DOI:
10.1039/C6GC90040B
(Editorial)
Green Chem., 2016,
18, 3180-3183
Green chemistry and resource efficiency: towards a green economy
Received
24th March 2016
, Accepted 24th March 2016
The impact that the second principle of green chemistry – Synthetic methods should be designed to maximize the incorporation of all materials in the process into the final product – has had over the last 25 years on the fundamental values and efficiency of chemicals manufacture is reviewed. It has played a major role in focusing the attention of the chemical industry world-wide, and particularly the pharmaceutical industry, on the problem of waste generation in chemicals manufacture. It precipitated a paradigm shift in how efficiency in organic synthesis is assessed and it provided the impetus for developing cleaner, more sustainable processes. The principle is also at the heart of the current drive towards a waste-free, circular economy.
1. Introduction: origins of green chemistry and waste minimisation
Green chemistry is basically concerned with the prevention of pollution by waste minimisation and the avoidance of toxic and hazardous substances in the production and application of chemical products. Its origins can be traced back to the environmental movement of the 1960s. In particular, publication of the books, Silent Spring by Rachel Carson in 19621 and The Closing Circle: Nature, Man and Technology by Barry Commoner in 1971,2 focused attention on the harmful side-effects of man-made chemical products, such as the insecticide DDT, and the devastating effects of pollution from chemical manufacturing processes on our natural environment, respectively. However, the solution to these problems is not less chemistry but the substitution of undesirable chemical products and processes by cleaner, safer and environmentally friendlier alternatives. In the 1980s emphasis was placed more and more on pollution abatement by waste prevention at source as opposed to waste remediation and pollution control by end-of-pipe solutions. The adoption of the US Pollution Prevention Act in 19903 focused industry, government, and public attention on the need to reduce the amount of pollution through cost-effective changes in operating procedures and raw materials use. It was noted that reducing waste at the source not only minimises the cost of its treatment but can actually strengthen economic competitiveness through more efficient use of raw materials. It heralded a fundamental change in the approach of the US Environmental Protection Agency (EPA) to protecting human health and the environment from one of “end of pipe” waste treatment to one of preventing waste generation, and planted the seed for the introduction of the term green chemistry at the EPA in the early 1990s. Apparently the time was ripe for its introduction as indicated by the publication of a paper with the title ‘Green chemistry – dream or reality (minimum impact chemistry)’ in a Czech journal in 1991.4
2. Resource efficiency, atom economy and the E factor
Green chemistry gained formal recognition with the publication of the 12 Principles of Green Chemistry by Anastas and Warner in 19985 in which the overall guiding element is ‘benign by design’ (Fig. 1). The second principle, ‘Synthetic methods should be designed to maximise the incorporation of all materials in the process into the final product’, is concerned with resource efficiency and waste minimisation. By optimising the efficiency of resource utilisation one is, at the same time, minimising waste generation. This is the essence of Trost's landmark paper, ‘The atom economy: a search for synthetic efficiency’, published in 19916 and celebrating its 25th anniversary this year. Atom economy (AE) is calculated by dividing the molecular weight of the product by the sum total of the molecular weights of all substances formed in the stoichiometric equation for the reaction involved. AE is a theoretical number which assumes the use of exact stoichiometric quantities of starting materials and a chemical yield of 100%, and disregards substances, such as solvents and chemicals used in the work-up of the reaction mixture, which do not appear in the stoichiometric equation. Nonetheless, it is an extremely useful tool for rapid evaluation, before any experiments are performed, of the resource efficiency and, by the same token, the amounts of waste that will be generated in alternative processes.
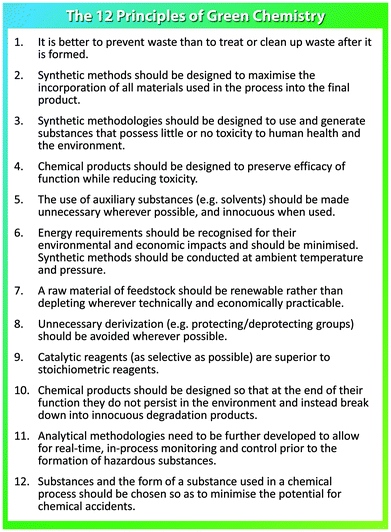 |
| Fig. 1 The 12 Principles of Green Chemistry. | |
At the same time, in the late 1980s, our attention was drawn to the enormous problem of waste generation in the fine chemical and pharmaceutical industries based on our hands-on experience with the industrial production of the pharmaceutical intermediate, phloroglucinol, from 1,3,5-trinitrotoluene (TNT) in a process which generated 40 kg of chromium-containing waste for every kg of product.7 Subsequently, based on an inventory of the amounts of waste formed in the manufacture of other fine chemicals and pharmaceuticals we concluded that tens of kgs of waste per kg of product were no exception in these industries. We concluded that a new paradigm was needed for efficiency in organic synthesis, one that assigned value to waste minimisation. Clearly, an environmental factor was missing. This led us to propose what we called the E(nvironmental) factor (kgs waste per kg product) for assessing the environmental footprint of manufacturing processes and the now well-known ‘Table of E Factors’ was used to illustrate the enormity of the waste problem in various segments of the chemical industry.8 The E factor differs from AE in two important ways. First, it takes into account all of the auxiliary components – waste solvent, waste from chemicals used in work-up, etc. – which are disregarded by AE. Second, AE is applied to individual steps but the E factor can easily be applied to a multi-step process thus facilitating a holistic assessment of a complete process.
Other mass metrics have been proposed, notably Process Mass Intensity (PMI)9 which is the ratio of kgs materials input, including process water, to kgs product and is equal to E + 1. However, as we have recently explained,10 the ideal PMI is 1 and the ideal E factor of zero is a better reflection of the goal of green chemistry: zero waste. Moreover, the E factor is mathematically more suitable for evaluating a multi-step process since step E factors are additive while step PMIs are not because PMI does not discount the step product from the mass balance. In our original publication we defined waste as “anything that is not the desired product”, with the exception of water. Our rationale for excluding water was the that including it could lead to a distortion of E factors, thus rendering meaningful comparison of processes difficult. However, the current trend in the pharmaceutical industry is to include water in the E factor and in an assessment of a biocatalytic process for atorvastatin we calculated the E factor with and without water.11 Energy was implicitly included in the E factor since its input results in the formation of waste, mainly in the form of carbon dioxide. However, in practice it was difficult to include it in E factors of the manufacture of fine chemicals and active pharmaceutical ingredients (APIs) which often involve multipurpose production facilities where allocation of energy costs to particular products is difficult. In contrast, energy costs and CO2 generation can't be disregarded in the production of commodity chemicals in the bio-based economy (see later).
Lower E factors are a reflection of lower material inputs, reduced costs from waste disposal, reduced energy demand and improved utilisation of manufacturing capacity. Hence, there are substantial economic benefits to be gained by integrating green chemistry into process R & D and manufacturing. Green chemistry is not only good for the environment, it makes good business sense. Undoubtedly, the pharmaceutical industry has, over the past two decades, made great strides in incorporating green chemistry and engineering philosophies into process development to achieve significant reductions in their E factors with concomitant economic and environmental benefits.
3. The role of catalysis in waste minimisation
A major source of the waste generated in organic synthesis is the use of stoichiometric inorganic or organic reagents, for example in oxidations, reductions and a multitude of other reactions. Twenty five years ago such time-honoured ‘stoichiometric methodologies’ were the norm in the manufacture of fine chemicals and APIs. Taken together with the fact that their synthesis generally involves multistep processes owing to the molecular complexity of APIs, this readily explains the large E factors observed in the pharmaceutical industry. Hence, the solution to the waste problem was evident: substitution of archaic atom inefficient stoichiometric methodologies with greener catalytic alternatives. Indeed, the widespread adoption, over the last two decades, of catalytic methodologies by process chemists in the pharmaceutical and fine chemical industries has been a major contributing factor in reducing the E factors of processes in these industries. Examples which readily come to mind are olefin metathesis, olefin hydroformylation, carbonylations, and (asymmetric) hydrogenations, to name but a few.
Biocatalysis also has numerous environmental and economic benefits to offer. The catalyst (an enzyme) is derived from renewable resources and is biocompatible, biodegradable and essentially non-hazardous and non-toxic. Biocatalysis avoids the use of scarce precious metals, the long term commercial viability of which is questionable. Moreover, the costs of removing traces of noble metal catalysts, to an acceptable level, from end products can be prohibitive. Enzymatic reactions are performed under mild conditions (physiological pH and ambient temperature and pressure) often without the need for functional group activation and protection and deprotection steps required in conventional organic syntheses. This translates to shorter syntheses and higher selectivities, affording products in higher purity in processes which are more efficient in energy and raw materials and generate less waste than conventional routes. It is not surprising, therefore, that in the last decade biocatalysis has been more and more integrated into mainstream organic synthesis, particularly with regard to the synthesis of APIs12
4. Renewable resources, the bio-based economy and waste valorisation
Another important goal of green chemistry and resource efficiency is the utilisation of renewable raw materials in the sustainable production of fuels and commodity chemicals from biomass,13,14 as opposed to their unsustainable production from non-renewable fossil resources such as oil, coal and natural gas. In order to be sustainable a technology must fulfill two conditions: (i) natural resources should be used at rates that do not unacceptably deplete supplies over the long term and (ii) residues should be generated at rates no higher than can be assimilated readily by the natural environment.15 It is abundantly clear that non-renewable fossil resources – oil, coal and natural gas – are being used at a much higher rate than they can be replaced by natural geological processes, making their use unsustainable in the long term. Furthermore, the use of fossil resources is generating carbon dioxide at rates that can't be assimilated by the natural environment, leading to increased carbon dioxide levels in the atmosphere, which are generally believed to be a direct cause of climate change. In contrast, the direct conversion of plant biomass to biofuels, chemicals, and biomaterials, in integrated biorefineries, forms the basis for a carbon neutral, bio-based economy. This can be viewed simply as another aspect of resource efficiency and waste minimisation. In the non-renewable carbon cycle of fossil resources there is an enormous production of carbon dioxide as waste whereas in the carbon cycle based on renewable plant biomass there is, in principle, no net production of carbon dioxide, that is waste is minimised and resource efficiency is optimised. A further benefit of a switch to renewable biomass as a feedstock could be the substitution of existing products by inherently safer alternatives with reduced environmental footprints, such as biocompatible and biodegradable plastics.16
However, in the EU at least, the use of first generation biomass feedstocks, such as corn and edible oil seeds is not seen as a sustainable option because of direct or indirect competition with food production. A more attractive scenario for the EU is the valorisation of waste biomass,17 notably agricultural and forestry residues, by employing green, resource efficient chemo- and biocatalytic processes.18 Global production of sugar cane bagasse, corn stover, wheat straw and rice straw, for example, is in the hundreds of millions of tonnes per annum,19 amounts which far exceed the annual production of basic petrochemicals. Two decades ago, the advent of green chemistry focused attention on waste prevention at source but it is now acknowledged that in some cases, such as agriculture and forestry, there is a definite need to create value from unavoidable waste. This is also tantamount to maximising resource efficiency in the true spirit of green chemistry and fits extremely well with current thinking regarding the so-called circular economy.20
5. Resource efficiency by design: towards a green, circular economy
As noted above, the essence of the second principle of green chemistry is design for resource efficiency. Increased interest in green and sustainable growth, coupled with growing concern for climate change, has focused attention on resource efficiency. This is stimulating the much needed move from a traditional linear flow of materials in a ‘take-make-use-dispose’ economy, to a greener, circular one which seeks to eliminate waste through deliberate design of products, processes with resource efficiency and recycling in mind. This philosophy forms the basis of the European Commission's “Roadmap to a resource efficient Europe”.21
Barry Commoner already recognised the linear vs. circular economy issue in the 1960s when he observed:2 “We have broken out of the circle of life, converting its endless cycles into man-made linear events: oil is taken from the ground, distilled into fuel, burned in an engine, converted thereby into noxious fumes which are emitted in the air”. However, the necessary transition from an unsustainable linear economy to a greener circular one is hampered by the fact that economic assessments are not being conducted on a level playing field. The true costs of the established ‘take-make-use-dispose’ production chains have to include the costs of resource usage/depletion, waste management and environmental pollution, which are currently externalised. They need to be internalised and new economic indicators are needed that take resource efficiency and circularity into account. We need to rethink how we can close the loops of production chains and optimise resource efficiency.
6. Concluding remarks
Over the last 25 years the concept of green chemistry, in particular the principle of designing synthetic methods to maximise the incorporation of all raw materials in the product, and the underpinning metrics, atom economy and the E factor, have been widely embraced by industry and academia worldwide. The shift towards green chemistry and resource efficiency is accelerating and has been supplemented by the drive towards substitution of the unsustainable use of fossil resources by sustainable use of renewable biomass with the help of green chemo- and biocatalytic processes. Looking to the future, an important spin-off of this trend will be the development of alternative, more sustainable products and, ultimately, the design of products with their regeneration and circularity of production in mind, in a truly green economy.
References
-
R. Carson, Silent Spring, Houghton Mifflin, Boston, 1962 Search PubMed.
-
B. Commoner, The Closing Circle: Nature, Man and Technology, Knopf, New York, 1971 Search PubMed.
-
http://www.epa.gov/laws-regulations/summary-pollution-prevention-act
.
- P. Drasar, Chem. Listy, 1991, 85(11), 1144–1149 CAS.
-
P. Anastas and J. C. Warner, Green Chemistry: Theory and Practice, Oxford University Press, Oxford, 1998 Search PubMed.
- B. M. Trost, Science, 1991, 254, 1471–1477 CAS.
- R. A. Sheldon, Green Chem., 2007, 9, 1273–1283 RSC.
- R. A. Sheldon, Chem. Ind., 1992, 903–906 CAS.
- C. Jimenez-Gonzalez, C. S. Ponder, Q. B. Broxterman and J. B. Manley, Org. Process Res. Dev., 2011, 15, 912–917 CrossRef CAS.
- F. Roschangar, R. A. Sheldon and C. H. Senanayake, Green Chem., 2015, 17, 752–768 RSC.
- S. K. Ma, J. Gruber, C. Davis, L. Newman, D. Gray, A. Wang, J. Grate, G. W. Huisman and R. A. Sheldon, Green Chem., 2010, 12, 81–86 RSC.
- G. W. Huisman and S. J. Collier, Curr. Opin. Chem. Biol., 2013, 17, 284–292 CrossRef CAS PubMed.
-
Catalytic Process Development for Renewable Materials, ed. P. Imhof and J. C. van der Waal, Wiley-VCH, Weinheim, 2013 Search PubMed.
- R. A. Sheldon, Green Chem., 2014, 16, 950–963 RSC.
-
T. E. Graedel, in Handbook of Green Chemistry and Technology, ed. J. Clark and D. J. Macquarrie, Wiley, New York, 2002, pp. 56–61 Search PubMed.
- E. De Jong, A. Higson, P. Walsh and M. Wellisch, Biofuels, Bioprod. Biorefin., 2012, 6, 606–624 CrossRef CAS.
- C. O. Tuck, E. Perez, I. T. Horvath, R. A. Sheldon and M. Poliakoff, Science, 2012, 337, 695–699 CrossRef CAS PubMed.
- R. A. Sheldon, J. Mol. Catal. A: Chem., 2016 DOI:10.1016/j.molcata.2016.01.013.
- J. K. Saini, R. Saini and L. Tewari, 3 Biotech., 2015, 5, 337–353 CrossRef.
-
http://www.ellenmacarthurfoundation.org/publications
.
- Communication from the Commision to the European Parliament, the Council, the European Economic and Social Committee and the Committee of the Regions, 2011, Roadmap to a Resource Efficient Europe, COM/2011/0571 final.
|
This journal is © The Royal Society of Chemistry 2016 |
Click here to see how this site uses Cookies. View our privacy policy here.