DOI:
10.1039/C5RA25066H
(Paper)
RSC Adv., 2016,
6, 8127-8133
Photocatalysis of NaYF4:Yb,Er/CdSe composites under 1560 nm laser excitation
Received
25th November 2015
, Accepted 9th January 2016
First published on 13th January 2016
Abstract
The most common materials used to generate near-infrared-driven photocatalysis occur by 980 nm laser excitation of composites that are a combination of a semiconductor and upconverting luminescence particles. The challenge remains to increase the light harvesting efficiency, and thus, it is necessary to extend the absorption spectra of photocatalysts. In this work, NaYF4:Yb,Er/CdSe composites were prepared by depositing CdSe nanocrystals onto the surface of NaYF4:Yb,Er microcrystals. UV and visible emission of light resulted from multiphoton upconverting processes in Er3+ under 1560 nm laser irradiation, which, in turn, activated the CdSe catalyst. The energy transfer between NaYF4:Yb,Er and CdSe was investigated by steady-state and dynamic fluorescence spectroscopy. The photocatalytic performance was investigated by the degradation of methylene blue in aqueous solution. These results show that Er coupled with semiconductor heterojunctions provides a photocatalyst that operates in the extended near-infrared range.
1. Introduction
Near-infrared (NIR) photocatalysts, using direct NIR-to-visible radiation or generating UV radiation through upconversion to provide the necessary energy to a semiconductor, are a new research area in the field of photocatalysis and photoelectrochemical water splitting.1–5 The application of an upconversion process using phosphor-like systems would lead to an increase in the photocatalytic performance of traditional UV or visible active photocatalysts. This occurs because the light absorption range is effectively extended to the infrared range, which accounts for nearly half of the solar energy output. In our previous work, we reported the NIR-driven photocatalysis of broadband and heterojunction composites of semiconductors combined with upconverting luminescence particles.6–9 Using this strategy, the direct absorption of NIR light by the semiconductor (Eg > 2.0 eV) does not occur, but when combined with upconverting materials, the same semiconductors showed photocatalytic properties under illumination of NIR light sources. From our initial work,6 other materials such as NaYF4:Yb,Tm/CdS,10 Er:YAlO3/ZnO,11 Er-doped BiVO4,12 CaF2:Yb,Er/TiO2
13 and NaYF4:Yb,Tm@TiO2,14 have been generated and shown to operate with NIR irradiation. However, all these studies have used 980 nm laser excitation. Therefore, from the viewpoint of harvesting solar energy, it is desirable to extend the light absorption spectra of the photocatalysts to operate over a broader range of the NIR solar spectrum.
The main hurdle limiting widespread usage of these materials is their low photocatalytic efficiency under NIR illumination. This means that the contribution of the upconverted photons to the overall efficiency compared to UV and visible photocatalysts would be small, on the order of 1–3%. However, NIR upconversion materials have potential application in the diagnosis and photodynamic therapy of cancers15–18 because of the much higher penetration depth of NIR into tissue compared to both UV and visible radiation. For example, inorganic upconversion nanoparticles have been extensively used for in vivo detection and visualization of diverse animal cells and bio-tissues. This field has been expanding rapidly and is discussed in several excellent reviews published in recent years.19,20 In particular, Xu et al. reported that 1600–1800 nm radiation have additional advantages for in vivo measurements.21 In this spectral window, light scattering by the tissue is small relative to both UV and visible light, which increases the penetration depth as well as improves the focusing capability of the NIR light. As a result, development of materials in exhibit upconversion at 1560 nm could be disable in the imaging or therapy of brain cell and blood vessels residing in a > 150 μm thick brain tissue section.22
In this paper, NIR photocatalysts of NaYF4:Yb,Er/CdSe composites were synthesized using a two-step hydrothermal method. CdSe nanocrystals were linked onto the surfaces of the NaYF4:Yb,Er microcrystals. The energy transfer between NaYF4:Yb,Er and CdSe was investigated by steady-state and dynamic fluorescence spectroscopy. The photocatalytic activities of the composites were evaluated by the degradation of methylene blue (MB) aqueous solutions under 1560 nm NIR irradiation. Designing materials such as those reported here that can operate over a wider range of NIR wavelengths will help in the design of photovoltaic devices that cover a broader region of the solar spectrum.
2. Experimental
2.1 Materials
Yttrium oxide (Y2O3), ytterbium oxides (Yb2O3) and erbium oxide (Er2O3) were obtained from the Shandong Yutai Fine Chemical Factory at 99.999% purity. Sodium fluoride (NaF) and ethylenediamine tetraacetic acid (EDTA) were obtained from the Beijing Chemical Reagent Factory. Cadmium chloride (CdCl2), selenium powder, sodium borohydride (NaBH4) and thiolglycolic acid (TGA) were purchased from the Tianjin Guangfu Fine Chemical Research Institute and used without further purification.
2.2 Synthesis of NaYF4:Yb,Er microcrystals
NaYF4:Yb,Er microcrystals were synthesized via the ethylene tetraacetic acid (EDTA) assisted hydrothermal method, described elsewhere.23 Briefly, 10 mL of 0.5 mmol Y(NO3)3, Yb(NO3)3 and Er(NO3)3 (Y
:
Yb
:
Er = 79
:
20
:
1) were added to 20 mL of 0.25 mmol EDTA in deionized water. The solution was stirred for 1 h to allow for the formation of a chelated RE–EDTA complex. Next, 20 mL of 0.5 M NaF solution was added to the solution to generate a RE3+
:
F− ratio of 1
:
16. After stirring for 0.5 h, the mixture was transferred to a 50 mL Teflon-lined stainless steel autoclave. The autoclave was sealed and placed in an oven at 160 °C for 18 h, and then cooled to room temperature in air. The white colored product was centrifuged and washed with deionized water and ethanol three times, followed by drying in air drying at 60 °C for 20 h. Finally, the sample was annealed at 400 °C for 1.5 h under an argon atmosphere.
2.3 Synthesis of CdSe nanoparticles
The preparation procedures of the CdSe nanoparticles are described elsewhere.24 Briefly, 0.47 g CdCl2·5H2O was dissolved in 100 mL deionized water, in a 250 mL three-neck flask under stirring and 0.5 mL TGA was then added dropwise. The pH of the solution was adjusted to 10.3, using 1.0 mol L−1 NaOH. Next, the solution was bubbled with N2 for 30 min. Then, 1.0 mL of 1 M oxygen-free NaHSe was injected, using a syringe into the vigorously stirred solution. The turning of the solution color from white to orange indicated the production of CdSe nanoparticles. The product was centrifuged at 8500 rpm and washed with deionized water and ethanol 3 times. The product was finally dried under vacuum at 60 °C for 10 h.
2.4 The preparation of NaYF4:Yb,Er/CdSe composites
NaYF4:Yb,Er/CdSe composites were fabricated using a method described elsewhere.10 Typically, 500 mg of NaYF4:Yb,Er microcrystals were dispersed in 20 mL deionized water containing 0.2 mL mercaptoethanol. After stirring for 3 h, the product was separated and washed. In a separate beaker, 100 mg of CdSe nanoparticles were dispersed in 10 mL deionized water containing 0.1 mL thioglycolic acid. The solution was stirred for 3 h, and then centrifuged and washed.
The NaYF4:Yb,Er microcrystals and CdSe nanoparticles were mixed in 20 mL deionized water, and heated at 160 °C for 3 h. The products were collected by centrifugation and washed with deionized water several times.
2.5 Characterizations
The crystal structures of the NaYF4:Yb,Er/CdSe composite were analyzed by a Rigaku RU-200b X-ray powder diffraction (XRD), using a nickel-filtered Cu Kα radiation (λ = 1.5418 Å). The size and morphology were characterized by SEM (JEOL-7500F with operating voltage of 15 kV) and TEM (JEM2000-EX, 100 kV). Energy dispersive X-ray (EDX) scan profiles were obtained on a JSM-7500F microscope coupled with an EDX spectrometer (Genesis Apollo XL). The absorption spectra were recorded with a UV-vis spectrophotometer (Shimadzu UV-3600). The emission spectra were recorded with a spectrophotometer (Hitachi F-4500) equipped with a Hamamatsu R928 photomultiplier and a 1560 nm continuous wave diode laser. The luminescence decays was studied by using a 1579 nm Raman shifter laser (pumped by the second harmonic of a Nd:YAG pulsed laser, pulse width 10 ns, repetition rate 10 Hz). All above measurements were performed at room temperature.
2.6 The evaluation of the photocatalytical activity
The photocatalytical activity of the NaYF4:Yb,Er/CdSe composite material was evaluated using the degradation of MB in aqueous solution. In a typical experiment, 0.5 mg of NaYF4:Yb,Er/CdSe composite particles was dispersed into a quartz vial containing 0.5 mL 15 mg L−1 MB aqueous solution. Prior to irradiation, the vial was kept in the dark for 12 h for establishing adsorption–desorption equilibrium of MB on the surface of the photocatalysts. A diode laser at 1560 nm, with a power density of 2.0 W cm−2, was used as the NIR source. After each 5 h irradiation, 0.3 mL aliquot of MB aqueous solution was extracted for UV-vis absorbance measurement. The sample was returned to the quartz vial after recording the UV spectrum. The dye concentration was measured with a UV-vis spectrometer, using the absorption peak at 665 nm. For comparison, the NIR-responsive photo degradation of MB, using CdSe and NaYF4:Yb,Er, were also measured by the same procedures above, respectively.
3. Results and discussion
3.1 Structure characterizations
The crystal structure of CdSe nanocrystals, and NaYF4:Yb,Er/CdSe composites were determined by XRD. As shown in Fig. 1(a), the XRD patterns of the CdSe nanoparticles were in a good agreement with the hexagonal phase CdSe (JCPDS no. 77-2307). Fig. 1(b) shows an XRD pattern of the NaYF4:Yb,Er/CdSe composite. The pattern is dominated by peaks associated with the hexagonal phase of NaYF4 (JCPDS no. 28-1192), while weak diffraction peaks of CdSe are observed at 25°.
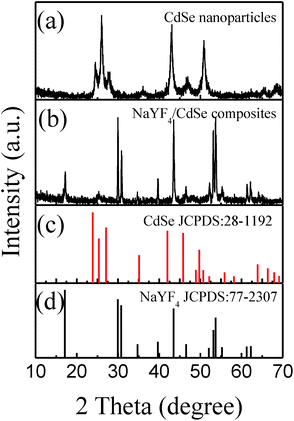 |
| Fig. 1 The XRD patterns of (a) the CdSe nanoparticles, (b) the NaYF4:Yb,Er/CdSe composites, (c) JCPDS no. 28-1192, (d) JCPDS no. 77-2307. | |
The morphologies of NaYF4:Yb,Er microcrystals and NaYF4:Yb,Er/CdSe composites were characterized by SEM and TEM. Fig. 2(a) shows that the NaYF4:Yb,Er microcrystals are hexagonal pillars with a uniform dimension of ∼2 μm in length and ∼1 μm in diameter. Fig. 2(b) show the SEM images of the NaYF4:Yb,Er/CdSe composites. EDX was employed to further analyze the elemental composition of the NaYF4:Yb,Er/CdSe composites (Fig. 2(c)), which suggests that the composites consist of Yb, Er, Na, Y, F, Cd and Se elements. Fig. 2(d) and (e) are the TEM images of NaYF4:Yb,Er/CdSe composites at various magnifications. The TEM image in Fig. 2(e) shows that on the surface of the NaYF4:Yb,Er/CdSe composites is a 50 nm-thickness layer of tightly interconnected nanoparticles with a size of 6–8 nm. Moreover, high-resolution transmission electron micrographs (HR-TEM) (Fig. 2(f)) show that these nanoparticles are composed of CdSe nanoparticles. In particular, a lattice of 0.35 nm corresponded to the interplanar distance of the (001) lattice plane of the hexagonal CdSe phase.
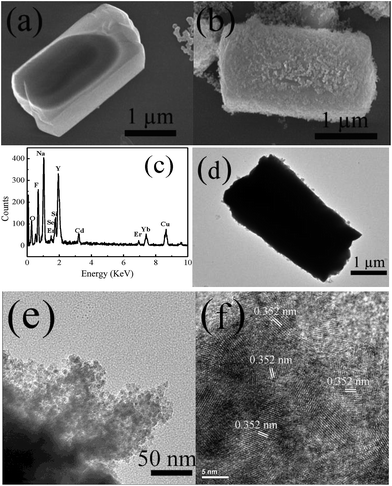 |
| Fig. 2 SEM images of (a) NaYF4:Yb,Er microcrystals and (b) NaYF4:Yb,Er/CdSe composites; (c), EDX pattern of sample shown in (d) and (e) which are the TEM images of NaYF4:Yb,Er/CdSe composites at various magnifications; (f) HRTEM image of CdSe nanoparticles on the NaYF4:Yb,Er surface. | |
3.2 Photoluminescence properties
Fig. 3 shows the upconverted emission spectra of NaYF4:Yb,Er microcrystals and NaYF4:Yb,Er/CdSe composites under 1560 nm excitation. In the spectrum of NaYF4:Yb,Er microcrystals, five emission peaks located at 379 nm (3.272 eV), 408 nm (3.039 eV), 520 nm (2.385 eV), 539 nm (2.301 eV) and 664 nm (1.867 eV) correspond to 4G11/2 → 4I15/2, 2H9/2 → 4I15/2, 2H11/2 → 4I15/2, 4S3/2 → 4I15/2 and 4F9/2 → 4I15/2 transitions of Er3+ ions, respectively.25 For comparison, the two spectra in Fig. 3 were normalized using the red emission of Er3+ ions at 664 nm (1.867 eV), as the excited state energy of 664 nm is lower than that of the CdSe nanocrystals acceptor and thus, should not be absorbed by CdSe nanocrystals.26,27 It was found that the emission spectrum of NaYF4:Yb,Er/CdSe composites is different from that of NaYF4:Yb,Er microcrystals. The intensities of the emission peaks at 379 nm, 408 nm, 520 nm and 539 nm for NaYF4:Yb,Er decrease after the incorporation of CdSe nanocrystals, compared to the red emission of Er3+ at 660 nm which did not change, as the energy of this excited state is lower than that of the CdSe acceptor.27 Specifically, for NaYF4:Yb,Er, the decrease in fluorescence intensity ratio were calculated using the equation, Idecay = 1 − Icoated/Iuncoated, where Iuncoated and Icoated are the intensity of the emission peak in NaYF4:Yb,Er without and with CdSe, respectively. The intensity decay values of Idecay for the 408, 520 and 539 nm peaks are calculated to be 0.75, 0.80 and 0.45, respectively. This decrease in fluorescence shows the presence of an energy transfer from NaYF4:Yb,Er to CdSe.
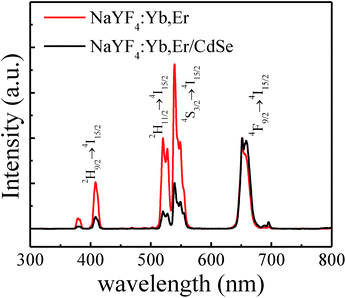 |
| Fig. 3 Photoluminescence spectra of NaYF4:Yb,Er microcrystals (red) and NaYF4:Yb,Er/CdSe composites (black) under 1560 nm excitation at room temperature. | |
3.3 UV-vis spectra
UV-vis absorption spectra of NaYF4:Yb,Er/CdSe composites and CdSe nanoparticles are shown in Fig. 4. The band-gap absorption peaks at ∼620 nm (2.0 eV) are characteristic of the CdSe nanoparticles.28 the peak at 976 nm is associated with 2F5/2 → 2F7/2 transition of Yb3+.29 As the energies of the excited states of Er3+ ions at 4G11/2 (3.272 eV), 2H9/2 (2.981 eV), 2H11/2 (2.322 eV) and 4S3/2 (2.246 eV) are larger than that of the CdSe bandgap, the excited energy of Er3+ exceeds with the bandgap of CdSe, and thus, can be absorbed by CdSe via energy transfer.
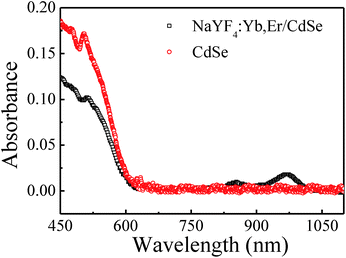 |
| Fig. 4 UV-vis spectrum of CdSe and NaYF4:Yb,Er/CdSe composites. | |
3.4 Energy transfer
To demonstrate the energy transfer process between NaYF4:Yb,Er and CdSe, the time-resolved fluorescence decay of the 2H9/2, 2H11/2, 4S3/2 and 4F9/2 levels of Er3+ was measured and shown in Fig. 5. Accelerated decay rates were found for the 2H9/2, 2H11/2, 4S3/2 excited states in NaYF4:Yb,Er/CdSe composite (see Fig. 5(a)–(c)), while identical decay rates were found for the 4F9/2 of Er3+ between NaYF4:Yb,Er/CdSe composite and pure NaYF4:Yb,Er (see Fig. 5(d)). This is consistent with the results from the emission spectra (Fig. 3). That a significant decrease in the emission intensity of 2H9/2, 2H11/2, 4S3/2 was found in NaYF4:Yb,Er/CdSe composites after the incorporation of CdSe. These results also suggest the presence of the Förster resonance energy transfer (FRET) between NaYF4:Yb,Er and CdSe.26 The efficiency of energy transfer was calculated using the equation Edecay = 1 − τcoated/τuncoated, where τcoated and τuncoated are the lifetime of the excited states in NaYF4:Yb,Er with and without CdSe, respectively.30 The lifetime of excited state of luminescence centers, τ, depends on the radiative transition rate (WR), the nonradiative transition rate (WNR) and the energy transfer rate, which can be expressed as τ = 1/(WR + WNR + WET).31,32 As shown in Table 1, the calculated energy transfer efficiencies for 2H9/2, 2H15/2, 4S3/2 and 4F9/2 levels were 0.36, 0.35, 0.20, and 0, respectively. These values are much lower than the Idecay, which were calculated to be 0.75, 0.80 and 0.45. This suggests that two mechanisms of energy transfer from the Er3+ ions to CdSe are present. One process is FRET. In this case, the presence of CdSe in contact with the NaYF4:Yb,Er microcrystals creates a nonradiative energy transfer channel from the excited state of Er3+ to CdSe. Such an energy transfer process lead to the acceleration of the relaxation of the excited state of Er3+, leading to an increase in the overall transition rate and thus, the reduction of fluorescence lifetime. This provides evidence that FRET takes place.26 The other process corresponds to a photon reabsorption (PR) process, where the emitted photons from Er3+ are adsorbed by the semiconductors.
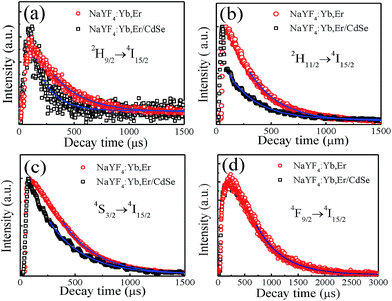 |
| Fig. 5 Luminescence dynamic curves form the 2H9/2 (a), 2H11/2 (b), 4S3/2 (c) and 4F9/2 (d) of Er3+ ions in NaYF4:Yb,Er microcrystals and NaYF4:Yb,Er/CdSe composites under the excitation of a 1579 nm pulsed Raman shift laser. | |
Table 1 Lifetimes of 2H9/2, 2H11/2, 4S3/2 and 4F9/2 levels of Er3+ in NaYF4:Yb,Er microcrystals and NaYF4:Yb, Er/CdSe composites from variations in Fig. 5
Samples |
Lifetime (μs) |
Energy level |
2H9/2 |
2H15/2 |
4S3/2 |
4F9/2 |
NaYF4:Yb,Er |
324 |
304 |
405 |
495 |
NaYF4:Yb,Er/CdSe |
210 |
196 |
323 |
495 |
Edecay |
0.36 |
0.3 |
0.20 |
0 |
Idecay |
0.75 |
0.8 |
0.45 |
0 |
3.5 Photocatalytic activity
MB was used as a model pollutant to investigate the photocatalytic activity of NaYF4:Yb,Er/CdSe under NIR irradiation of 1560 nm. Fig. 6(a) shows the absorbance spectra of MB catalysed by the NaYF4:Yb,Er/CdSe composites as a function of irradiation time under 1560 nm irradiation. The intensity of the band at 650 nm, due to MB, decreased with an increase in the NIR irradiation time. A 65% degradation of MB was found for NaYF4:Yb,Er/CdSe composites after 30 h irradiation.
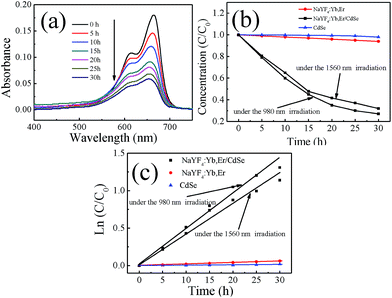 |
| Fig. 6 (a) Absorbance spectra of MB catalyzed by NaYF4:Yb,Er/CdSe composites as a function of the irradiation time under 1560 nm laser; (b) normalized concentration of MB decomposed by CdSe, NaYF4:Yb,Er and NaYF4:Yb,Er/CdSe (1560 nm and 980 nm irradiation). (c) The kinetic data for the photodegradation of MB. | |
The degradation efficiency can be evaluated through the time dependent change of the concentration of MB during the NIR irradiation relative to the original MB. Fig. 6(b) shows the time dependent value of C/C0, where C0 is the original concentration of MB and C is the concentration of MB irradiated with a 1560 nm laser for time (t).
It is well known that NIR irradiation and nonradiative relaxation of excited Yb3+ and Er3+ ions can generate thermal energy. To verify whether the thermal energy dominated the degradation of MB, control experiments of MB in the presence of NaYF4:Yb,Er with NIR radiation were performed. As shown in Fig. 6(b), these solutions showed very limited activities (2%) in the degradation of MB after 30 h irradiation.
This suggests that the thermal energy generated by a 1560 nm laser irradiation is not sufficient to induce the thermal degradation of MB. As a result, the degradation of MB by upconverting/semiconductor composite catalysts induced predominately by photocatalysis rather than photolysis or thermolysis. The degradation of MB under 980 nm radiation was also performed. There was showed 75% activity in the degradation of MB after 30 h irradiation.
The reaction kinetics for the MB photodegradation were fitted using a Langmuir–Hinshelwood kinetic model. The rate constants were calculated to be 3.5 × 10−4 (min−1) and 8.0 × 10−4 (min−1) for NaYF4:Yb,Er/CdSe under the 1560 nm and 980 nm laser irradiation, respectively. Compared to our previous research result,9 the rate constants of NaYF4:Yb,Tm/CdS were calculated to be 3.38 × 10−4 (min−1) under the 980 nm laser irradiation.
There are two factors that can used to explain the reason for the rate constants. First, the population of the states 1I6, 1D2, and 1G4 of Tm3+ are due to five-photon, four-photon, and three-photon UC processes under 980 nm laser irradiation, respectively.33 In contrast, the population of the states 2H9/2, 2H15/2 and 4S3/2 of Er3+ are all due to four-photon, UC processes under 1560 nm laser irradiation.34 However, two photons are needed to excite Yb3+ ions to the 2F5/2 state from their ground state 2F7/2 under 1560 nm excitation, while only one photon is required under 980 nm excitation. As a result, the UV or visible UC emissions under 1560 excitation are of lower intensity, compared to those under 980 nm excitation, because they belong to a higher-order photon process.34 The second factor is that the emission intensity increases with an increase of activator concentration.35 In our case, the content of Er3+ (1%) is twice than that of Tm3+ (0.5%) in NaYF4 used in our earlier work using 980 nm illumination. Therefore, the higher rates due to the higher concentration of Er is offset by the lower rates due to the longer 1560 nm laser irradiation.
3.6 Mechanism
Fig. 7 describes the overall photocatalysis processes of NaYF4:Yb,Er/CdSe composite catalysts under 1560 nm excitation, including the formation of excited levels of Er3+, the activation of semiconductors via energy transfer, the charge migration between semiconductors, and the generation of free radicals. There are three steps. First, Er3+ has a large absorption around 1560 nm excitation, which matches well with their 4I15/2 → 4I13/2 transition. Upon 1560 nm excitation, higher energy levels of Er3+ ions (2H9/2, 2H11/2, 4S3/2 and 4F9/2) are populated, including ground-state absorption (GSA), excited-state absorption (ESA), and energy transfer (ET).34 Second, the energy of these excited levels exceeds the energy gap of CdSe. According to the SEM and TEM images, NaYF4:Yb,Er and CdSe are in intimate contact. This short-range interaction allows FRET to occur from NaYF4:Yb,Er to CdSe.26 In addition to FRET, there can also be photon reabsorption (PR). Lastly, the activated CdSe produces electrons and holes in the conduction band (CB) and the valence band (VB), respectively. These electron–hole pairs migrate from the inner region to the surfaces to take part in surface reactions. As shown in Fig. 7, the excited electrons arriving on the surfaces react with the oxygen adsorbed on the surfaces of CdSe to form O2− or O22−, which combines with H+ to form hydrogen peroxide (H2O2).36 H2O2 can react with the superoxide radical anion (O2·−), reducing it to hydroxyl radicals (·OH),36 whereas, the photogenerated holes can react with H2O to form hydroxyl radicals (·OH).36,37 The ·OH species is a strong oxidant for the partial or complete mineralization of organic chemicals.
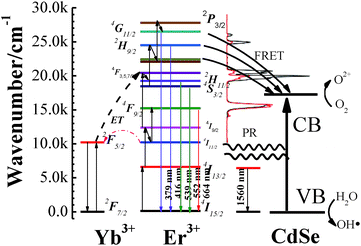 |
| Fig. 7 Energy transfer upconversion scheme of NIR 1560 nm radiation to populate 2H9/2, 2H15/2, 4S3/2 and 4F9/2 levels of Er3+ ions in NaYF4:Yb,Er microcrystals. The emitting levels are populated, either by nonradiative de-excitation (gray wave arrows) or cross-relaxations. The UCNPs energy is transferred to CdSe, either through FRET or photon reabsorption (PR). The corresponding conduction (CB) and valence bands (VB) of CdSe are presented. | |
4. Conclusions
In summary, NaYF4:Yb,Er/CdSe, near-infrared photocatalysts, were prepared by subsequent deposition of CdSe nanoparticles on the surface of NaYF4:Yb,Er upconversion microcrystals. XRD, SEM and TEM analysis show that the CdSe nanoparticles are in contact with the surface of upconversion microcrystals to form a heterojunction structure. UCPL spectra and time resolved spectra were used to characterize the energy transfer between NaYF4:Yb,Er and CdSe. The intensity decay values of Idecay of 408 nm (2H9/2 → 4I15/2), 520 nm (2H15/2 → 4I15/2) and 539 nm (4S3/2 → 4I15/2) of Er3+ are calculated to be 0.75, 0.80 and 0.45, respectively. However, the calculated energy transfer efficiencies for the 2H9/2, 2H15/2, and 4S3/2 levels were 0.36, 0.35, and 0.20, respectively. These values show that the energy transfer processes include FRET and PR between NaYF4:Yb,Er and CdSe. The decomposition of MB was carried out by the photocatalytic composites under 1560 nm laser irradiation. It was found that the rate constant was calculated to be 3.5 × 10−4 (min−1) for NaYF4:Yb,Er/CdSe. This rate constant is similar in value to the rate constants of NaYF4:Yb,Tm/CdS under 980 nm laser irradiations,9 the Tm concentration was twice that of Er which suggest that an increase in the content of Er3+ should increase catalytic efficiency under the 1560 nm laser irradiation. This study demonstrates the potential improvements in the utility rate of solar energy for photochemical and photoelectrical applications based on upconversion materials.
Acknowledgements
The authors gratefully acknowledge financial support from China Scholarship Council. This work has been financially supported by the National Natural Science Foundation of China (NSFC 11274139, 61275189, 51302103, 11474132, 11404136).
Notes and references
- D.-X. Xu, Z.-W. Lian, M.-L. Fu, B. Yuan, J.-W. Shi and H.-J. Cui, Appl. Catal., B, 2013, 142, 377–386 CrossRef
. - F. Fresno, R. Portela, S. Suarez and J. M. Coronado, J. Mater. Chem. A, 2014, 2, 2863–2884 CAS
. - W. Q. Fan, H. Y. Bai and W. D. Shi, CrystEngComm, 2014, 16, 3059–3067 RSC
. - Z. Wang, Y. Liu, B. Huang, Y. Dai, Z. Lou, G. Wang, X. Zhang and X. Qin, Phys. Chem. Chem. Phys., 2014, 16, 2758–2774 RSC
. - C. K. Chen, H. M. Chen, C.-J. Chen and R.-S. Liu, Chem. Commun., 2013, 49, 7917–7919 RSC
. - W. P. Qin, D. S. Zhang, D. Zhao, L. L. Wang and K. Z. Zheng, Chem. Commun., 2010, 46, 2304–2306 RSC
. - Y. N. Tang, W. H. Di, X. S. Zhai, R. Y. Yang and W. P. Qin, ACS Catal., 2013, 3, 405–412 CrossRef CAS
. - X. Guo, W. Song, C. Chen, W. Di and W. Qin, Phys. Chem. Chem. Phys., 2013, 15, 14681–14688 RSC
. - X. Guo, W. Di, C. Chen, C. Liu, X. Wang and W. Qin, Dalton Trans., 2014, 43, 1048–1054 RSC
. - C. H. Li, F. Wang, J. A. Zhu and J. C. Yu, Appl. Catal., B, 2010, 100, 433–439 CrossRef CAS
. - J. Wang, J. Li, Y. P. Xie, L. Q. Zhang, G. X. Han, Y. Li, R. Xu and X. D. Zhang, Inorg. Mater., 2010, 46, 399–404 CrossRef CAS
. - S. Obregon, S. W. Lee and G. Colon, Dalton Trans., 2014, 43, 311–316 RSC
. - S. Huang, L. Gu, C. Miao, Z. Lou, N. Zhu, H. Yuan and A. Shan, J. Mater. Chem. A, 2013, 1, 7874–7879 CAS
. - Y. Zhang and Z. Hong, Nanoscale, 2013, 5, 8930–8933 RSC
. - L. Zeng, L. Luo, Y. Pan, S. Luo, G. Lu and A. Wu, Nanoscale, 2015, 7, 8946–8954 RSC
. - H. Li, S. Song, W. Wang and K. Chen, Dalton Trans., 2015, 44, 16081–16090 RSC
. - Q. Q. Dou, A. Rengaramchandran, S. T. Selvan, R. Paulmurugan and Y. Zhang, Sci. Rep., 2015, 5, 8252 CrossRef CAS PubMed
. - X. Liu and J. Qiu, Chem. Soc. Rev., 2015, 44, 8714–8746 RSC
. - Y. I. Park, K. T. Lee, Y. D. Suh and T. Hyeon, Chem. Soc. Rev., 2015, 44, 1302–1317 RSC
. - A. Sedlmeier and H. H. Gorris, Chem. Soc. Rev., 2015, 44, 1526–1560 RSC
. - N. G. Horton, K. Wang, D. Kobat, C. G. Clark, F. W. Wise, C. B. Schaffer and C. Xu, Nat. Photonics, 2013, 7, 205–209 CrossRef CAS PubMed
. - J. Tragardh, G. Robb, K. K. E. Gadalla, S. Cobb, C. Travis, G. L. Oppo and G. McConnell, Opt. Lett., 2015, 40, 3484–3487 CrossRef PubMed
. - G. F. Wang, W. P. Qin, L. L. Wang, G. D. Wei, P. F. Zhu and R. J. Kim, Opt. Express, 2008, 16, 11907–11914 CrossRef CAS PubMed
. - J. Aldana, Y. A. Wang and X. G. Peng, J. Am. Chem. Soc., 2001, 123, 8844–8850 CrossRef CAS PubMed
. - F. Auzel, Chem. Rev., 2004, 104, 139–173 CrossRef CAS PubMed
. - A. Bednarkiewicz, M. Nyk, M. Samoc and W. Strek, J. Phys. Chem. C, 2010, 114, 17535–17541 CAS
. - C. Yan, A. Dadvand, F. Rosei and D. F. Perepichka, J. Am. Chem. Soc., 2010, 132, 8868–8869 CrossRef CAS PubMed
. - Q. Zhang, P. Yang, H. S. Chen, B. B. Huang and J. X. Shen, J. Nanopart. Res., 2014, 16, 2236 CrossRef
. - Q. Shang, H. Yu, X. Kong, H. Wang, X. Wang, Y. Sun, Y. Zhang and Q. Zeng, J. Lumin., 2008, 128, 1211–1216 CrossRef CAS
. - H. Lu, O. Schops, U. Woggon and C. M. Niemeyer, J. Am. Chem. Soc., 2008, 130, 4815–4827 CrossRef CAS PubMed
. - W. H. Di, X. J. Wang, B. J. Chen, S. Z. Lu and X. X. Zhao, J. Phys. Chem. B, 2005, 109, 13154–13158 CrossRef CAS PubMed
. - L. X. Yu, H. W. Song, Z. X. Liu, L. M. Yang and S. Z. L. Z. H. Zheng, J. Phys. Chem. B, 2005, 109, 11450–11455 CrossRef CAS PubMed
. - G. F. Wang, W. P. Qin, J. S. Zhang, J. S. Zhang, Y. Wang, C. Y. Cao, L. L. Wang, G. D. Wei, P. F. Zhu and R. J. Kim, J. Phys. Chem. C, 2008, 112, 12161–12167 CAS
. - K. Z. Zheng, D. Zhao, D. S. Zhang, N. Liu and W. P. Qin, Opt. Lett., 2010, 35, 2442–2444 CrossRef CAS PubMed
. - F. Shi, J. S. Wang, D. S. Zhang, G. S. Qin and W. P. Qin, J. Mater. Chem., 2011, 21, 13413–13421 RSC
. - Q. Kang, Q. Z. Lu, S. H. Liu, L. X. Yang, L. F. Wen, S. L. Luo and Q. Y. Cai, Biomaterials, 2010, 31, 3317–3326 CrossRef CAS PubMed
. - A. Sarkar, A. Shchukarev, A. R. Leino, K. Kordas, J. P. Mikkola, P. O. Petrov, E. S. Tuchina, A. P. Popov, M. E. Darvin, M. C. Meinke, J. Lademann and V. V. Tuchin, Nanotechnology, 2012, 23, 475711 CrossRef PubMed
.
|
This journal is © The Royal Society of Chemistry 2016 |
Click here to see how this site uses Cookies. View our privacy policy here.