DOI:
10.1039/C5RA21977A
(Paper)
RSC Adv., 2016,
6, 4136-4144
Plasmonic paper: a porous and flexible substrate enabling nanoparticle-based combinatorial chemistry†
Received
21st October 2015
, Accepted 23rd December 2015
First published on 23rd December 2015
Abstract
The intrinsic properties of nanoparticles are both fundamentally interesting and offer tremendous potential for a variety of applications. However, leveraging their utility is difficult because these properties are often intimately connected to nanoparticle stability in solution. We report that cellulose filter paper is a versatile substrate to capture, stabilize, and manipulate nanoparticles. Once immobilized, we present ensemble averaged extinction spectra and propose that this plasmonic paper is a solid state equivalent to a nanoparticle solution. We also demonstrate that capillary flow within the paper creates concentration gradients of reagents for combinatorial chemical reactions on the immobilized nanoparticles. Importantly, the added stability afforded to the nanoparticles by the paper allows for exposure to chemical environments that would otherwise disrupt a nanoparticle colloid. These new capabilities could enable new architectures in nanoparticle-based sensors and biodiagnostics where many of the previous design limitations are entirely avoided.
Introduction
Advances in morphological control during nanoparticle syntheses have given rise to increasingly sophisticated structures to include rods,1,2 cubes,3 concave cubes4 and cages.5–7 These new structures have the potential to profoundly impact the areas of chemical sensing,8–10 catalysis,11 biodiagnostics,12,13 bioimaging14,15 and therapy.16–18 The intrinsic properties of nanomaterials have already enabled a variety of fundamental investigations and applications in research laboratories.19–23 However, in order to leverage their intrinsic properties, nanomaterials typically require modification with functional ligands. Perturbation during processing and handling often results in aggregation, which ultimately renders nanomaterial properties inaccessible. Consequently, developing surface modification strategies that also circumvent aggregation is necessary and researchers have gone to great lengths to address this challenge. Indeed, a current state-of-the-art “toolbox” of compatible reagents has been developed to impart stability and functionality which includes ligands such as DNA,24,25 polymers,26 peptides,27–29 etc. However, device architectures that require transitioning these colloidal structures from solution into the solid state, without a loss of their intrinsic properties, remain difficult to realize because particles often aggregate during the transfer process.
Paper was previously selected to serve as the substrate for a number of nanoparticle-based sensing applications10,30–34 because of its versatility as a substrate for the production of low-cost biodiagnostic and detection platforms.35,36 Importantly, the optical properties of nanoparticles on a substrate will resemble those in solution only when the nanoparticles adopt a random configuration since these properties are polarization dependent. Although nanoparticles have been immobilized on glass and other planar substrates,16,37,38 the 3-dimensional network of cellulose fibers in paper enables a random arrangement of particles and facilitates the interrogation of all plasmon modes. This capability becomes significant where structure–function investigations of nanoparticle reactivity depend on probing all available particle orientations and facets to a particular chemical environment. Here, we report a comprehensive investigation of the material previously dubbed “plasmonic paper”, and present new insight into its utility as a substrate for the capture, characterization, and manipulation of nanoparticles. This research has allowed us to expand on previously reported uses of plasmonic paper,10,32,39,40 to include effects of chemical environment and aging on nanoparticle properties. This new understanding ultimately leads to the ability to perform combinatorial chemical reactions on the imbedded nanoparticles by leveraging the wettable paper substrate.
Methods
Synthesis of gold nanorods (AuNRs)
Gold nanorods were synthesized using a seed-mediated approach.41 Seed solution was prepared by adding 0.6 mL of an ice-cold sodium borohydride solution (10 mM) into 10 mL of 0.1 M cetyltrimethylammonium bromide (CTAB) and 2.5 × 10−4 M chloroauric acid (HAuCl4) solution under vigorous stirring at 25 °C. The color of the seed solution changed from yellow to brown. Growth solution was prepared by mixing 95 mL of CTAB (0.1 M), 0.5 mL of silver nitrate (10 mM), 4.5 mL of HAuCl4 (10 mM), and 0.55 mL ascorbic acid (0.1 M) consecutively. The solution was homogenized by gentle stirring. To the resulting colorless solution, 0.12 mL of freshly prepared seed solution was added and set aside in the dark overnight. Prior to use, the AuNRs solution was redispersed in nanopure water (18.2 MΩ cm). Spherical gold nanoparticles and gold concave cubes were synthesized using previously reported protocols with no changes.4,42
Plasmonic paper preparation
Common laboratory filter paper (Whatman™ #1) was used for particle adsorption to form plasmonic paper. Briefly, 1 cm × 1 cm paper swatches were taken and immersed in solutions of nanoparticles at ≈2OD at λmax and left overnight at room temperature. The paper strip was removed from solution, washed with water and dried under nitrogen gas for further characterization.
Polyelectrolyte coating
For coating AuNRs with polyelectrolyte, a 5 mL solution of twice-centrifuged AuNR solution was added drop-wise to 5 mL of poly(sodium styrene sulfonate) (PSS) (70 kDa, 0.2% w/v) in NaCl aqueous solution (6 mM) under vigorous stirring, followed by stirring for 3 hours. To remove excess PSS, the above solution was centrifuged at 8600 rcf for 10 min, and the pellet was dispersed in nanopure water after removing the supernatant. To modify AuNRs with poly(allylamine hydrochloride) (PAH), 5 mL of PSS coated AuNR solution was added drop-wise to 5 mL of PAH (100–180 kDa, 0.2% w/v) in 6 mM NaCl aqueous solution, followed by stirring for 3 hours. Paper substrates (1 cm × 1 cm) were incubated in these polyelectrolyte coated AuNRs to form a uniform deposition.
PEG coating
For coating AuNR with PEG, 1 mL of twice centrifuged AuNRs was exposed to 200 μL of 2 mM SH–PEG and 100 μL of 2 mM K2CO3. The resultant solution was sonicated for one hour and centrifuged at 8000 rpm for 10 minutes. The centrifuged pellet was redispersed in water and is used to adsorb on filter paper.
Synthesis of Au@AgNCs
2 mL twice-centrifuged AuNPs and 4 mL CTAC (20 mM) were mixed at 60 °C under stirring for 20 min. 5 mL AgNO3 (2 mM), 2.5 mL CTAC (80 mM) and 2.5 mL ascorbic acid (0.1 M) were added under stirring at 60 °C for 4 h. Subsequently, the as synthesized Au@AgNP solution was cooled by immersing the reaction vial in ice-cold water.
Plasmonic ink
The Au@AgNCs are twice centrifuged at 8000 rpm and concentrated is used as plasmonic ink. 10 μL of this pellet was injected into a ball-point pen that is cleaned with ethanol and water. The ball-point pen filled with plasmonic ink was used to draw a straight line with 5 strokes on a filter paper.
Combinatorial chemistry
The paper substrate with 5 strokes of Au@Ag nanocubes was then used to do the combinatorial chemistry experiment. A 10 μL solution of 0.5 mM HAuCl4 was applied to the tip of the paper at 40°. The wicking of solvent by the paper allows for gold nanorattle formation where the extent of reaction depends on local concentration of Au3+. On the other side of the paper, a 10 μL solution of 3% H2O2 is introduced. Due the differential etching of the Au@Ag nanocubes, AuNPs are formed to different extents.
Electron microscopy
Transmission electron microscopy micrographs were recorded on a JEM-2100F (JEOL) field emission instrument. Samples were prepared by drying a drop of the solution on a carbon coated grid, which had been previously made hydrophilic by glow discharge. Scanning electron microscope images were obtained using a FEI Nova 2300 Field Emission SEM at an accelerating voltage of 10 kV. Gold sputtering (60 s) was used to coat the paper substrate prior to imaging.
Atomic force microscopy
Atomic force microscopy was performed using a Bruker Dimension Icon in tapping mode under ambient conditions. For imaging, silicon cantilevers (Nanosensors SSS-NCHR) with spring constants of ∼42 N m−1 and resonance frequency of 330 kHz were used. Scan rates were ∼0.5 Hz with 512 data points per line. Scan sizes ranged from tens of microns to hundreds of nanometers. At larger length scales, nanoparticle features were indistinguishable from the paper fibers. At scan sizes of ∼5 μm, nanoparticles on the paper could be observed.
Extinction spectra measurements
Extinction spectra from paper substrates were collected using a CRAIC microspectrophotometer (QDI 302) coupled to a Leica optical microscope (DM 4000M) with 20× objective in the range of 450–800 nm with 10 accumulations and 100 ms exposure time in reflection mode. The spectral resolution of the microspectrophotometer is 0.2 nm. Multiple UV-visible spectra (∼10) were collected from different locations of the hydrated paper strip to assure homogeneity. In refractive index sensitivity (RIS) experiments, the measurements were taken on hydrated paper or paper soaked in a sucrose solution. In combinatorial experiments, the paper was dried to prevent convolution of results from any unreacted materials remaining on the substrate. A Shimadzu UV-1800 spectrophotometer was employed to collect UV-vis extinction spectra from solution. Analysis was completed using OriginPro software. Raw spectra were imported and signal processed using an adjacent averaging smoothing algorithm to remove extraneous noise within the measurement. Where solution extinction measurements are compared to on the plasmonic paper substrate, sample backgrounds were subtracted for each case.
Results and discussion
In a typical experiment, nanoparticles were immobilized onto a swatch of Whatman™ #1 filter paper by immersing the substrate into a solution of nanoparticles and rotating overnight (Fig. S1a†). The association of the particles to the paper was obvious, since the color intensity of the nanoparticle solution decreased and was essentially transferred to the substrate to during the course of the experiment (Fig. S1a and b†). The transfer process was confirmed by comparing the extinction of the nanoparticles solution before and after the adsorption period (Fig. S1c†). Empirically, it was observed that the nanoparticle loading onto the paper was proportional to both their concentration in solution, and the immobilization time. However, these observations have not been quantified, and are the subject of further investigations. In order to demonstrate that this approach was both facile and generalizable, it was imperative to show that particles of different shapes, sizes and surfactant chemistries could easily be incorporated onto the paper substrate and subsequently characterized. To this end, three different particle shapes and sizes were synthesized using previously reported methods (Fig. S2†). In particular, we examined gold concave cubes,4 nanorods1,41 and spheres.42 Following synthesis, we characterized the morphology of the nanoparticles by transmission electron microscopy (TEM), to confirm that the nanoparticle shapes were of the expected morphology (Fig. 1a–c).
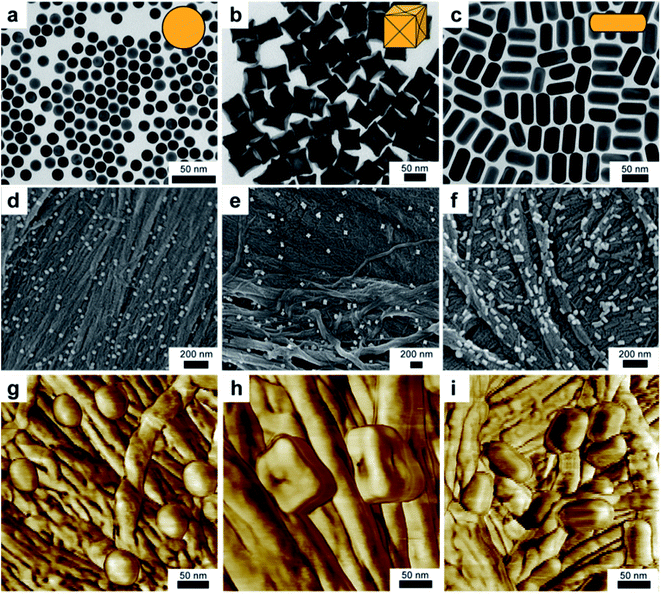 |
| Fig. 1 Transmission electron micrographs of precursor gold nanoparticles: (a) spheres, (b) concave nanocubes and (c) nanorods. Large area scanning electron micrographs showing the same batch of (d) spheres, (e) concave cubes and (f) nanorods after immobilization on the filter paper surface. Phase contrast tapping-mode atomic force microscopy showing high resolution imaging capabilities for (g) spheres, (h) concave cubes and (i) nanorods. | |
Whatman™ #1, being pervasive within research labs, was selected for the bulk of these experiments, however a variety additional papers were interrogated (Fig. S3†). The apparent bulk association of the particles with the substrate prompted further characterization of plasmonic paper. Micrographs from scanning electron microscopy (SEM) show that the particles are well distributed throughout the paper, regardless of particle shape (Fig. 1d–f). Because cellulose paper is a non-conductive substrate, a thin conductive layer of gold was sputtered onto the surface to facilitate imaging by electron microscopy. While it is possible to characterize the substrate at lower magnifications, degradation of the paper by the electron beam precludes high-resolution imaging. This deficiency can be addressed by augmenting characterization with phase contrast tapping mode atomic force microscopy (AFM, Fig. 1g–i). Although distinguishing nanoparticle morphology on the paper substrate can be difficult at the length scales readily afforded SEM (Fig. 1d and e), nanoparticle geometry is clearly apparent in AFM images (Fig. 1g and h).
Within the nanoparticle research community, the intrinsic optical properties of gold nanorods are well characterized.43 The longitudinal plasmon resonance band progressively red shifts with increasing aspect ratio of the nanorods. In fact, the peak wavelength of longitudinal band exhibits a linear relation with the aspect ratio (AR)44 given by λmax = 95AR + 420. Using this relationship as a reference for comparison, the optical properties of substrates functionalized with nanorods of different aspect ratios were characterized using a UV-vis microspectrophotometer (Fig. 2a). Notably, the nanorods retain their shape and optical properties following their adsorption onto paper because the familiar red-shift is observed in the longitudinal dipolar plasmon resonance.
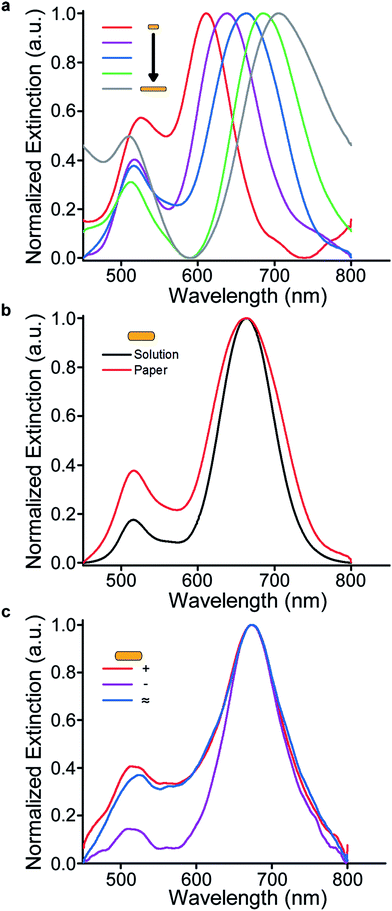 |
| Fig. 2 (a) Normalized extinction spectra of plasmonic paper using gold nanorods of increasing aspect ratio. (b) Normalized extinction spectra comparing LSPR peak position from a nanorod solution to a hydrated swatch of plasmonic paper containing the same nanorods. (c) Normalized extinction spectra illustrating adsorption of positively charged, negatively charged, and neutral nanorods onto the paper. | |
Conveniently, paper is a porous and wettable substrate. Because the nanoparticles reside on the paper fibers, the vast majority of the medium that surrounds them is the content of the interstitial regions. The refractive index of the medium surrounding the nanoparticles can be made equivalent to an analogous nanoparticle solution, simply by hydrating the paper. To highlight the extent of their similarity, the nanorod extinction in solution was compared directly to that when immobilized on hydrated paper, and were shown to be functionally equivalent (Fig. 2b). Irrespective of shape, the similarity between spectra originating from nanoparticles in solution and immobilized on hydrated paper (Fig. S4†). In the case of dry plasmonic paper, a substantial blue-shift in resonance is observed, and is the result of change in the refractive index surrounding the nanoparticles (Fig. S5†).
The experiments described previously employed “as-synthesized” nanoparticle solutions in their native surfactants. In order to probe the dependence of the paper substrate adsorption process on nanoparticle surface charge, a variety of ligand chemistries were employed. To demonstrate the modularity of this approach, solutions of nanorods having different surface charges were adsorbed to the paper surface. As demonstrated by their extinction spectra while on the paper, surface charge does not preclude adsorption (Fig. 2c and S6†). This finding effectively nullifies a hypothesis that adsorption of particles to the cellulose fibers is governed predominantly by electrostatic association. To further investigate the adsorption process, we sought to determine whether hydrogen bonding was driving the association between the paper and nanoparticles. To this end, gold nanorods capped with a hydrophobic polystyrene shell were incubated with the paper substrate. Importantly, because these particles were dissolved in toluene, uptake attributed to hydrogen bonding should be minimized. Since the adsorption process was seemingly unaffected (Fig. S7†), we hypothesize that van der Waals interactions govern the adsorption. Corroborating this proposed mechanism are results indicating that passivation of the paper surface with typical blocking agents like poly-L-lysine and bovine serum albumin can reduce uptake (Fig. S8†). Observations that adsorption occurs over longer timescales than the initial wetting of the paper, and that mechanically stirring the solution facilitates additional adsorption further support this hypothesis. Taken together, the characterization and interrogation of the adsorption process demonstrate that nanoparticles adsorb to paper regardless of particle size, shape, and surface chemistry.
Nanoparticles' tendency to aggregate under a variety of chemical conditions is well known. If these structures are to ever be employed in biodiagnostics, they must remain stable in harsh and complex chemical environments (e.g. sweat, blood, urine, etc.). A solution of nanorods was exposed to a number of chemical conditions and compared to immobilized nanorods. Specifically, aliquots of nanoparticles were subjected to freezing, heating, organic solvent, salt, strong acid, or strong base (Fig. 3a). As expected, the solution of nanoparticles was prone to aggregation following an overnight exposure, as demonstrated by a decrease in the extinction. Importantly, the optical properties of nanoparticles in solution are only accessible when the particles remain soluble. Conversely, the paper scaffold acts to effectively “lock-in” the nanoparticle and imparts additional stability. For example, immobilized nanoparticles retain their properties when exposed to 1 M salt (Fig. 3b), whereas free nanoparticles completely aggregate. The additional stability afforded by the paper substrate demonstrates that plasmonic paper could be employed in assays involving biological fluids like sweat. With the exception of heating at 80 °C for 12 hours, all of the peaks are superimposable. Importantly, the blue-shift in the nanorod plasmon resonance when heated is observed both on the substrate and in solution, and is likely the result a decrease in aspect ratio. This effect would likely be negligible in applications where isotropic particles could be employed.
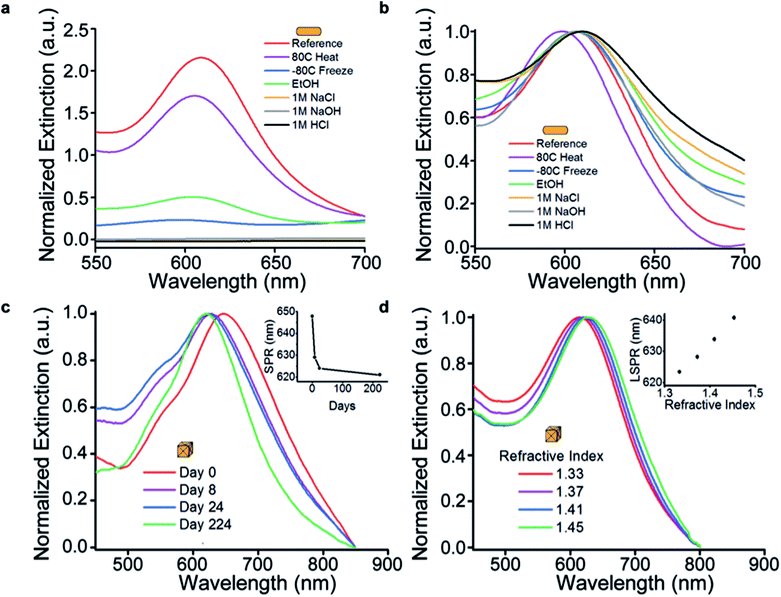 |
| Fig. 3 (a) Extinction spectra of unmodified, and as synthesized gold nanorods when suspended in solutions of a variety of chemical environments, and when exposed to extreme temperature for a period of 12 hours. (b) Equivalent normalized extinction spectra of plasmonic paper after exposure to identical chemical and temperature conditions. (c) Normalized extinction spectra of plasmonic paper as a function of time. SPR peak position over a 224 day experimental window (inset). (d) Normalized extinction spectra of plasmonic paper SPR peak position with respect to refractive index of the surrounding medium of gold concave cubes. Refractive index sensitivity for the concave cube particles while on the paper substrate (inset). | |
To investigate the shelf-life of plasmonic paper, concave cube structures with high-index facets4 were employed because their sharp tips are susceptible to edge rounding and result in substantial changes to their extinction spectrum. While some shift in the SPR peak position was observed, the peak began to stabilize only days after synthesis, and remained relatively stable during the 224 day experimental window (Fig. 3c). Should plasmonic paper be employed as a substrate for LSPR or SERS-based investigations, it may be desirable to have particles of a particular resonance. The predictable change in LSPR can be addressed during experiment design to ensure target peak position over the course of an extended shelf life. For example, slightly larger particles could be synthesized, resulting in red-shifted resonances. As the particles age, edge round, and blue-shift, they will fall into a desired window.
Because particle stability is no longer governed by the presence of surfactant in the solution, the solvent can be manipulated as needed for a particular application. For example, the refractive index sensitivity (RIS) of nanoparticles can be measured simply by wetting the substrates with sucrose solutions of known refractive index (Fig. 3d). In a typical experiment, the extinction of a swatch of paper containing gold concave nanocubes was measured, and subsequently divided for comparative characterization. The substrates were then measured after exposure to aqueous sucrose solutions ranging from 0% to 65% (m/v) to change the local refractive index surrounding the particle from 1.33 (H2O) to 1.45 (65% sucrose). Using this approach, the RIS of the gold concave nanocubes was determined to be 145 nm per RIU. Importantly, the often demonstrated linear relationship between refractive index and LSPR peak position is also observed in the case of plasmonic paper. While higher sensitivities for other particle geometries have been reported,45–47 this demonstration serves as a proof-of-concept for the viability of this approach to directly measuring RIS for various nanoparticle shapes.
Traditionally, chemical manipulation of nanostructures is done in an iterative approach. For example, creating a 2-parameter reaction matrix can elucidate the effects reagent concentration on output geometry and enable refinement of synthetic parameters to produce high-yields of desired shapes. However, the ability to perform experiments using a combinatorial chemistry approach on a single substrate could simplify the screening process during chemical modification. We hypothesized that capillary action provided by the paper substrate could facilitate solvent flow and also be exploited to create spatial concentration gradients of reagents for chemical reactions. Similarly, an opposing gradient would exist and be related to the extent of the overall reaction. The extent of the reactions could therefore be monitored spectroscopically based on physical location. Demonstration of this effect in two dimensions implies that combinatorial chemistry using the paper substrate is possible. To test this hypothesis, two distinct chemical reactions were performed on the same starting material. An experimental approach was selected where obvious colorimetric and morphological differences exist between starting material and product. Specifically, nanocubes consisting of a spherical gold core, surrounded by a cubic silver shell (Au@Ag) were synthesized6 (Fig. S9†). Au@Ag nanocubes were written onto the filter paper substrate using a ball-point pen (Fig. 4a).39,40,48 The line composed of silver nanoparticles was indicated by its yellow color. Given that H2O2 is a known wet etchant for bulk silver, we predicted that it could be employed to selectively etch the silver on the surface of the nanoparticle. The resultant etched particle would be comprised primarily of the gold spherical core, and thus have a very distinct optical signature. While it would be desirable to characterize analogous products synthesized from solution, colloidal instability precludes a comparable experiment in the presence of H2O2. In principle, this demonstrates that the paper substrate can facilitate chemical reactions on nanoparticles that are not accessible while in solution. This could potentially lead to new nanoparticles morphologies not available in the parameter space of solution phase syntheses. On the opposite end of the substrate HAuCl4 could concomitantly be utilized to transform the Au@Ag particles into gold nanorattles using a well-established galvanic replacement reaction.6,46,49
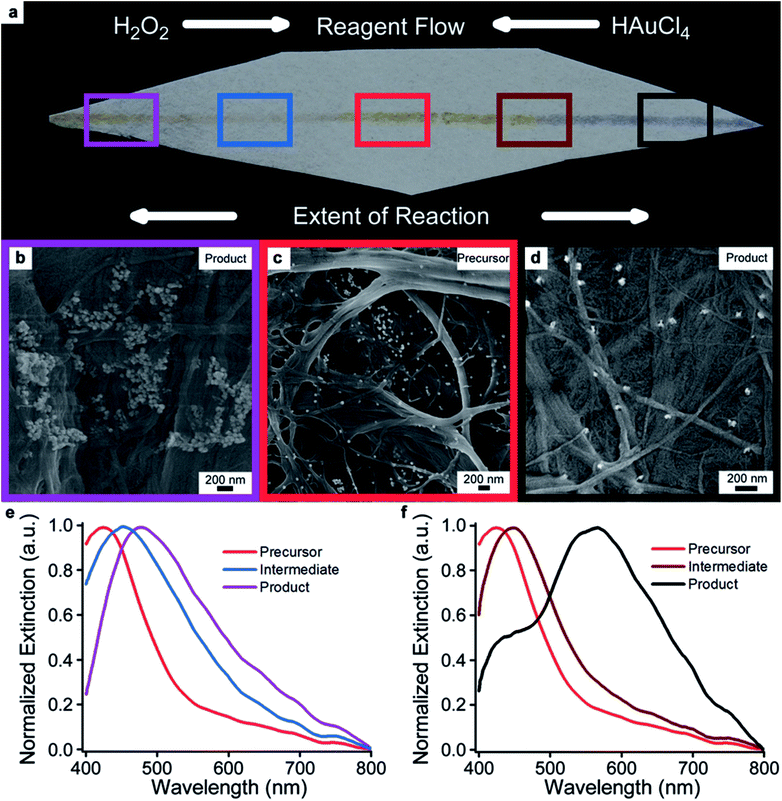 |
| Fig. 4 (a) Photographic image of Au@Ag nanoparticles directly written onto a swatch of filter paper with a ball point pen. (b) SEM image showing the region of nanoparticles etched by an aqueous solution of H2O2. (c) SEM image of the unreacted nanoparticles. (d) SEM image showing the formation of gold nanorattles from the precursor particles by galvanic exchange in the presence of an aqueous solution of HAuCl4. (e) Normalized extinction spectra showing the extent of etching along the line of nanoparticles in the presence of H2O2. (f) Normalized extinction spectra showing the extent of galvanic exchange reaction progress along the same line, but on opposite end of the substrate. Regions of spectroscopic interrogation and corresponding SEM have been color coded for clarity. | |
Briefly, solutions of H2O2 and HAuCl4 were added to distal ends of the paper. In each case, the particles within that region were converted into their respective products, as indicated by color changes. As the solvent fronts moved medially, the extent of conversion decreased as the reagents in solution were consumed. Importantly, the volumes of each solvent were sufficient to flow across the median of the substrate. However, since these reactive components were consumed during the reaction, the middle of the substrate was not converted to either product as indicated by the persistent yellow color of the starting material. SEM micrographs corroborate changes in nanoparticle morphology following the reaction (Fig. 4b–d), however, optical signatures are better indicators of any change in morphology when using the paper substrate. The optical properties of the products formed while on the substrate and in the presence of H2O2 (Fig. 4e) and HAuCl4 (Fig. 4f) were then spectroscopically interrogated and compared to that of the unreacted starting material. While both reactions result in the formation of Au-based products, significant differences in morphology impart vastly different optical signatures. For clarity, the regions for interrogation, corresponding SEM, and respective optical signatures have been color coded for clarity. As expected, the concentration gradient of the particular reactant creates a gradient for the extent of reaction in the opposing direction. To verify this result, multiple spectra along the line have been collected, and their results corroborate a gradient being established for the extent of reaction. The consequence of H2O2 etching results in a well-known red-shift in the resonance wavelength from the UV to the visible, indicating a conversion from mostly silver character to mostly gold character. On the opposite end, the formation of nanorattle products results in the appearance of a new resonance at 566 nm in the extinction spectrum. Importantly, this experiment is a proof-of-concept demonstration of the combinatorial gradients with limited dependence on spatial resolution. Quantitative resolution could be achieved, simply by adding a piezo-controlled stage to the microspectrophotometer.
Conclusions
Taken together, the data presented herein suggest that plasmonic paper is a solid-state analogue to nanoparticle colloids. In principle, this approach is limited only by the scalability of nanoparticle synthesis and paper production. When immobilized on the paper, these particles remain stable under a variety of conditions not amenable to a nanoparticle solution. Because the particles and their associated optical properties are effectively “locked-in” once immobilized on the paper substrate, nanoparticle aggregation during surface modification is no longer an experimental hurdle. This new capability could enable a more direct pathway toward chemical modification and application of nanoparticles. Not only does plasmonic paper preserve nanoparticle function and stability in complex environments, it also facilitates characterization of intrinsic properties. The wettability of the paper substrate uniquely enables a simplistic approach to combinatorial chemistry on nanoparticle surfaces. We assert that the simplicity in fabrication, and versatility in function of plasmonic paper make this a highly promising platform for the development of point-of-need diagnostics. While this work incorporated plasmonic particles, we believe that this approach can be extended to other nanomaterials to be used for applications in catalysis and electronics. In this way, these nanoparticle-infused paper substrates could enable a new research frontier where many previous design limitations of nanoparticle devices, such as undesired particle aggregation or difficulty with particle surface modification, are entirely avoided.
Acknowledgements
This work was partly funded by AFOSR and AFRL/RX and performed while A. L. S. held a National Research Council Research Associateship Award at the Air Force Research Laboratory. The authors would like to thank Dr Ali Jawaid and Dr Rich Vaia for gold nanorod samples modified with thiolated polystyrene ligands for characterization of adsorption from hydrophobic solution.
Notes and references
- B. Nikoobakht and M. A. El-Sayed, Chem. Mater., 2003, 15, 1957–1962 CrossRef CAS.
- A. L. Schmucker, N. Harris, M. J. Banholzer, M. G. Blaber, K. D. Osberg, G. C. Schatz and C. A. Mirkin, ACS Nano, 2010, 4, 5453–5463 CrossRef CAS PubMed.
- K. Sohn, F. Kim, K. C. Pradel, J. S. Wu, Y. Peng, F. M. Zhou and J. X. Huang, ACS Nano, 2009, 3, 2191–2198 CrossRef CAS PubMed.
- J. Zhang, M. R. Langille, M. L. Personick, K. Zhang, S. Li and C. A. Mirkin, J. Am. Chem. Soc., 2010, 132, 14012–14014 CrossRef CAS PubMed.
- Y. Sun, B. T. Mayers and Y. Xia, Nano Lett., 2002, 2, 481–485 CrossRef CAS.
- Y. Yang, J. Liu, Z.-W. Fu and D. Qin, J. Am. Chem. Soc., 2014, 136, 8153–8156 CrossRef CAS PubMed.
- M. R. Jones, K. D. Osberg, R. J. Macfarlane, M. R. Langille and C. A. Mirkin, Chem. Rev., 2011, 111, 3736–3827 CrossRef CAS PubMed.
- J. N. Anker, W. P. Hall, O. Lyandres, N. C. Shah, J. Zhao and R. P. van Duyne, Nat. Mater., 2008, 7, 442–453 CrossRef CAS PubMed.
- S. S. R. Dasary, A. K. Singh, D. Senapati, H. Yu and P. C. Ray, J. Am. Chem. Soc., 2009, 131, 13806–13812 CrossRef CAS PubMed.
- C. H. Lee, M. E. Hankus, L. Tian, P. M. Pellegrino and S. Singamaneni, Anal. Chem., 2011, 83, 8953–8958 CrossRef CAS PubMed.
- S. Guo, Y. Fang, S. Dong and E. Wang, J. Phys. Chem. C, 2007, 111, 17104–17109 CAS.
- N. L. Rosi and C. A. Mirkin, Chem. Rev., 2005, 105, 1547–1562 CrossRef CAS PubMed.
- P. K. Jain, X. Huang, I. H. El-Sayed and M. A. El-Sayad, Plasmonics, 2007, 2, 107–118 CrossRef CAS.
- X. Huang, I. H. El-Sayed, W. Qian and M. A. El-Sayed, J. Am. Chem. Soc., 2006, 128, 2115–2120 CrossRef CAS PubMed.
- P. K. Jain, K. S. Lee, I. H. El-Sayed and M. A. El-Sayed, J. Phys. Chem. B, 2006, 110, 7238–7248 CrossRef CAS PubMed.
- J. N. Anker, W. P. Hall, O. Lyandres, N. C. Shah, J. Zhao and R. P. van Duyne, Nat. Mater., 2008, 7, 442–453 CrossRef CAS PubMed.
- W. P. Hall, J. N. Anker, Y. Lin, J. Modica, M. Mrksich and R. P. van Duyne, J. Am. Chem. Soc., 2008, 130, 5836–5837 CrossRef CAS PubMed.
- W. P. Hall, J. Modica, J. Anker, Y. Lin, M. Mrksich and R. P. van Duyne, Nano Lett., 2011, 11, 1098–1105 CrossRef CAS PubMed.
- P. K. Jain, W. Y. Huang and M. A. El-Sayed, Nano Lett., 2007, 7, 2080–2088 CrossRef CAS.
- C. Sonnichsen, B. M. Reinhard, J. Liphardt and A. P. Alivisatos, Nat. Biotechnol., 2005, 23, 741–745 CrossRef PubMed.
- K. L. Kelly, E. Coronado, L. L. Zhao and G. C. Schatz, J. Phys. Chem. B, 2003, 107, 668–677 CrossRef CAS.
- P. Nordlander, C. Oubre, E. Prodan, K. Li and M. I. Stockman, Nano Lett., 2004, 4, 899–903 CrossRef CAS.
- K. D. Osberg, N. Harris, T. Ozel, J. C. Ku, G. C. Schatz and C. A. Mirkin, Nano Lett., 2014, 14, 6949–6954 CrossRef CAS PubMed.
- C. A. Mirkin, R. L. Letsinger, R. C. Mucic and J. J. Storhoff, Nature, 1996, 382, 607–609 CrossRef CAS PubMed.
- A. P. Alivisatos, K. P. Johnsson, X. Peng, T. E. Wilson, C. J. Loweth, M. P. Bruchez and P. G. Schultz, Nature, 1996, 382, 609–611 CrossRef CAS PubMed.
- I. Pastoriza-Santos, J. Perez-Juste and L. M. Liz-Marzan, Chem. Mater., 2006, 18, 2465–2467 CrossRef CAS.
- J. M. Slocik, J. S. Zabinski Jr, D. M. Phillips and R. R. Naik, Small, 2008, 4, 548–551 CrossRef CAS PubMed.
- R. Lévy, N. T. K. Thanh, R. C. Doty, I. Hussain, R. J. Nichols, D. J. Schiffrin, M. Brust and D. G. Fernig, J. Am. Chem. Soc., 2004, 126, 10076–10084 CrossRef PubMed.
- L. Jia, L. Guo, J. Zhu and Y. Ma, Mater. Sci. Eng., C, 2014, 43, 231–236 CrossRef CAS PubMed.
- A. Abbas, A. Brimer, J. M. Slocik, L. Tian, R. R. Naik and S. Singamaneni, Anal. Chem., 2013, 85, 3977–3983 CrossRef CAS PubMed.
- S. Z. Nergiz, N. Gandra, M. E. Farrell, L. Tian, P. M. Pellegrino and S. Singamaneni, J. Mater. Chem. A, 2013, 1, 6543–6549 CAS.
- L. M. Tian, J. J. Morrissey, R. Kattumenu, N. Gandra, E. D. Kharasch and S. Singamaneni, Anal. Chem., 2012, 84, 9928–9934 CrossRef CAS PubMed.
- C. H. Lee, L. M. Tian and S. Singamaneni, ACS Appl. Mater. Interfaces, 2010, 2, 3429–3435 CAS.
- C. H. Lee, M. E. Hankus, L. M. Tian, P. M. Pellegrino and S. Singamaneni, Proc. SPIE, 2012, 8358, 83515 CrossRef.
- A. W. Martinez, S. T. Phillips and G. M. Whitesides, Proc. Natl. Acad. Sci. U. S. A., 2008, 105, 19606–19611 CrossRef CAS PubMed.
- A. W. Martinez, S. T. Phillips, G. M. Whitesides and E. Carrilho, Anal. Chem., 2010, 82, 3–10 CrossRef CAS PubMed.
- E. Ringe, J. M. McMahon, K. Sohn, C. Cobley, Y. Xia, J. Huang, G. C. Schatz, L. D. Marks and R. P. van Duyne, J. Phys. Chem. C, 2010, 114, 12511–12516 CAS.
- J. M. Bingham, K. A. Willets, N. C. Shah, D. Q. Andrews and R. P. van Duyne, J. Phys. Chem. C, 2009, 113, 16839–16842 CAS.
- L. Tian, S. Tadepalli, M. E. Farrell, K.-K. Liu, N. Gandra, P. M. Pellegrino and S. Singamaneni, J. Mater. Chem. C, 2014, 2, 5438–5446 RSC.
- L. Tian, S. Tadepalli, S. H. Park, K.-K. Liu, J. J. Morrissey, E. D. Kharasch, R. R. Naik and S. Singamaneni, Biosens. Bioelectron., 2014, 59, 208–215 CrossRef CAS PubMed.
- C. J. Orendorff, L. Gearheart, N. R. Jana and C. J. Murphy, Phys. Chem. Chem. Phys., 2006, 8, 165–170 RSC.
- Y. Zheng, X. Zhong, Z. Li and Y. Xia, Part. Part. Syst. Charact., 2014, 31, 266–273 CrossRef CAS.
- R. Gans, Ann. Phys., 1912, 342, 881–900 CrossRef.
- X. H. Huang, S. Neretina and M. A. El-Sayed, Adv. Mater., 2009, 21, 4880–4910 CrossRef CAS PubMed.
- M. J. Banholzer, N. Harris, J. E. Millstone, G. C. Schatz and C. A. Mirkin, J. Phys. Chem. C, 2010, 114, 7521–7526 CAS.
- M. A. Mahmoud, J. Phys. Chem. C, 2014, 118, 10321–10328 CAS.
- H. Chen, X. Kou, Z. Yang, W. Ni and J. Wang, Langmuir, 2008, 24, 5233–5237 CrossRef CAS PubMed.
- L. Polavarapu, A. L. Porta, S. M. Novikov, M. Coronado-Puchau and L. M. Liz-Marzán, Small, 2014, 10, 3065–3071 CrossRef CAS PubMed.
- J. Y. Chen, B. Wiley, Z. Y. Li, D. Campbell, F. Saeki, H. Cang, L. Au, J. Lee, X. D. Li and Y. N. Xia, Adv. Mater., 2005, 17, 2255–2261 CrossRef CAS.
Footnote |
† Electronic supplementary information (ESI) available: Experimental methods, additional spectroscopic characterization and TEM images. See DOI: 10.1039/c5ra21977a |
|
This journal is © The Royal Society of Chemistry 2016 |
Click here to see how this site uses Cookies. View our privacy policy here.