DOI:
10.1039/C5RA26357C
(Paper)
RSC Adv., 2016,
6, 9911-9915
Synthesis, structure and properties of an octahedral dinuclear-based Cu12 nanocage of trimesoyltri(L-alanine)†
Received
10th December 2015
, Accepted 13th January 2016
First published on 18th January 2016
Abstract
The reaction of a three-armed ligand of trimesoyltri(L-alanine) (TMTAH3) with CuCl2 gave [Cu12(TMTA)8(H2O)12]·8CH3CH2OH·40H2O (1) as expected. It features paddlewheel dinuclear secondary building blocks, six of which are connected by eight TMTA3− linkers, forming a Mo6C184+-like dinuclear-based octahedral nanocage. The magnetic studies revealed the presence of antiferromagnetic interactions between the Cu(II) ions in 1. The desolvated sample of 1 presents nice selectivity on the adsorption of H2 and CO2 over CH4.
Introduction
Metal–organic nanocages, which are also called metal–organic polyhedrons and coordination cages,1 have attracted great attention of chemists because of their aesthetic topologies2 and potential applications in fields such as gas storage, separation, catalysis, sensing, and drug delivery.3 In comparison to metal–organic frameworks, they show much better solubility in many solvents.3d Thus, a lot of effort has been devoted to designing and constructing metal–organic nanocages under control.4 However, their rational design and syntheses with desired properties are still a great challenge for chemists. Thus we take the challenge to develop the synthetic strategy of nanocages.
As well known, Mo6Cl84+ has an octahedral Mo612+ skeleton (Scheme 1a),5 in which the three Mo atoms in each triangular face are further consolidated by one μ3-Cl− ion. Based on this structure, we have ever contemplated that it might also be extended into metal–organic nanocage compounds with the Mo vertexes replaced by four-connecting polynuclear secondary building blocks6 and the μ3-Cl− ions replaced by three-armed organic linkers as shown in Scheme 1b. Thus the design of appropriate organic linkers is of great importance in building such cages.7 Three factors are to be taken into consideration for this linker. The first one is that the flexibility of the linker should be controlled in a certain range to sustain a stable cage. Usually, the rigid and semi-rigid linkers show much advantage over flexible ones in the construction of such nanocages out of the stability reasons. Another factor is to fuse some linking groups onto the organic linkers to help to form the polynuclear secondary building blocks. As well known, carboxylate groups are often to be a nice selection for its strong linking ability and versatile linking modes.8 The third one is to keep it in mind that the designed organic linkers must consolidate three polynuclear secondary building blocks.
 |
| Scheme 1 (a) A schematic show of Mo6Cl84+; (b) a schematic show of the designed cage based on the structure of Mo6Cl84+ with L and Mn representing three-armed linker and polynuclear secondary building block, respectively. | |
Based on these considerations, we designed a semi-rigid three-armed organic linker of trimesoyltri(L-alanine) (TMTAH3) as shown in Scheme 2, which possesses the above-mentioned three characters and can link three polynuclear building blocks just like the chloride ion in Mo6C184+. Herein, we report a new discrete metal–organic nanocage of [Cu12(TMTA)8(H2O)12]·8CH3CH2OH·40H2O (1). Its structure, magnetic properties and gas adsorption properties were investigated.
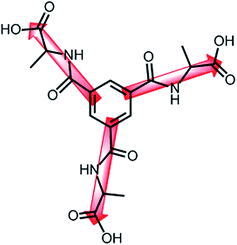 |
| Scheme 2 A schematic show of the three-armed linker of trimesoyltri(L-alanine) (TMTAH3) in this study. | |
Experimental section
Materials and physical measurements
Trimesoyltri(L-alanine) (TMTAH3) was prepared according to a literature method reported by our group.9 The other materials and reagents in this study were used as obtained commercially without further purification. Elemental analyses (C, H, and N) were performed on a Perkin-Elmer 2400II CHN elemental analyzer. The infrared spectra were recorded from KBr pellets in the range of 4000–400 cm−1 with a Perkin-Elmer spectrum one FTIR spectrometer. The powder X-ray diffraction (PXRD) data were collected on a Rigaku D/max 2500v/pc diffractometer with Cu-Kα radiation (λ = 1.5418 Å). All magnetic measurements were carried out on a Quantum Design MPMS-XL7 SQUID magnetometer. The experimental susceptibility data were corrected for the diamagnetic contribution calculated from Pascal constants, and the diamagnetism of the sample and sample holder were taken into account. The adsorption isotherms of H2 at 77 K and CO2 and CH4 at 298 K were measured with BELSORP-HP volumetric adsorption equipment from Bel Japan, Inc.
Synthesis of 1
A solid mixture of TMTAH3 (0.1 mmol, 0.0423 g) with CuCl2·2H2O (0.3 mmol, 0.0519 g) was dissolved in a mixed solvent of DMF (2 mL) and C2H5OH (8 mL). After being stirred for 20 minutes, 1 mol L−1 KOH (0.2 mL) were added. The resulted mixture was further stirred for 3 hours and then filtrated. The filtrate was allowed to evaporate the solvent at room temperature for seven weeks, giving green crystals which were collected, washed with ethanol, and then air-dried for six hours. Yield: 0.0176 g, 26% (calculated based on TMTAH3). Anal. calcd (%) for C160H296Cu12N24O132 (1): C, 35.39; H, 5.49; N, 6.19. Found: C, 35.70; H, 5.29; N, 6.42. IR (KBr pellet, cm−1): 3402 (m), 1646 (s), 1535 (m), 1453 (m), 1414 (m), 1393 (m), 1378 (m), 1344 (w), 1327 (w), 1289 (m), 1207 (w), 1151 (w), 1124 (w), 1106 (w), 1074 (w), 1053 (w), 695 (w).
X-ray crystallography
Crystallographic data for all complexes were collected on a Bruker SMART CCD diffractometer with graphite monochromated Mo-Kα radiation (λ = 0.71073 Å). Absorption correction was applied by using the multi-scan program SADABS.10 The structure was solved by direct methods of SHELXS-97 and refined by full-matrix least-squares against F2 using the SHELXTL package.11 The non-H atoms were refined again initially using isotropic and later anisotropic thermal displacement parameters. Complex 1 is very easy to volatilize the lattice guest molecules while exposed in air, which easily leads to the disorder of the guest molecules. Thus these crystalline solvent molecules could not be modeled properly and were removed by the SQUEEZE routine in PLATON.12 It shows a solvent-accessible volume of 11
761.7 Å3 (43.4%) per unit cell (27
100.0 Å3). The final formula was determined from the SQUEEZE results combined with the TG analysis and elemental analysis. The hydrogen atoms of coordinated water molecules were located in a difference Fourier map and refined isotropically in the final refinement cycles. The other hydrogen atoms were placed in calculated positions and refined using a riding model. Crystallographic data for compound 1 are given in Table S1.† Selected bond lengths and bond angles are given in Table S2.†
Results and discussion
Syntheses and general characterizations
The titled compounds were separated from the reaction of CuCl2 with TMTAH3 in the presence of KOH at room temperature by routine solution method. The optimized solvent is the mixed solvent of DMF with ethanol. The use of other solvents in this reaction could not give the titled compounds as expected. The change of CuCl2 into the other Cu(II) salts such as Cu(NO3)2 and Cu(ClO4)2 could also produce the titled compound, but with much poorer quality of the crystals. The experimental PXRD pattern of the microcrystals of 1 agrees well with the simulated one based on its crystal structure (Fig. S1†), confirming the phase purity of 1. The TG analysis (Fig. S2†) of 1 reveals a fast weight loss upon heating to 90 °C, followed by a plateau in the temperature range of 90–180 °C. The weight loss of 19.3% below 138 °C corresponds to the loss of all solvated molecules (calcd 20.06%). After the plateau, the weight loss is first fast and then slow, which might be due to the collapse of the cage framework. The weight loss is not complete even when the sample was heated to 936 °C.
Crystal structure
The single crystal X-ray analysis indicates that complex 1 crystallizes in the tetragonal space group P42212. The coordination asymmetrical unit of 1 contains six crystallographically independent Cu(II) ions, four TMTA3− ligands and six coordinated water molecules as shown in Fig. S3.† All Cu2+ ions in 1 are five-coordinated in square pyramidal geometries by four carboxylato O atoms from four different TMTA3− ligands in the square plane and one water molecule in the apical position. The equatorial Cu–O bond distances (1.944(5)–1.999(5) Å) are shorter than the axial Cu–O bond distances (2.131(5)–2.410(5) Å), which is attributed to the Jahn–Teller (JT) effect of Cu2+ ions. Two of such Cu(II) ions are bridged by four carboxylate groups from four different TMTA3− ligands, forming a paddlewheel binuclear [Cu2(COO)4(H2O)2] unit with a Cu⋯Cu distance of 2.6292(11)–2.6362(11) Å. All TMTA3− ligands in 1 behave as μ6:η6-bridges, linking six Cu(II) ions from three [Cu2(COO)4(H2O)2] units. Six paddlewheel [Cu2(COO)4(H2O)2] units are consolidated by eight TMTA3− ligands to form a [Cu12(TMTA)8(H2O)12] cage with a diameter of about 23.0(1) Å as shown in Fig. 1, S4 and S5.† It has a pore with an effective diameter of 5.7(1) Å (Fig. S5†). Each Cu(II) ion in this cage is coordinated by four TMTA3− linkers, which in return act as six-connecting linkers. This kind of connection for the whole cage can be depicted in Fig. 2a–c. Regarding the [Cu2(COO)4(H2O)2] unit as one node, the TMTA3− ligand can be viewed as a three-connecting linker and each [Cu2(COO)4(H2O)2] node interacts with four three-connecting linkers. This kind of linkage results in the construction of a Mo6C184+-like neutral octahedral cage (Fig. 2d), which is similar to the documented coordination cages.1b,13 These nanocages pack in the lattice in a face-centered cubic style as depicted in Fig. 3 and S6.†
 |
| Fig. 1 A perspective view of the cage viewed down the C3 symmetry axis (a) and the C4 axis (b). | |
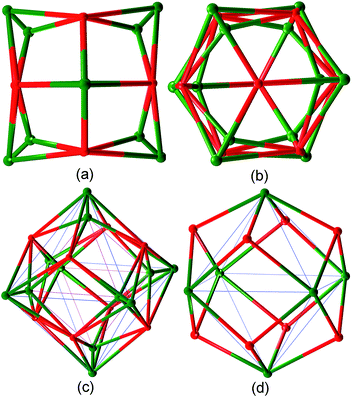 |
| Fig. 2 Topological views of 1 from different directions. Red spheres represent TMTA3− ligands, green spheres represent the Cu atoms in (a–c) and Cu2 binuclear units in (d). | |
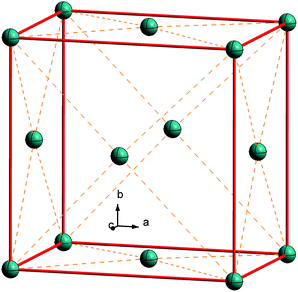 |
| Fig. 3 A schematic show of the packing diagram of the octahedral cages (cyan spheres) of 1. | |
Magnetic studies
The magnetic properties of 1 were investigated by the solid state magnetic susceptibility measurements in the temperature range 2–300 K in a DC field of 1000 Oe, which are shown in Fig. 4 in the form of χmT and χm versus T plots. It presents a χmT value of 4.51 cm3 K mol−1 at 300 K, which is nearly equal to that (4.50 cm3 K mol−1) calculated for twelve spin-only non-interacting Cu(II) ions. Upon cooling, the χmT value decreases gradually to 1.07 cm3 K mol−1 at 2 K. The profile of the χmT versus T plot suggests the presence of strong antiferromagnetic interactions between the metal ions in the title complex,14 which is mainly due to the intradinuclear magnetic interactions in the paddlewheel dinuclear [Cu2(COO)4(H2O)2] building units. It agrees well with the complexes containing paddlewheel dinuclear [Cu2(COO)4(H2O)2] secondary building units which show strong antiferromagnetic interactions between the intradinuclear Cu(II) ions through the face-on dx2–y2 orbitals by the four equatorial-to-equatorial carboxylate bridges.15
 |
| Fig. 4 Plots of χm and χmT vs. T for 1. | |
Gas sorption
The structural analysis of 1 reveals a volume of 11
761.7 Å3 (43.4%) per unit cell (27
100.0 Å3) for free solvent molecules and a void with effective diameter of 5.7(1) Å in the octahedral cage. There are also some functionalities of –C(
O)–NH– on the inner wall of the cage which favors to interact with other guest molecules.16 All of these features suggest that 1 might present gas adsorption properties. Thus the adsorption of H2, CO2 and CH4 gases for compound 1 was studied by measuring the corresponding adsorption isotherms using a volumetric gas-sorption apparatus. The polycrystalline samples of 1 were activated at 100 °C in a dynamic vacuum for 6 h before the adsorption measurements. The activated sample of 1 shows selectivity for gas adsorption. No apparent adsorption of CH4 was found for the pre-activated samples of 1. However, it can adsorb a certain amount of H2 and CO2 as revealed in their adsorption isotherms (Fig. 5). The high pressure hydrogen adsorption isotherm of 1 was measured at 77 K, which gives an uptake of 29.47 cm3 g−1 (0.27 wt%) at 20.4 atm. The high pressure CO2 adsorption isotherm of 1 was collected at 298 K, which presents an uptake of 15.68 cm3 g−1 (3.09 wt%) at 21.9 atm. It shows nearly the same increasing rate upon increasing the gas pressure below 8.87 atm for the adsorption of both H2 and CO2. The further increasing gas pressure leads to the same increasing rate for the adsorption of CO2, however a much higher increasing rate for the uptake of H2. The marked hysteresis loops are observed for both H2 and CO2 adsorption isotherms of 1, which reveals that the desolvated sample of 1 allows both H2 and CO2 to be stored at a pressure lower than that they are charged.9,17 Although the adsorption of H2 and CO2 for the desolvated sample of 1 is not high as expected, it really shows nice sorption selectivity for the gas molecules.
 |
| Fig. 5 H2 and CO2 adsorption isotherms of 1. Filled and open circles represent adsorption and desorption branches, respectively. Connecting traces are guides for eyes. | |
Conclusions
In conclusion, a novel Cu12 nanocage was built from the linkage of six paddlewheel dinuclear [Cu2(COO)4(H2O)2] secondary building units by the three-armed linker of trimesoyltri(L-alanine). It features a Mo6C184+-like structure. The magnetic investigation revealed the presence of dominant antiferromagnetic interaction between the adjacent metal ions of the title compound. The desolvated title compound shows nice selectivity for gas molecules as revealed by the adsorption measurements of H2, CO2 and CH4 gases. More examples for developing the synthetic strategies of aimed meta-organic nanocages are under way in our group.
Acknowledgements
We thank the financial supports by National Natural Foundation of China (Grant No. 21261004 and 21271050), Guangxi Natural Science Foundation of China (Grant No. 2013GXNSFGA019008 and 2013GXNSFAA019039).
Notes and references
-
(a) A. Mallick, B. Garai, D. D. Díaz and R. Banerjee, Angew. Chem., Int. Ed., 2013, 52, 13755 CrossRef CAS PubMed
;
(b) M. Paul, N. N. Adarsh and P. Dastidar, Cryst. Growth Des., 2014, 14, 1331 CrossRef CAS
;
(c) J. Park, Y.-P. Chen, Z. Perry, J.-R. Li and H.-C. Zhou, J. Am. Chem. Soc., 2014, 136, 16895 CrossRef CAS PubMed
;
(d) A. B. Grommet, J. L. Bolliger, C. Browne and J. R. Nitschke, Angew. Chem., Int. Ed., 2015, 54, 15100 CrossRef CAS PubMed
;
(e) W. Cullen, K. A. Thomas, C. A. Hunter and M. D. Ward, Chem. Sci., 2015, 6, 4025 RSC
. -
(a) A. C. Sudik, A. R. Millward, N. W. Ockwig, A. P. Côtét, J. Kim and O. M. Yaghi, J. Am. Chem. Soc., 2005, 127, 7110 CrossRef CAS PubMed
;
(b) D. J. Tranchemontagne, Z. Ni, M. O'Keeffe and O. M. Yaghi, Angew. Chem., Int. Ed., 2008, 47, 5136 CrossRef CAS PubMed
;
(c) N. Takeda, K. Umemoto, K. Yamaguchi and M. Fujita, Nature, 1999, 398, 794 CrossRef CAS
. -
(a) A. W. Augustyniak, M. Fandzloch, M. Domingo, I. Łakomska and J. A. R. Navarro, Chem. Commun., 2015, 51, 14724 RSC
;
(b) W. Lu, D. Yuan, A. Yakovenko and H.-C. Zhou, Chem. Commun., 2011, 47, 4968 RSC
;
(c) H.-N. Wang, X. Meng, G.-S. Yang, X.-L. Wang, K.-Z. Shao, Z.-M. Su and C.-G. Wang, Chem. Commun., 2011, 47, 7128 RSC
;
(d) J. Ma, Y. Ying, Q. Yang, Y. Ban, H. Huang, X. Guo, Y. Xiao, D. Liu, Y. Li, W. Yang and C. Zhong, Chem. Commun., 2015, 51, 4249 RSC
;
(e) J. Zhang, H. Yu, C. Zhang, C. He and C. Duan, New J. Chem., 2014, 38, 3137 RSC
;
(f) Y. Liu, X. Wu, C. He, Z. Li and C. Duan, Dalton Trans., 2010, 39, 7727 RSC
. -
(a) T. D. Hamilton, G. S. Papaefstathiou and L. R. MacGillivray, J. Solid State Chem., 2005, 178, 2409 CrossRef CAS
;
(b) X. Jing, C. He, Y. Yang and C. Duan, J. Am. Chem. Soc., 2015, 137, 3967 CrossRef CAS PubMed
;
(c) M. Zhang, W. Lu, J.-R. Li, M. Bosch, Y.-P. Chen, T.-F. Liu, Y. Liu and H.-C. Zhou, Inorg. Chem. Front., 2014, 1, 159 RSC
. -
(a) J. C. Sheldon, Nature, 1959, 184, 1210 CrossRef CAS
;
(b) F. A. Cotton and N. F. Curtis, Inorg. Chem., 1965, 4, 241 CrossRef CAS
;
(c) T. Saito, M. Nishida, T. Yamagata, Y. Yamagata and Y. Yamaguchi, Inorg. Chem., 1986, 25, 1111 CrossRef CAS
. -
(a) D. Kim, X. Liu, M. Oh, X. Song, Y. Zou, D. Singh, K. S. Kima and M. S. Lah, CrystEngComm, 2014, 16, 6391 RSC
;
(b) X. Liu, M. Park, S. Hong, M. Oh, J. W. Yoon, J.-S. Chang and M. S. Lah, Inorg. Chem., 2009, 48, 11507 CrossRef CAS PubMed
;
(c) M. Eddaoudi, J. Kim, J. B. Wachter, H. K. Chae, M. O'Keeffe and O. M. Yaghi, J. Am. Chem. Soc., 2001, 123, 4368 CrossRef CAS PubMed
. -
(a) D. L. Caulder and K. N. Raymond, Acc. Chem. Res., 1999, 32, 975 CrossRef CAS
;
(b) S. Leininger, B. Olenyuk and P. J. Stang, Chem. Rev., 2000, 100, 853 CrossRef CAS PubMed
. -
(a) Z. Chen, L. Quan, L. Liu, Y. Wang, H. Zou and F. Liang, Eur. J. Inorg. Chem., 2015, 1463 CrossRef CAS
;
(b) Y.-C. Ou, J. Wang, J.-D. Leng, Z.-J. Lin and M.-L. Tong, Dalton Trans., 2011, 40, 3592 RSC
;
(c) V. Gómez, M. Corbella, F. A. Mautner, O. Roubeau, S. J. Teat, M. Font-Bardia and T. Calvet, Polyhedron, 2012, 45, 185 CrossRef
;
(d) G. P. Guedes, I. F. Santos, L. A. Mercante, N. L. Speziali, J. A. L. C. Resende, A. M. R. Bernardino, M. Andruh and M. G. F. Vaz, Cryst. Growth Des., 2015, 15, 1027 CrossRef CAS
. - Z. Chen, X. Liu, C. Zhang, Z. Zhang and F. Liang, Dalton Trans., 2011, 40, 1911 RSC
. - G. M. Sheldrick, SADABS, 2.05, University of Göttingen, Germany, 2002 Search PubMed
. - G. M. Sheldrick, SHELXTL NT, version 5.1, University of Göttingen, Göttingen, Germany, 1997 Search PubMed
. - A. L. Spek, J. Appl. Crystallogr., 2003, 36, 7 CrossRef CAS
. -
(a) M. J. Prakash, Y. Zou, S. Hong, M. Park, M.-P. N. Bul, G. H. Seong and M. S. Lah, Inorg. Chem., 2009, 48, 1281 CrossRef CAS PubMed
;
(b) K. Harano, S. Hiraoka and M. Shionoya, J. Am. Chem. Soc., 2007, 129, 5300 CrossRef CAS PubMed
;
(c) K.-i. Yamashita, M. Kawano and M. Fujita, Chem. Commun., 2007, 4102 RSC
. -
(a) J. Svorec, P. Polakovičová, J. Moncol, V. Kuchtanin, M. Breza, S. Šoralová, Z. Padělková, J. Mrozinski, T. Lis and P. Segla, Polyhedron, 2014, 81, 216 CrossRef CAS
;
(b) A. Motreff, R. C. da Costa, H. Allouchi, M. Duttine, C. Mathonière, C. Duboc and J.-M. Vincent, Inorg. Chem., 2009, 48, 5623 CrossRef CAS PubMed
;
(c) H.-X. Zhang, Q.-X. Yao, X.-H. Jin, Z.-F. Ju and J. Zhang, CrystEngComm, 2009, 11, 1807 RSC
;
(d) L. K. Sposato, J. H. Nettleman, M. A. Braverman, R. M. Supkowski and R. L. LaDuca, Cryst. Growth Des., 2010, 10, 335 CrossRef CAS
. -
(a) L. K. Sposato, J. A. Nettleman and R. L. LaDuca, CrystEngComm, 2010, 12, 2374 RSC
;
(b) K. Hassanein, O. Castillo, C. J. Gómez-García, F. Zamora and P. Amo-Ochoa, Cryst. Growth Des., 2015, 15, 5485 CrossRef CAS
;
(c) D. L. Reger, A. Debreczeni, B. Reinecke, V. Rassolov and M. D. Smith, Inorg. Chem., 2009, 48, 8911 CrossRef CAS PubMed
;
(d) J. Zhao, W.-W. Dong, Y.-P. Wu, Y.-N. Wang, C. Wang, D.-S. Li and Q.-C. Zhang, J. Mater. Chem. A, 2015, 3, 6962 RSC
;
(e) D. W. Ryu, W. R. Lee, K. S. Lim, W. J. Phang and C. S. Hong, Cryst. Growth Des., 2014, 14, 6472 CrossRef CAS
. -
(a) H. Deng, C. J. Doonan, H. Furukawa, R. B. Ferreira, J. Towne, C. B. Knobler, B. Wang and O. M. Yaghi, Science, 2010, 327, 846 CrossRef CAS PubMed
;
(b) S. Hasegawa, S. Horike, R. Matsuda, S. Furukawa, K. Mochizuki, Y. Kinoshita and S. Kitagawa, J. Am. Chem. Soc., 2007, 129, 2607 CrossRef CAS PubMed
;
(c) R. J. Marshall, S. L. Griffin, C. Wilson and R. S. Forgan, J. Am. Chem. Soc., 2015, 137, 9527 CrossRef CAS PubMed
;
(d) P.-Q. Liao, W.-X. Zhang, J.-P. Zhang and X.-M. Chen, Nat. Chem., 2015, 6, 8697 CAS
. -
(a) H. J. Choi, M. Dinca and J. R. Long, J. Am. Chem. Soc., 2008, 130, 7848 CrossRef CAS PubMed
;
(b) H. Chun, D. N. Dybtsev, H. Kim and K. Kim, Chem.–Eur. J., 2005, 11, 3521 CrossRef CAS PubMed
;
(c) X. Zhao, B. Xiao, A. J. Fletcher, K. M. Thomas, D. Bradshaw and M. J. Rosseinsky, Science, 2004, 306, 1012 CrossRef CAS PubMed
;
(d) J.-M. Gu, T.-H. Kwon, J.-H. Park and S. Huh, Dalton Trans., 2010, 39, 5608 RSC
;
(e) P. Kanoo, K. L. Gurunatha and T. K. Maji, J. Mater. Chem., 2010, 20, 1322 RSC
.
Footnote |
† Electronic supplementary information (ESI) available: PXRD patterns, TG curve, additional structural figures, and tables for crystallographic data and selected bond lengths and angles. CCDC 1439771. For ESI and crystallographic data in CIF or other electronic format see DOI: 10.1039/c5ra26357c |
|
This journal is © The Royal Society of Chemistry 2016 |
Click here to see how this site uses Cookies. View our privacy policy here.