DOI:
10.1039/C5GC01823D
(Paper)
Green Chem., 2016,
18, 157-164
Efficient removal of both basic and non-basic nitrogen compounds from fuels by deep eutectic solvents†
Received
6th August 2015
, Accepted 7th September 2015
First published on 7th September 2015
Introduction
The removal of nitrogen compounds (N-compounds) from fuels has been paid considerable attention over the last few years, not only because of the increasingly stringent environmental regulations on the nitrogen content in transportation fuels to reduce nitrogen oxide emission, but also due to the inhibiting effect of N-compounds on the hydrodesulphurization process of fuels.1–3 Nowadays the most popular denitrogenation method in industry is hydrodenitrogenation, but it requires harsh operation conditions, involves large cost of hydrogen resource and equipment, and is difficult to achieve high denitrogenation efficiency. In this context, it is of great necessity to develop non-hydrogenation denitrogenation technologies for the purification of fuels, such as liquid–liquid extraction and adsorption. However, because there are both basic N-compounds (e.g. pyridine, quinoline) and non-basic N-compounds (e.g. carbazole, indole and pyrrole) in the fuels, and their physicochemical characteristics are very different, such denitrogenation technologies often encounter the dilemma of failure in simultaneous efficient removal of both basic and non-basic N-compounds. Acidic extractants or adsorbents, e.g. liquid acids, metal ion-loaded solvents and framework materials could efficiently separate basic N-compounds from fuels, but their affinity to non-basic N-compounds was much lower.4–7 Other materials such as aprotic solvents could achieve efficient removal of non-basic N-compounds, but were much less effective for removing the basic ones. Another troublesome problem is the considerable loss of oils during the denitrogenation processes due to relatively low separation selectivity of N-compounds to oils. Strategies to address this problem mostly rely on the Brönsted or Lewis acid–base reactions between N-compounds and separation materials, but at the cost of increased difficulty in the recycling of materials. Besides, an environmentally undesirable large consumption of volatile solvents usually accompanies these denitrogenation processes. Recently, the development of ionic liquid-based denitrogenation technologies has received remarkable progress in addressing the above problems, owing to the attractive features of ionic liquids such as negligible volatility and tunable solvation properties.8–17 However, concerns about the multistage synthesis and purification, the relatively large cost and the possible toxicity of ionic liquids were also reported.18,19 Therefore, it remains an important and challenging task to develop new materials for fuel denitrogenation to achieve simultaneous efficient removal of both basic and non-basic N-compounds along with high selectivity, easy regeneration and environmental merits.
As a new class of solvents, deep eutectic solvents (DESs) have received more and more attention in recent years since the pioneering work of Abbott et al.20–22 DESs possess the interesting features of ionic liquids such as very low volatility and tunable physicochemical characteristics, thus providing new opportunities for the improvement of chemical processes along with much lower emission of volatile organic compounds than molecular solvents. Moreover, because DESs are usually obtained by a simple mixing of two cheap and safe compounds, in many cases they are superior to ionic liquids in terms of toxicity and cost and lead to enhanced greenness and economy of applications. Up to now, DESs have been used as efficient green solvents in a variety of applications such as catalysis, extraction, electrochemistry and biochemistry.23–31 However, the removal of N-compounds from fuels by DESs has not been reported.
Herein, we for the first time have reported a DES-based denitrogenation method of fuels that features highly efficient removal of both basic and non-basic N-compounds as well as excellent selectivity of N-compounds to fuel oils. The essence of this method is the preparation of DESs with good dual hydrogen-bond donor/acceptor ability and high polarity, which enables strong specific interactions with both basic and non-basic N-compounds and weak affinity to oils. We also showed how we could achieve such good denitrogenation performance with only a physical-extraction mechanism by tuning the properties of DESs, which avoids the dilemma of troublesome regeneration in reaction-induced denitrogenation. With the top-performing DES, choline chloride
:
phenylacetic acid (1
:
2, molar ratio), as the extractant at a DES
:
oil mass ratio of 1
:
1, the extraction efficiencies of basic pyridine and non-basic carbazole were 99.2% and 98.2%, respectively, and the pyridine-to-heptane and carbazole-to-heptane selectivity were 28
065 and 11
826, respectively, superior to those of the compared extractants. We noted that during the preparation of this work, Hizaddin et al. reported a COSMO-RS simulation study concerning the denitrogenation with DESs without any experiments and its conclusion that urea-based DESs are top-performing DESs is in contrast to the experimental conclusion of this work.32 To the best of our knowledge, this work is the first experimental research on the fuel denitrogenation with DESs, and it reveals that not all DESs are suitable for the denitrogenation of fuels and a precise control of the properties of DES is crucial for achieving efficient nitrogen-removal, high selectivity and favorable recyclability simultaneously.
Results and discussion
Extraction of N-compounds by DESs
Eight DESs with different components were synthesized to investigate the removal efficiency of N-compounds from model oil (Table 1). The obtained distribution coefficients (D) and extraction efficiencies (E) of N-compounds at 308 K are listed in Table 2, with a 1
:
1 mass ratio of DES to oil. According to the individual D and E values of pyridine and carbazole by each DES, these DESs can be divided into different groups. The first group includes one choline chloride-based DES (DES1) and two DESs without choline chloride (DES7, DES8), characterized by an almost 100% removal of pyridine from model oil but a poor ability to remove carbazole (D < 0.5; E < 35%). The second group is DES2 that showed an almost 100% removal of pyridine and a moderate efficiency of removing carbazole (D = 4.10; E = 80.4%). The third group includes DES3 and DES4, which exhibited excellent removal efficiency for both pyridine (D = 129.1, 26.1; E = 99.2%, 96.3%) and carbazole (D = 54.4, 47.1; E = 98.2%, 97.9%). And the last group includes DES-5 and DES-6, showing poor extraction ability for both pyridine (D = 1.06, 0.07; E = 51.4%, 6.5) and carbazole (D = 3.74, 0.67; E = 78.9%, 40.1%).
Table 1 Compositions and abbreviations for DESs used in this work
Component 1 |
Component 2 |
Mole ratio |
Abbreviation |
Choline chloride |
Malonic acid |
1 : 1 |
DES1 |
Choline chloride |
Glycolic acid |
1 : 1 |
DES2 |
Choline chloride |
Phenylacetic acid |
1 : 2 |
DES3 |
Choline chloride |
Phenylpropionic acid |
1 : 2 |
DES4 |
Choline chloride |
Glycerol |
1 : 2 |
DES5 |
Choline chloride |
Urea |
1 : 2 |
DES6 |
Glycerol |
Citric acid |
2 : 1 |
DES7 |
Fructose |
Malic acid |
1 : 1 |
DES8 |
Table 2 Distribution coefficient (D) and extraction efficiency (E) of carbazole and pyridine with different extractants at 308 K with a 1
:
1 mass ratio of extractant
:
oila
Extractant |
D
car
|
D
py
|
E
car
|
E
py
|
“∼+∞” means that the concentration of N-compound in the raffinate phase was too small to get an accurate value of the distribution coefficient. The corresponding E value was almost 100%.
|
DES1 |
0.53 |
∼+∞ |
34.6% |
100% |
DES2 |
4.10 |
∼+∞ |
80.4% |
100% |
DES3 |
54.4 |
129.1 |
98.2% |
99.2% |
DES4 |
47.1 |
26.1 |
97.9% |
96.3% |
DES5 |
3.74 |
1.06 |
78.9% |
51.4% |
DES6 |
0.67 |
0.07 |
40.1% |
6.5% |
DES7 |
0.000 |
∼+∞ |
0.0% |
100% |
DES8 |
0.003 |
∼+∞ |
0.3% |
100% |
DMSO |
69.5 |
3.97 |
98.6% |
79.9% |
DMF |
26.6 |
4.49 |
96.4% |
81.8% |
70% acetic acid |
1.69 |
∼+∞ |
62.8% |
100% |
[bmim][N(CN)2] |
∼+∞ |
2.81 |
100% |
73.8% |
Except for the last group, all the DESs exhibited an excellent performance of the removal of pyridine. Most of all, DES3 was highly efficient for both basic pyridine and non-basic carbazole, which is of great interest for the denitrogenation of fuels because fuels always contain both basic and non-basic N-compounds. Comparisons were made between DESs and conventional extractants. The compared conventional extractants include both polar aprotic solvents (dimethyl sulfoxide and dimethyl formamide) and organic acid (70% aqueous solution of acetic acid), as well as an ionic liquid 1-butyl-3-methylimidazolium dicyanamide ([bmim][N(CN)2]). Dimethyl sulfoxide (DMSO), dimethyl formamide (DMF) and aqueous acetic acid have been reported as classical denitrogenation extractants of fuel oils by previous studies, and [bmim][N(CN)2] is one of the most effective denitrogenation extractants among the reported ionic liquids. As presented in Table 2 and Fig. 1, all the investigated conventional extractants could only be highly efficient for one of the two N-compounds, without the ability of simultaneous efficient removal of both basic and non-basic N-compounds. The 70% aqueous acetic acid was very effective for the extraction of pyridine, with an extraction efficiency of almost 100%, but it performed much worse in the extraction of carbazole (D = 1.69; E = 62.8%). Contrarily, DMSO, DMF and [bmim][N(CN)2] showed good distribution coefficient and extraction efficiency for carbazole, but their ability to remove pyridine from model oil was much lower (D < 4.5; E < 82%). In other words, with regard to the simultaneous efficient removal of both basic N-compound and acidic N-compound, which is the requirement of practical applications, DES3 was superior to all the compared common solvents and ionic liquid in Fig. 1.
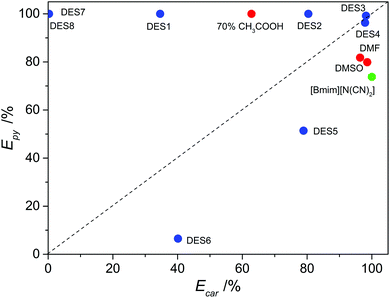 |
| Fig. 1 Extraction efficiency of pyridine (Epy) and carbazole (Ecar) by different extractants at 308 K with an extractant : oil mass ratio of 1 : 1. | |
More interesting findings were obtained from the extraction experiments besides the values of D and E. As presented in Table 2, the extraction efficiencies of pyridine by DES1, DES2, DES7 and DES8 were all almost 100%, higher than those obtained by DES3 and DES4, looking that these DESs are better solvents for removing pyridine than DES3 and DES4. However, this is not right after a careful analysis of the extraction results. When performing high-performance liquid chromatography (HPLC) analysis for the samples of the two equilibrated phases of model oil + DES3 (or DES4) systems, it was found that there was a peak of pyridine in the samples of both the oil phase and the DES phase with a good mass conservation (Fig. 2), indicating that in these systems pyridine was extracted by a physical extraction mechanism. However, when performing HPLC analysis for the samples of model oil + DES1 (or DES2, DES7, DES8) systems, although only a minimal peak of pyridine was observed in the sample of the oil phase that demonstrated an excellent removal of pyridine, there was no pyridine peak in the sample of the DES phase. This result indicated that the extracted pyridine in these DESs no longer existed in the form of pyridine molecules, but as a derivative, as a reaction product of pyridine with DESs, in other words, it underwent a reactive extraction mechanism.
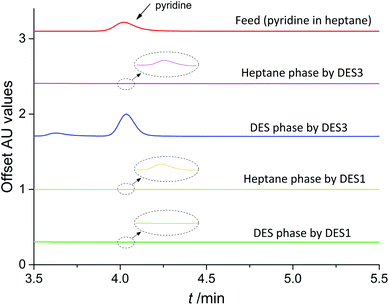 |
| Fig. 2 HPLC curves of different samples for the analysis of pyridine. | |
It is of great interest that DESs 3, 4 and DESs 1, 2, 7, and 8 exhibited a distinct extraction mechanism of pyridine although one of the components of these DESs was a carboxylic acid, especially considering that the extraction efficiencies of pyridine by non-reactive DES3 and DES4 were only slightly lower than those of reactive DESs 1, 2, 7, and 8. Because the regeneration of the extractant would be much easier in non-reactive physical extraction processes than in reactive extractions, DES3 and DES4 are more promising extractants than the reactive DESs for removing pyridine. In fact, considering that the efficient extraction of pyridine by aqueous acetic acid and other conventional acidic materials are also usually based on chemical reactions, DES3 and DES4 are still attractive even when compared with them. This phenomenon aroused our great interest in the question that whether we can rationally design DESs with such non-reactive high efficiency of removing pyridine. To shed light on this question, we investigated the relationship between the extraction performance for pyridine and the properties of DESs. Excitingly, it was discovered that whether the DES-based denitrogenation was physical extraction or chemical extraction significantly related to the proton dissociation enthalpy of the hydrogen-bond donor (HBD) component of DESs. The HBD component refers to one of the two components of DES that has a relatively strong hydrogen-bond donor ability, usually the carboxylic acids, while the other components usually serve as hydrogen-bond acceptors (HBA) in the formation of DESs with HBD. As shown in Fig. 3, when the proton dissociation enthalpy of HBD is lower than approximately 1400 kJ mol−1, all the DESs extract pyridine through chemical reactions, whereas the extractive denitrogenation by DESs becomes a physical extraction process when the proton dissociation enthalpy is higher than 1400 kJ mol−1. Moreover, an approximate linear relationship was even discovered between the proton dissociation enthalpy of HBD and the extraction efficiency of pyridine in the physical extraction region. These results strongly suggest the essential role of a precise control of the proton dissociation enthalpy of HBD (in other words the acidity of HBD) in developing efficient DESs for the removal of pyridine. A too high proton dissociation enthalpy (low acidity) will lead to poor removal efficiency of pyridine, while a too low enthalpy (high acidity) will lead to proton-transfer reaction between DESs and pyridine and thus more difficulty in regeneration of DESs.
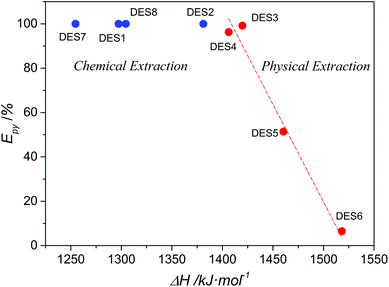 |
| Fig. 3 The extraction efficiency of pyridine (Epy) by different DESs versus the calculated proton dissociation enthalpy (ΔH) of the hydrogen-bond donor component of DESs. The red dash line stands for a linear correlation of the red points. | |
In fact, an excessive acidity of HBD in DESs probably also leads to disfavored extraction of carbazole. For DESs 1–4 that comprise choline chloride and carboxylic acid, the extraction efficiency of carbazole showed the order: DES1 < DES2 < DES3 ≈ DES4 (Table 2), which is very similar to the order of the proton dissociation enthalpy of the corresponding HBDs (Fig. 3). A higher acidity of HBD probably caused a stronger “internal” HBD–HBA interaction in DESs and thus weakened the “external” HBA ability of the DES to extract the hydrogen-bond acidic molecule, carbazole. The failure of removing carbazole by choline chloride-free DES7 and DES8 indicated the importance of the structure of the HBA component of DES. Glycerol and fructose in DES7 and DES8 are much weaker HBAs than choline chloride, thus leading to much poorer extraction of carbazole than the other choline chloride-based DESs. These analyses collectively indicate that the better denitrogenation performance of DES3 than others can be ascribed to its good dual HBD/HBA ability. Besides, it is also noted in Table 2 that the urea-based DES6 showed poor extraction efficiency for both carbazole and pyridine, which is largely different from the suggestions of conductor-like screening model for real solvent (COSMO-RS) simulations.32
Solubility of n-heptane in DESs and selectivity of N-compounds to n-heptane
To achieve an economic feasibility, an industrially attractive denitrogenation extractant must have not only high extraction efficiency of N-compounds, but also minimal solubility of oils in the extractant to obtain large nitrogen-to-oil selectivity. Therefore, the solubility of n-heptane in DES3, which showed the best nitrogen-removal efficiency among the investigated DESs, was compared with those in the conventional solvents. As presented in Fig. 4, the solubility of n-heptane in DES3 at 308 K was only 4.6 mg g−1, much smaller than those in DMSO (16.2 mg g−1) and DMF (105.44 mg g−1), and very similar to those in 70% aqueous acetic acid (3.3 mg g−1) and [bmim][N(CN)2] (4.7 mg g−1). As a consequence, among the compared extractants, only DES3 exhibited simultaneously excellent selectivity for both pyridine and carbazole to n-heptane (Fig. 5). The selectivity of pyridine to n-heptane by DES3 was 28
065, approximately 35–650 times as large as those by [bmim][N(CN)2], DMSO and DMF. The selectivity of carbazole to n-heptane by DES3 was 11
826, approximately 3–47 times as large as those by DMSO, DMF and 70% aqueous acetic acid. Therefore, compared to the investigated conventional extractants, DES3 not only had high extraction efficiency of both basic and non-basic N-compounds, but also achieved minimal solubility of n-heptane and large selectivity of N-compounds to n-heptane, which is highly attractive for the denitrogenation of fuels. The high polarity of DESs is considered responsible for the restrained solubilization of n-heptane.33,34 While taking other characters including the low volatility, good biocompatibility and easy preparation of DESs into account, the advantage of DESs becomes even more pronounced.
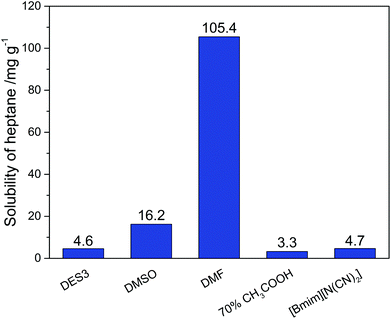 |
| Fig. 4 Solubility of n-heptane in different extractants at 308 K. | |
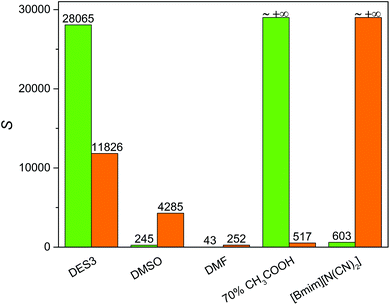 |
| Fig. 5 Selectivity of pyridine to n-heptane (green) and carbazole to n-heptane (orange) by different extractants at 308 K and an extractant : oil mass ratio of 1 : 1. | |
Effect of mass ratio on denitrogenation
The effect of the mass ratio of DES
:
oil on the denitrogenation was investigated for the extraction of pyridine and carbazole from model oils, with results presented in Fig. 6. As observed, the distribution coefficient decreased on increasing the mass ratio of DES3
:
oil, e.g., at a DES3
:
oil mass ratio of 1
:
1, the distribution coefficients of pyridine and carbazole were 129.1 and 54.4, respectively, and became 43.6 and 31.1 at a DES3
:
oil mass ratio of 1
:
5. Nevertheless, the decreased values were still considerably large for both pyridine and carbazole. The extraction efficiency was not too sensitive to the increase of the DES3
:
oil mass ratio. At a 1
:
5 DES3
:
oil mass ratio, the extraction efficiencies of pyridine and carbazole were 89.7% and 86.1%, respectively, showing that a good denitrogenation performance can be realized even with a low DES
:
oil ratio.
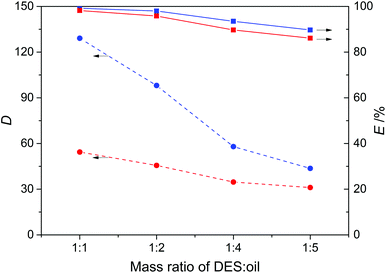 |
| Fig. 6 Effect of the mass ratio of DES3 to oil on the distribution coefficient (D, dashed lines) and extraction efficiency (E, solid lines) of pyridine (blue) and carbazole (red) at 308 K. | |
Effect of temperature on denitrogenation
In order to assess the effect of temperature, five temperature points from 293 K to 333 K were selected for the extraction of pyridine and carbazole from model oils using DES3 as the extractant. The result is given in Fig. 7, showing that both the distribution coefficients and extraction efficiency for pyridine and carbazole were not sensitive to temperature. The distribution coefficient only slightly decreased at higher temperature ranges, and the extraction efficiency was almost constant being 99% and 98% for pyridine and carbazole, respectively. These results demonstrate that the denitrogenation performance of DES3 can be attractive and stable in a wide temperature range, and also imply the non-reactive nature of the extractive removal of N-compounds by DES3 because reaction-induced chemical extractions usually have notable temperature dependence due to the more significant thermal effect of reactions.
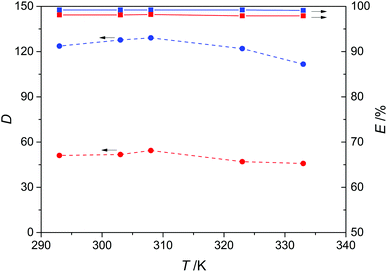 |
| Fig. 7 Effect of temperature on the distribution coefficient (D, dashed lines) and extraction efficiency (E, solid lines) of pyridine (blue) and carbazole (red) with a 1 : 1 mass ratio of DES3 to oil. | |
Effect of initial concentration
Considering that the concentration of N-compounds in various fuels is different, we investigated the effect of the initial concentration of pyridine and carbazole in model oil on denitrogenation, with a concentration range of 500–1500 ppm for pyridine and 50–200 ppm for carbazole, respectively. As presented in Fig. 8, the variations of the extraction efficiency of pyridine and carbazole were both ignorable within the investigated concentration range, signifying that the effectiveness of this denitrogenation approach is not restricted to fuel oils with a specific nitrogen concentration and is likely to be maintained even for those nitrogen-rich fuels.
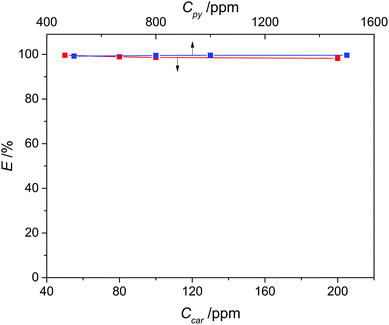 |
| Fig. 8 Effect of initial concentration of N-compounds on the extraction efficiency of pyridine (blue) and carbazole (red) with a 1 : 1 mass ratio of DES3 to oil at 308 K. | |
Regeneration of DES
The regeneration of DES was performed by stripping with n-heptane. Fig. 9 shows the extraction efficiency of pyridine and carbazole using regenerated DES3. It can be seen that, the regenerated DES3 proved as effective as a fresh one after four cycles, with almost constant values of extraction efficiency for both pyridine and carbazole. Therefore, the reusability of DES3 was demonstrated, which is attractive for the practical applications of extractive denitrogenation of fuels.
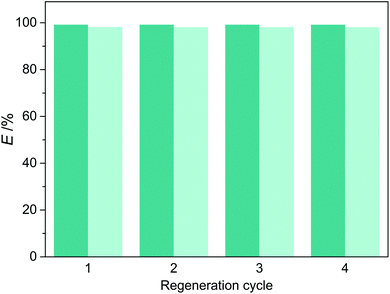 |
| Fig. 9 Extraction efficiency of pyridine (dark) and carbazole (light) in different regeneration cycles of DES3. | |
Conclusions
In summary, we reported a novel denitrogenation method of fuels using DESs as extractants. This method is capable of combining many attractive features, including a simultaneous efficient removal of both basic and non-basic N-compounds, an excellent selectivity of N-compound to oil, a physical extraction mechanism, and the “green” character and relatively low cost of materials. The structure of DESs showed a notable influence on the denitrogenation, not only on the removal ability for N-compounds, but also on the extraction mechanism. A precise control of the acidity of the HBD component in DES is crucial for the denitrogenation performance. A too low acidity leads to insufficient affinity to the basic N-compound, while a too high acidity leads to chemical reaction between DES and basic N-compound and troubled extractant regeneration. Excessive acidity of HBD and insufficient hydrogen-bond basicity of HBA would probably also lead to weak extraction of non-basic N-compound. Among the investigated DESs, the 1
:
2 molar mixture of choline chloride and phenylacetic acid (abbr. DES3) presented the best denitrogenation performance owing to its good dual HBD/HBA ability. Without chemical reactions, the extraction efficiencies of pyridine and carbazole by DES3 at 308 K with a 1
:
1 DES
:
oil molar ratio were 99.2% and 98.2%, respectively, better than the performance of DMSO, DMF, 70% aqueous acetic acid and [bmim][N(CN)2]. These high extraction efficiencies, plus the low solubility of n-heptane due to the high polarity of DES, contributed to excellent selectivity of pyridine/carbazole to n-heptane, with values higher than 10
000. The extraction efficiency was not sensitive to the DES
:
oil mass ratio and temperature, and the DES was well regenerated without the loss of efficiency even after four cycles. These results collectively demonstrate the considerable potential of DESs as promising materials for the green and efficient denitrogenation of fuels, and also are useful for the design of DESs with superior performance.
Experimental
Chemicals and materials
Phenylacetic acid (≥99.5%) and phenylpropionic acid (≥99.5%) were purchased from Shanghai Lingfeng Chemical Reagent Co. Ltd. n-Heptane (≥99%) and choline chloride (≥98%) were received from Aladdin Industrial Inc., China. Citric acid (99.5%), malic acid (≥99%), malonic acid (≥98%), carbazole (≥95%), pyridine (≥99.5%), urea (≥99%), glycerol (≥99%), fructose (≥99%), N,N-dimethylformamide (DMF, ≥99.5%), dimethyl sulfoxide (DMSO, ≥99%) and acetic acid (≥99.5%) were purchased from Sinopharm Chemical Reagent Co. Ltd. [bmim][N(CN)2] (≥99%) was purchased from Lanzhou Greenchem ILS, LICP, CAS. All reagents were used as received without further purification.
Synthesis of DESs
The two components for formation of DESs were mixed at an appropriate molar ratio in a round-bottomed flask. After that, the mixture became a transparent liquid by vigorously stirring with a magnetic stirrer at a temperature of 353–373 K for 1–1.5 h. The structure of the obtained DESs was confirmed by 1H NMR analysis. Before being used for extraction, the DESs were dried under high vacuum above their melting points for 24 h.
Extraction
In the extractive denitrogenation, model oils were prepared by dissolving an appropriate amount of pyridine (500 ppm) and carbazole (200 ppm) in n-heptane (here, ppm refers to mass fraction). Typically, the resulting model oil solutions were mixed with pure DESs at a 1
:
1 mass ratio, followed by shaking at a speed of 250 rpm in a shaker (Shanghai Zhichu ZQTY-70) for 2 h at a constant temperature and then settling for 2 h at the same temperature. The shaking time was checked to be enough for attaining the extraction equilibrium by preliminary experiments (see Fig. S1 in the ESI†). Samples of both phases were taken by using a pipette without disturbing the phase boundary, and were diluted with methanol for high performance liquid chromatography analysis.
HPLC analysis of N-compounds
HPLC was used to analyze the concentration of N-compounds in both light and heavy phases.9 The HPLC system consisted of an autosampler, Atlantis T3 (5 μm, 4.6 × 250 mm) column, a Waters 1525 binary pump and a Waters 2487 dual λ absorbance detector. The mobile phase for the detection of pyridine was 60% methanol in water (v/v, %) with a flow rate of 1.0 ml min−1, while for carbazole the mobile phase was 80% methanol in water (v/v, %) with a flow rate of 1.0 ml min−1. The detection of pyridine and carbazole was performed at a wavelength of 240 nm and 330 nm, respectively. Duplicate injections were performed for the analysis of each sample. The distribution coefficient (D) and extraction efficiency (E) were calculated by using the following equations, respectively: | 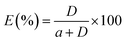 | (2) |
where wDESi and whepi denote the mass fractions of the solute in the DES phase and in the n-heptane phase, respectively, and a refers to the mass ratio of feed solution to DES.
Solubility of n-heptane and selectivity
The extractant was mixed with equal-mass pure n-heptane at 308 K for 1 h and then maintained at the same temperature to achieve a thorough phase separation. After that, a sample of the extractant phase was taken and diluted by methanol for HPLC analysis of n-heptane. The HPLC system was the same with the analysis of N-compounds, except that the dual λ absorbance detector was replaced by a refractive index detector. The mass fraction solubility of n-heptane in DES can be used to calculate the selectivity of N-compounds to n-heptane with the following equation: | S = DN-compound/Dheptane = DN-compound/(wDEShep/whephep) | (3) |
where the mass fraction of n-heptane in the DES phase wDEShep was approximately replaced by the mass fraction solubility of n-heptane in DES, and the mass fraction of n-heptane in the n-heptane phase whephep approximately equals 1.0 due to the low concentration of solutes in the n-heptane phase.
Regeneration of DES
The regeneration of DES was performed by the back extraction of N-compounds with pure n-heptane. After that, the DES was easily isolated using a separating funnel and recovered through rotary evaporation, followed by high vacuum drying at 333 K for subsequent reuse.
Calculation details
The proton dissociation enthalpy of solvents was obtained by quantum chemical calculations using Gaussian 09 D.01 software.35 At first, the geometries of solvents and their deprotonated anions were optimized at the B3LYP/6-31+G(d,p) level with DFT-D3 empirical dispersion corrections (see Fig. S2 in the ESI†).36,37 All the optimized geometries were confirmed to be minima on the potential energy surface via vibrational frequency analysis, and the enthalpy of each optimized geometry was obtained also via vibrational frequency analysis. Then, the proton dissociation enthalpy of a specific compound (ΔH) was calculated by the following equation: | ΔH = H(M−) + H(H+) − H(M) | (4) |
where H(M−) and H(M) were the calculated enthalpy of the deprotonated anion and the molecule at 298 K, respectively, and H(H+) was the proton gas-phase enthalpy under the standard conditions with a value of 6.19 kJ mol−1.38
Acknowledgements
The authors are grateful for financial support from the National Natural Science Foundation of China (21476192, 21222601 and 21436010), the Zhejiang Provincial Natural Science Foundation of China (LY15B060003) and the Fundamental Research Funds for the Central Universities of China (2015FZA4022).
References
- G. C. Laredo, P. M. Vega-Merino, F. Trejo-Zarraga and J. Castillo, Fuel Process. Technol., 2013, 106, 21 CrossRef CAS.
- I. Ahmed, N. A. Khan and S. H. Jhung, Inorg. Chem., 2013, 52, 14155 CrossRef CAS PubMed.
- S. Kumar, V. C. Srivastava, S. M. Nanoti and A. Kumar, AIChE J., 2015, 61, 2257 CrossRef CAS.
- D. Y. Han, G. X. Li, Z. B. Cao, X. Y. Zhai and M. M. Yuan, Energ. Source., Part A, 2013, 35, 622 CrossRef CAS.
- J. Qi, Y. Yan, Y. Su, F. Qu and Y. Dai, Energy Fuels, 1998, 12, 788 CrossRef CAS.
- J. Qi, Y. Yan, W. Fei, Y. Su and Y. Dai, Fuel, 1998, 77, 255 CrossRef CAS.
- I. Ahmed and S. H. Jhung, Chem. Eng. J., 2014, 251, 35 CrossRef CAS.
- A. R. Hansmeier, G. W. Meindersma and A. B. de Haan, Green Chem., 2011, 13, 1907 RSC.
- X. Chen, S. Yuan, A. A. Abdeltawab, S. S. Al-Deyab, J. Zhang, L. Yu and G. Yu, Sep. Purif. Technol., 2014, 133, 187 CrossRef CAS.
- G. C. Laredo, N. V. Likhanova, I. V. Lijanova, B. Rodriguez-Heredia, J. Jesus Castillo and P. Perez-Romo, Fuel Process. Technol., 2015, 130, 38 CrossRef CAS.
- M. Vilas, E. J. Gonzalez and E. Tojo, Fluid Phase Equilib., 2015, 396, 66 CrossRef CAS.
- H. Wang, C. Xie, S. Yu and F. Liu, Chem. Eng. J., 2014, 237, 286 CrossRef CAS.
- C. Asumana, G. Yu, Y. Guan, S. Yang, S. Zhou and X. Chen, Green Chem., 2011, 13, 3300 RSC.
- M. R. Shah, R. Anantharaj, T. Banerjee and G. D. Yadav, J. Chem. Thermodyn., 2013, 62, 142 CrossRef CAS.
- L. Alonso, A. Arce, M. Francisco and A. Soto, J. Chem. Eng. Data, 2010, 55, 3262 CrossRef CAS.
- S. Zhang, Q. Zhang and Z. C. Zhang, Ind. Eng. Chem. Res., 2004, 43, 614 CrossRef CAS.
- T. Jiao, X. Zhuang, H. He, L. Zhao, C. Li, H. Chen and S. Zhang, Green Chem., 2015, 17, 3783 RSC.
- F. Pena-Pereira and J. Namieśnik, ChemSusChem, 2014, 7, 1784 CrossRef CAS PubMed.
- R. Martinez-Palou and R. Luque, Energy Environ. Sci., 2014, 7, 2414 CAS.
- A. Paiva, R. Craveiro, I. Aroso, M. Martins, R. L. Reis and A. Duarte, ACS Sustainable Chem. Eng., 2014, 2, 1063 CrossRef CAS.
- A. P. Abbott, D. Boothby, G. Capper, D. L. Davies and R. K. Rasheed, J. Am. Chem. Soc., 2004, 126, 9142 CrossRef CAS PubMed.
- Y. T. Dai, J. van Spronsen, G. J. Witkamp, R. Verpoorte and Y. H. Choi, Anal. Chim. Acta, 2013, 766, 61 CrossRef CAS PubMed.
- K. Pang, Y. C. Hou, W. Z. Wu, W. J. Guo, W. Peng and K. N. Marsh, Green Chem., 2012, 14, 2398 RSC.
- W. Zhu, C. Wang, H. Li, P. Wu, S. Xun, W. Jiang, Z. Chen, Z. Zhao and H. Li, Green Chem., 2015, 17, 2464 RSC.
- A. P. Abbott, R. C. Harris, K. S. Ryder, C. D'Agostino, L. F. Gladden and M. D. Mantle, Green Chem., 2011, 13, 82 RSC.
- Q. Zhang, K. De Oliveira Vigier, S. Royer and F. Jérôme, Chem. Soc. Rev., 2012, 41, 7108 RSC.
- C. Li, D. Li, S. Zou, Z. Li, J. Yin, A. Wang, Y. Cui, Z. Yao and Q. Zhao, Green Chem., 2013, 15, 2793 RSC.
- F. S. Oliveira, A. B. Pereiro, L. P. N. Rebelo and I. M. Marrucho, Green Chem., 2013, 15, 1326 RSC.
- H. Liao, Y. Jiang, Z. Zhou, S. Chen and S. Sun, Angew. Chem., Int. Ed., 2008, 47, 9100 CrossRef CAS PubMed.
- G. García, S. Aparicio, R. Ullah and M. Atilhan, Energy Fuels, 2015, 29, 2616 CrossRef.
- Y. Dai, G. Witkamp, R. Verpoorte and Y. H. Choi, Anal. Chem., 2013, 85, 6272 CrossRef CAS PubMed.
- H. F. Hizaddin, A. Ramalingam, M. A. Hashim and M. K. O. Hadj-Kali, J. Chem. Eng. Data, 2014, 59, 3470 CrossRef CAS.
- A. Pandey, R. Rai, M. Pal and S. Pandey, Phys. Chem. Chem. Phys., 2014, 16, 1559 RSC.
- A. Pandey and S. Pandey, J. Phys. Chem. B, 2014, 118, 14652 CrossRef CAS PubMed.
-
M. J. Frisch, G. W. Trucks, H. B. Schlegel, G. E. Scuseria, M. A. Robb, J. R. Cheeseman, G. Scalmani, V. Barone, B. Mennucci, G. A. Petersson, H. Nakatsuji, M. Caricato, X. Li, H. P. Hratchian, A. F. Izmaylov, J. Bloino, G. Zheng, J. L. Sonnenberg, M. Hada, M. Ehara, K. Toyota, R. Fukuda, J. Hasegawa, M. Ishida, T. Nakajima, Y. Honda, O. Kitao, H. Nakai, T. Vreven Jr., J. A. Montgomery, J. E. Peralta, F. Ogliaro, M. Bearpark, J. J. Heyd, E. Brothers, K. N. Kudin, V. N. Staroverov, R. Kobayashi, J. Normand, K. Raghavachari, A. Rendell, J. C. Burant, S. S. Iyengar, J. Tomasi, M. Cossi, N. Rega, J. M. Millam, M. Klene, J. E. Knox, J. B. Cross, V. Bakken, C. Adamo, J. Jaramillo, R. Gomperts, R. E. Stratmann, O. Yazyev, A. J. Austin, R. Cammi, C. Pomelli, J. W. Ochterski, R. L. Martin, K. Morokuma, V. G. Zakrzewski, G. A. Voth, P. Salvador, J. J. Dannenberg, S. Dapprich, A. D. Daniels, Ö. Farkas, J. B. Foresman, J. V. Ortiz, J. Cioslowski and D. J. Fox, Gaussian 09, Revision D.01, Gaussian, Inc., Wallingford,
CT, 2009 Search PubMed.
- C. T. Lee, W. T. Yang and R. G. Parr, Phys. Rev. B: Condens. Matter, 1988, 37, 785 CrossRef CAS.
- S. Grimme, J. Antony, S. Ehrlich and H. Krieg, J. Chem. Phys., 2010, 132, 154104 CrossRef PubMed.
- R. Vessecchi and S. E. Galembeck, J. Phys. Chem. A, 2008, 112, 4060 CrossRef CAS PubMed.
Footnote |
† Electronic supplementary information (ESI) available: Figures showing the effect of shaking time on denitrogenation and optimized geometries of molecules and anions. See DOI: 10.1039/c5gc01823d |
|
This journal is © The Royal Society of Chemistry 2016 |
Click here to see how this site uses Cookies. View our privacy policy here.