover, the differences observed between the ratios of C16:1/C16:0 and C18:1/C18:0 suggest a different efficiency of metabolic conversion by the Δ9 desaturase isoenzymes.49 Novak and Innis46 showed that the long chain n-3 fatty acids are relevant for hepatic metabolic regulation in the milk-fed rat neonates. Although we neither directly nor indirectly measured the activities of key enzymes involved in the lipid metabolism of neonates, SRD-breast milk exhibited a significantly lower proportion of long-chain n-3 PUFAs compared to RD-breast milk. Thus, the previously described46 effect could also be accomplished in our experimental model.
Further experimental evidence links the capacity of fish oil to limit fat deposition, preferentially located in visceral depots. Most of the studies were carried out in adult experimental models of pre-existing metabolic and morphological changes of visceral fat pad tissue and/or obesity (ref. 24, 28 and references therein). To the best of our knowledge, the present is the first study to analyze the post-weaning effects of dietary n-3 fatty acids on adiposity induced by a sucrose-rich diet from the onset of pregnancy until adulthood. The presence of fish oil as a source of fat in the SRD after weaning not only slightly mitigated adiposity but also improved the fat carcass content and plasma free fatty acids, with no effect on total body weight or total energy intake. In this line, Hou et al.22 showed that in post-suckling Sprague-Dawley rats fed on 6% fish oil, the diet during the post-suckling period prevents programmed an excess of adipose accumulation in early post-natal overfed rats by reducing the litter sizes after post-natal day 3. The mechanism behind the changes induced by fish oil could involve the binding of n-3 fatty acids (especially EPA) to DNA and the subsequent activation of the PPAR-γ2 expression. This transcription factor results in a coordinated increase of a large number of genes that are involved in the lipid metabolism and remodeling of the adipose tissue in adult animals.50
In agreement with previous results,16 the presence of sucrose at any time of life (SRD–SRD group) induces dyslipidemia and liver steatosis in the adult offspring, associated with a deregulation of the hepatic enzymes involved in lipid metabolism. The fructose moiety of the sucrose diet has proved to be related to the up-regulation of the gene expression of hepatic fatty acid synthesis. Mukai et al.10 reported that a 10% fructose intake during rat pregnancy led to upregulation of maternal and fetal hepatic sterol regulatory element-binding protein (SREBP1c). Indeed, fetuses from fructose-fed mothers (10% drinking water) displayed higher hepatic triglyceride content associated with a higher expression of genes related to lipogenesis and a lower expression of fatty acid catabolism genes.11 On the other hand it is well known that PPARα is strongly expressed in liver tissue. This fact led to a modulation of many genes involved in mitochondrial, peroxisomal and microsomal fatty acid oxidation.51 The decreased PPARα protein mass content depicted in SRD–SRD offspring suggests this is at least one of the ways to explain the observed lipid steatosis. In this regard, Hein et al.27 and Nagai et al.52 found that a sucrose/fructose-enriched diet also produced a decline of PPARα protein (and activity), thus reducing fatty acid oxidation. The accumulation of triglycerides in non-adipose tissues such as heart and skeletal muscle observed in the SRD–SRD groups could be the consequence of the increased availability of plasma metabolic substrates and the altered glucose homeostasis that we previously described.16 In this concern, adult rats chronically fed a sucrose-rich diet showed dyslipidemia, and peripheral insulin resistance accompanied by high levels of heart and muscle triglycerides.24,25,32
The replacement of dietary n-6 linoleic acid by n-3 PUFA (from fish oil) in the SRD after weaning is able to prevent hypertriglyceridemia and liver steatosis induced by a maternal and post-weaning sucrose-rich diet. This is in agreement with the well-known effect of these n-3 PUFAs in different adult experimental models of dyslipidemia and insulin resistance.24 The reversion of hypertriglyceridemia and the high intrahepatic lipid content are coincident and would be related to the reported normalization of lipogenic enzymes and CPT I activities – the major rate-limiting enzyme in the mitochondrial entry of fatty acids and oxidation – since n-3 PUFAs are natural ligands of PPARs. The increase of PPARα protein mass levels in the SRD–FO group by n-3 PUFA could consequently trigger the activation of CPT I. Dietary fish oil after weaning also markedly prevents triglyceride storage in both muscles, heart and skeletal muscle. This fact could be a consequence of the amelioration of the plasma lipid milieu (normal levels of triglyceride and a moderate increase of free fatty acid) observed in the offspring when fish oil is present after weaning. Besides, several studies in rodents indicate that n-3 polyunsaturated fatty acids increase fat oxidation in tissues including skeletal muscle.24,53 In this regard, an increased availability of n-3 fatty acids provided by the diet could induce a fuel bifurcation in muscle that enhances beta oxidations as a preferred pathway for fatty acids instead of triglyceride synthesis. Moreover, n-3 PUFAs could also be related to the changes in the fatty acid content of membrane phospholipids in insulin-target tissues and, subsequently, might positively influence insulin action.29 Although we did not analyze glucose homeostasis in SRD–FO offspring, we cannot discard the possibility that an amelioration of glucose homeostasis could also influence lipid content in heart and skeletal muscle.
In brief, using an experimental animal model this study places emphasis on nutritional challenges – such as enriched sucrose feeding – during early offspring life-time and subsequent health risks. Moreover, the partial substitution of the source of fat in the diet (n-6 by n-3) after weaning seems to be a feasible interventional strategy that could prevent and/or reduce the outcomes induced by a sucrose-rich diet from the onset of pregnancy until adulthood in offspring.
Authors’ contributions
Conceived and designed the experiments: A. Chicco, M. A. Fortino. Performed the experiments: A. Creus, P. Illesca, G. J. Hein, S. Rodriguez. Analyzed the data: A. Chicco, M. A. Fortino. Wrote the paper: A. Chicco.
Conflict of interest
The authors have no conflicts of interest.
Funding
The present study was carried out with the financial support of University of Litoral, Grant PI 50120110100032LI (CAI + D 2012).
Acknowledgements
A preliminary report was presented at the VI Latin American Symposium on Maternal–Fetal Interaction and Placenta and V Latin American Symposium on Reproductive Immunology Meeting 2015. The authors wish to thank Dr Carlos Marra for his insightful comments and helpful assistance in the interpretation of the breast milk analysis. Thanks are also given to Walter Da Ru for his technical assistance.
References
- B. Brenseke, M. R. Prater, J. Bahamonde and J. C. Gutierrez, J. Pregnancy, 2013 DOI:101155/2013/368461.
- S. E. Ozanne and C. N. Hales, Nature, 2004, 427, 411–412 CrossRef CAS PubMed.
- M. H. Vickers, B. H. Brier, W. S. Cutfield, P. L. Hofman and P. D. Gluckman, Am. J. Physiol. Endocrinol. Metab., 2000, 279, E83–E87 CAS.
- S. A. Bayol, B. H. Simbi, R. C. Fowkes and N. C. Stickland, Endocrinology, 2010, 151, 1451–1461 CrossRef CAS PubMed.
- C. Thone-Reineke, P. Kalk, M. Dorn, S. Klaus, K. Simon, T. Pfab, M. Godes, P. Persson, T. Unger and B. Hocher, Am. J. Physiol.: Regul. Integr. Comp. Physiol., 2006, 291, R1025–R1030 CrossRef CAS PubMed.
- A. L. Rodrigues, E. G. de Moura, M. C. Pasos, S. C. Dutra and P. C. Lisboa, J. Physiol., 2009, 587, 2647–3661 CrossRef CAS PubMed.
- D. M. Sloboda, M. Li, R. Patel, Z. E. Clayton, C. Yap and M. H. Vickers, J. Obesity, 2014 DOI:10.1155/2014/203474.
- M. I. Goran, K. Dumke, S. G. Bouret, B. Kayser, R. W. Walker and B. Blumberg, Nat. Rev. Endocrinol., 2013 DOI:10.1038/nrendo.2013.108.
- M. H. Vickers, Z. E. Clayton, C. Yap and D. M. Soloboda, Endocrinology, 2011, 152, 1378–1387 CrossRef CAS PubMed.
- Y. Mukai, M. Kumazawa and S. Sato, Endocrine, 2013, 44, 79–86 CrossRef CAS PubMed.
- L. Rodriguez, M. I. Panadero, N. Roglands, P. Otero, J. J. Alvarez-Millán, J. C. Laguna and C. Bocos, J. Nutr. Biochem., 2013, 24, 1709–1716 CrossRef CAS PubMed.
- A. Soria, A. Chicco, N. Mocchiutti, R. Gutman, Y. B. Lombardo, A. Martin Hidalgo and E. Herrera, J. Nutr., 1996, 126, 2481–2486 CAS.
- C. D. Berdanier, Am. J. Clin. Nutr., 1975, 28, 1416–1421 CAS.
- A. M. Samuelsson, P. A. Matthews, E. Jansen, P. D. Taylor and L. Poston, Front. Physiol., 2013 DOI:103389/2013/00014.
- M. E. D'Alessandro, M. E. Oliva, M. R. Ferreira, D. Selenscig, Y. B. Lombardo and A. Chicco, Clin. Exp. Pharmacol. Physiol., 2012, 39, 623–629 CrossRef PubMed.
- M. E. D'Alessandro, M. E. Oliva, M. A. Fortino and A. Chicco, Food Funct., 2014, 5, 446–453 Search PubMed.
- M. H. Vickers and D. M. Sloboda, Nutr. Diet. Suppl., 2010, 2, 137–149 Search PubMed.
- L. Chen and L. G. Nyomba, Endocrinology, 2003, 144, 500–508 CrossRef CAS PubMed.
- C. S. Wyrwoll, P. J. Mark, T. A. Mori, I. B. Puddey and B. J. Waddell, Endocrinology, 2006, 147, 599–606 CrossRef CAS PubMed.
- C. S. Wyrwoll, P. J. Mark and B. J. Waddell, Hypertension, 2007, 50, 579–584 CrossRef CAS PubMed.
- I. S. Zulkafli, B. J. Waddell and P. J. Mark, Endocrinology, 2013, 154, 3110–3117 CrossRef CAS PubMed.
- M. Hou, C. Ji, J. Wang, Y. Liu, B. Sun, M. Guo, J. Buren and X. Li, J. Endocrinol., 2012, 215, 119–127 CrossRef CAS PubMed.
- C. Joffre, A. Nadjar, M. Lebbadi, F. Calon and S. Laye, Prostaglandins, Leukotrienes Essent. Fatty Acids, 2014, 91, 1–20 CrossRef CAS PubMed.
- Y. B. Lombardo and A. G. Chicco, J. Nutr. Biochem., 2006, 17, 1–13 CrossRef CAS PubMed.
- P. G. Reeves, F. H. Nielsen and G. C. Fahey Jr., J. Nutr., 1993a, 123, 1939–1951 Search PubMed.
- A. Chicco, M. E. D'Alessandro, L. Karabatas, C. Pastorale, J. C. Basabe and Y. B. Lombardo, J. Nutr., 2003, 133, 127–133 CAS.
- G. J. Hein, A. M. Bernasconi, M. A. Montanaro, M. Pellon-Maison, G. Finarelli, A. Chicco, Y. B. Lombardo and R. R. Brenner, Am. J. Physiol.: Endocrinol. Metab., 2010, 298, E429–E439 CrossRef CAS PubMed.
- A. Rossi, Y. B. Lombardo, J. M. Lacorte, A. G. Chicco, C. Rouault, G. Slama and S. W. Rizkalla, Am. J. Physiol.: Regul. Integr. Comp. Physiol., 2005, 289, R486–R494 CrossRef CAS PubMed.
- M. E. D'Alessandro, A. Chicco and Y. B. Lombardo, Prostaglandins, Leukotrienes Essent. Fatty Acids, 2013, 88, 171–177 CrossRef PubMed.
- N. Sinitskaya, S. Gourmelen, C. Schuster-Klein, B. Guardiola-Lemaitre, P. Pévet and E. Challet, Clin. Sci., 2007, 113, 417–425 CrossRef CAS PubMed.
- J. Folch, M. Lees and G. H. Sloane Stanley, J. Biol. Chem., 1957, 226, 497–509 CAS.
- Y. B. Lombardo, A. Chicco, M. E. D'Alessandro, M. Martinelli, A. Soria and R. Gutman, Biochim. Biophys. Acta, Lipids Lipid Metab., 1996, 1299, 175–182 CrossRef.
-
F. Orata, Advanced gas chromatography-Progress in agricultural, biomedical and industrial apllications, inDerivatization reacctions and reagent for gas chromatography analysis, ed. M. A. Mohd, 2012, pp. 83–108 Search PubMed.
- E. L. B. Novelli, Y. S. Diniz, C. M. Galhardi, G. M. X. Ebaid, H. G. Rodrigues, F. Mani, A. A. H. Fernandes, A. C. Cicogna and J. L. V. B. Novelli Filho, Lab. Anim., 2007, 41, 111–119 CrossRef CAS PubMed.
- S. A. Laurell, Scand. J. Clin. Lab. Invest., 1966, 18, 667–672 CrossRef.
-
Official method of analysis of Association of Official Agricultural Chemists, ed. P. Cunniff, AOAC International, Gaithersburg, Maryland, USA, 16th edn, 1999 Search PubMed.
- R. Zimmermann, G. Haemmerle, E. M. Wagner, J. G. Strauss, D. Kratkyand and R. Zechner, J. Lipid Res., 2003, 44, 2089–2099 CrossRef CAS PubMed.
- A. M. Cohen, S. Briller and E. Shafrir, Biochim. Biophys. Acta, Gen. Subj., 1971, 279, 129–138 CrossRef.
- A. P. Halestrap and R. M. Denton, Biochem. J., 1973, 132, 509–517 CrossRef CAS PubMed.
- H. Karlic, S. Lohninger, T. Koeck and A. Lohninger, J. Histochem. Cytochem., 2002, 50, 205–212 CrossRef CAS PubMed.
-
G. W. P. Snedecor and W. G. Cochran, in Factorial experiments, ed. I. A. Ames, Iowa State University Press, 1967, pp. 339–350 Search PubMed.
- C. R. Toop, B. S. Muhlhausler, K. O'Dea and S. Gentili, J. Dev. Origins Health Dis., 2015, 6, 38–46 CrossRef CAS PubMed.
- J. F. Clapp III, Proc. Nutr. Soc., 2002, 61, 45–50 CrossRef.
- M. C. Neville and M. F. Picciano, Annu. Rev. Nutr., 1997, 17, 159–184 CrossRef CAS PubMed.
- M. S. Hartvigsen, H. Mu and C.-H. Hoy, Nutr. Res., 2003, 23, 747–760 CrossRef.
- E. M. Novak and S. M. Innis, Am. J. Physiol.: Endocrinol. Metab., 2011, 301, E807–E817 CrossRef CAS PubMed.
- A. Ghusain-Choueiri and E. A. Rath, Br. J. Nutr., 1995, 74, 821–831 CAS.
- T. Priego, J. Sanchez, A. P. Garcıa, A. Palou and C. Picó, Lipids, 2013, 48, 481–495 CrossRef CAS PubMed.
- H. Guillou, D. Zadravec, P. G. P. Martin and A. Jacobsson, Prog. Lipid Res., 2010, 49, 186–199 CrossRef CAS PubMed.
- R. P. Brun and B. M. Spiegelman, J. Endocrinol., 1997, 155, 217–218 CrossRef CAS PubMed.
- B. Desvergne and W. Wahli, Endocr. Rev., 1999, 20, 649–688 CAS.
- Y. Nagai, Y. Nishio, T. Nakamura, H. Maegawa, R. Kikkawa and A. Kashiwagi, Am. J. Physiol.: Endocrinol. Metab., 2002, 282, E180–E190 CrossRef PubMed.
- G. R. Herzberg, R. T. Craig Skinner and R. Levy, Nutr. Res., 1996, 16, 639–644 CrossRef CAS.
|
This journal is © The Royal Society of Chemistry 2016 |
Click here to see how this site uses Cookies. View our privacy policy here.