DOI:
10.1039/C5RA27751E
(Paper)
RSC Adv., 2016,
6, 27452-27459
Development of the catalytic reactivity of an oxo–peroxo Mo(VI) Schiff base complex supported on supermagnetic nanoparticles as a reusable green nanocatalyst for selective epoxidation of olefins†
Received
25th December 2015
, Accepted 2nd March 2016
First published on 4th March 2016
Abstract
A novel ancillary branch coated oxo–peroxo Mo(VI)tetradentate Schiff base complex on superparamagnetic nanoparticles was prepared and characterized by IR spectroscopy, X-ray powder diffraction (XRD), scanning electron microscopy (SEM), transmission electron microscopy (TEM), vibrating sample magnetometry (VSM), diffuse reflectance spectra (DRS) and atomic absorption spectroscopy (AAS). The catalyst was used for the selective epoxidation of cyclooctene, cyclohexene, styrene, indene, α-pinene, 1-hepten, 1-octene, 1-dodecen and trans-stilben using tert-butyl hydroperoxide as an oxidant in 1,2-dichloroethane. This catalyst is efficient for the oxidation of cyclooctene, with a moderate 100% selectivity for epoxidation with 97% conversion in 30 min. We were able to separate the supermagnetic nanocatalyst by using an external magnetic field, and to use the catalyst at least five successive times without significant decrease in conversion. The proposed supermagnetic nanocatalyst has advantages in catalytic activity, selectivity, catalytic reaction time and reusability by easy separation.
Introduction
Catalytic epoxidation of olefins is an interest of many researchers for the synthesis of fine chemicals. Because of their versatility as intermediates, epoxies are of great value in both synthetic organic chemistry and chemical technology.1 Molybdenum(VI) Schiff base complexes have been intensively used as oxidation catalysts for a variety of organic substrates, because molybdenum complexes offer significant advantages such as being economic, environmentally friendly and commercially available.2 Oxo–peroxo molybdenum complexes investigated particularly for epoxidation of olefins.3–6 The catalytic activity of peroxido molybdenum complexes is related on the number of peroxido ligands attached to the catalyst and the nature of the remaining ligands in the coordination sphere.7,8
TBHP is environment-friendly and has good thermal stability.9 Moreover, one of the main problems of homogeneous transition metal complexes as catalysts is the formation of oxo and peroxo dimeric and other polymeric species.10 The formation of these compounds can deactivate catalysts irreversibly. This problem may be solved by immobilizing of the metal complexes within the solid supports so it can separate complexes from each other.11 Meanwhile, the separation, recycling, insufficient stability of homogeneous catalysts and leaching of the active metal into the solvent are part of serious problems. Immobilization of the homogeneous metal complex onto solid supports has been the subject of a lot of research in catalytic fields because of long catalytic lifetime, easy separation, thermal stability, high selectivity and easy recyclability.12–14 A main method for heterogenizing of homogenous catalyst is to anchor the soluble catalyst on to large surface area inorganic supports.15,16 Many different solid catalysts such as zeolite and metal oxide can heterogenized homogeneous catalysts.17,18 Nowadays, core–shell superparamagnetic Fe3O4 nanoparticles have been used strongly because of their unique properties including the high surface area, low toxicity, separability and biocompatibility.19–21 Magnetic separation renders the recycling of catalysts from the solution by external magnetic fields much easier than by filtration and centrifugation. Meanwhile, using of core–shell can prevent the occurrence of aggregation of magnetic Fe3O4 nanoparticles, and endow the magnetic materials with favorable biocompatibility.
In this work we have reported the immobilization of N,N-bis(5-chloromethyl-salicylidene)-1,2-phenylenediamine oxoperoxo molybdenum(VI) (CM-salophMoO(O2)) onto the surface of amino-modified core–shell supermagnetic nanoparticles, Fe3O4@APTMS/CM-salophMoO(O2). The resulting heterogeneous catalysis has been characterized by using different techniques. We have been studying for selective alkene epoxidations, using tert-butyl hydroperoxide (70% aqueous) as oxidant. The catalytic performances of supported catalyst have been compared to homogeneous analogues. The reusability of prepared catalysis based on core–shell supermagnetic nanoparticles was also studied in the epoxidation of cyclooctene with tert-butyl hydroperoxide in 1,2-DCE.
Experimental section
Materials and physical measurements
All reagent used were purified using known procedures. 5-Chloromethyl-salicylaldehyde22 have prepared according to literature procedures. 1,2-Phenylenediamine, cyclooctene, cyclohexene, styrene, indene, α-pinene, 1-hepten, 1-octene, 1-dodecen, trans-stilben, 3-aminopropyltrimethoxysilane (APTMS), iron(II)chloride (FeCl2·4H2O), iron(III)chloride (FeCl3·6H2O), and ammoniumhydroxide (25% [w/w]) were purchased from Merck Chemical Company. 1H NMR spectra were recorded using a Bruker FT NMR 500 (500 MHz) spectrophotometer (CD3)2SO. Elemental analyses (carbon, hydrogen and nitrogen) were performed using a Heraeus Elemental Analyzer CHN-O-Rapid (Elementar-Analysesysteme, GmbH). Atomic absorption analysis was carried out on a shimadzu 120 spectrophotometer. The purity of the solvents, cyclooctene, cyclohexene, styrene, indene, α-pinene, 1-hepten, 1-octene, 1-dodecen, trans-stilben and analysis of the oxidation products was determined by gas chromatography using Agilent 7890 with a capillary column and FID detector. Column temperature was programmed between 180 °C and 200 °C (2 °C min−1). Nitrogen was used as carrier gas (40 ml min−1) at injection temperature. FT-IR spectra were obtained by Shimadzu 8400S spectrophotometer in KBr pellets. Diffuse reflectance spectra (DRS) were taken on a Scinco 4100 the range 200–1100 nm using BaSO4 as reference. The powder small angle X-ray diffraction studies were done on ITALSTRUCTURE X-ray diffractometer with CuKα (λ = 1.54 Å) radiation. The voltage and current applied to the X-ray tube were 40 kV and 30 mA, respectively, with scanning speed as 0.001° min−1. Surface morphology and distribution of particles were studied via VEGA-TESCAN scanning electron microscopy, using an accelerating voltage of 20 kV.
Preparation of Fe3O4@APTMS
The chemical co-precipitation was used for preparation of Fe3O4 nanoparticles.23 Then silica coated Fe3O4 NPs (Fe3O4@SiO2) synthesized by the hydrolysis of tetraethylorthosilicate (TEOS) using the sol–gel process.24 A suspension of Fe3O4@SiO2 (0.5 g) was dispersed in ethanol (50 ml), and 3-aminopropyltrimethoxysilane (APTMS) (2.5 ml) dissolved in 50 ml ethanol was added dropwise to the suspension. The reaction mixture was stirred at 70 °C for 5 h. Finally the brown aminated MNPs was separated magnetically and washed with distilled water for several times to remove any unbound APTMS. FT-IR (KBr, cm−1): 3406 [ν(O–H)], 1033 [ν(Si–O–Si)], 575 [ν(Fe–O)].
Preparation of N,N-bis(5-chloromethyl-salicylidene)-1,2-phenylenediamine (5-CM-saloph) (4)
5-Chloromethyl-2-hydroxybenzaldehyde was prepared and characterized as described in literature.25 A mixture of salicylaldehyde (0.25 mol), paraformaldehyde (0.15 mol) and 150 ml of conc. HCl was stirred at room temperature for 48 h. The white solid was filtered, washed with 0.5% NaHCO3 solution, dried and recrystallized in petroleum ether. The solution of (2.5 mmol, 0.27 g) 1,2-phenylenediamine in 20 ml dichloromethane was added dropwise to the solution of 5-chloromethylsalicyaldehyde (5 mmol, 0.85 g) in 20 ml dichloromethane. The mixture was refluxed for 2 h. The orange coloured precipitates were collected, and washed with reaction solvent and then dried in vacuum (yield 1 g, 96%). Anal. calcd for C22H18N2O2Cl2: C, 63.61; H, 4.81; N, 6.71. Found: C, 63.81; H, 4.51; N, 6.49. IR (KBr, cm−1): 1654 [ν(C
N)], 3401 [ν(O–H)]. 1H NMR (500 MHz, CDCl3, 25 °C) δppm: 11.2 (s, 2H, OH), 8.1 (s, 2H, Ar-CH
N), 6.8–7.5 (m, 10H, Ar), 4.22 (s, 4H, alkane) (ESI, Fig. S1†). 13C NMR (500 MHz, CDCl3): 55.9, 115.2, 118.3, 118.8, 119.6, 120.8, 127.7, 142.5, 152.2, 155.6, 163.3 (ESI, Fig. S2†).
Synthesis of 5-CM-salophen complex of oxoperoxomolybdenum(VI)
The CM-salophMoO(O2) complex is synthesized by dissolving 0.72 g of MoO3 (5 mmol) in hydrogen peroxide (30%, 10 ml) by stirring at room temperature. Then the N,N-bis(5-chloromethyl-salicylidene)-1,2-phenylenediamine (5 mmol) dissolved in a minimum volume of methanol and added the above solution under stirring gave yellow solid. The solid was filtered off, washed with water, methanol and finally with diethyl ether and dried in vacuo (yield 55%). Anal. calcd for C22H16N2O5Cl2Mo: C, 47.42; H, 3.26; N, 5.03; Mo, 17.23. Found: C, 47.01; H, 3.50; N, 4.7; Mo, 16.95. IR (KBr, cm−1): 1620 [ν(C
N)], 860 [ν(O–O)] and 944 [ν(Mo
O)]. 1H NMR (500 MHz, DMSO-d6, 25 °C) δppm: 8.6, 8.7 (s, 2H, Ar-CH
N), 6.8–7.8 (m, 10H, Ar), 4.3, 4.4 (s, 4H, alkane) (ESI, Fig. S3†). 13C NMR (500 MHz, DMSO-d6): 56.1, 57.1, 111.2, 111.7, 112.8, 114.6, 116.1, 117.5, 118.6, 119.6, 121.4, 123.2, 124.6, 143.2, 144.3, 155.4, 156.4, 161.2, 162.7, 166.3, 167.2, 169.3 (ESI, Fig. S4†). DRS (solid phase, nm): 368 and 435 nm.
Preparation of Fe3O4@APTMS/CM-salophMoO(O2)
A solution containing the CM-salophMoO(O2) complex (5 mmol, in 50 ml toluene) was added to a suspension of amine-functionalized Fe3O4@APTMS (1.0 g in 50 ml toluene). The resulting mixture was stirred at 70 °C for 24 h. After cooling, the brown Fe3O4@APTMS/CM-salophMoO(O2) nanocatalyst was separated using an external magnet and washed several times with water and ethanol.
Epoxidation of alkenes by catalyst
Epoxidation of alkenes such as cyclooctene, cyclohexene, styrene, indene, α-pinene, 1-hepten, 1-octene, 1-dodecen, trans-stilben using homogeneous and heterogeneous form of catalyst was carried out in a 25 ml Schlenk tube. All glassware's were oven-dried prior to use. The system was purged with argon gas. In a typical experiment, a mixture of 0.2 g of Fe3O4@APTMS/CM-salophMo(O2)O (2.3 × 10−5 mol for net complex), and 1, 2-dichloroethane (DCE) (5 ml), 5 mmol freshly distilled alkenes and 12.5 mmol of TBHP was refluxed for 2 h. The reaction followed by separation of the catalyst using an external magnetic field (for heterogeneous catalyst). The separated catalyst washed with absolute ethanol could be reused after drying at 80 °C under vacuum. 1,2-Dichlorobenzene was used as an internal standard. The solution was then injected to GC analysis.
Result and discussion
Synthesis and characterization
Scheme 1 shows the synthesis of tetradentate Schiff base (derived from 5-chloromethylsalicyaldehyde and 1,2-phenylenediamine) oxo–peroxomolybdenum(VI) complex and immobilization of complex on Fe3O4/SiO2 core–shell nanoparticles as a heterogeneous recyclable nanocatalyst.
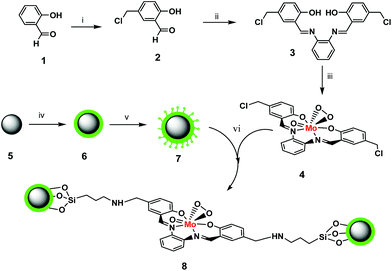 |
| Scheme 1 Preparation of heterogeneous nanocatalyst, (i) paraformaldehyde/HCl (ii) O-phenylenediamine (iii) MoO3/H2O2 (iv) FeCl2/FeCl3/NH3 (v) APTMS (vi) reflux in toluene/24 h. | |
1H NMR of Schiff base ligand and Mo complex confirm the formation of compounds 3 and 4. In the 1H NMR spectra of the Schiff base ligand the signal at 11.2, 8.1 and 4.22 assigned for H-phenol, H-imine and H-methylene respectively. By considering of the 1H NMR and 13C NMR spectra of Mo(VI) complex it seems that the more splitting is shown because of the non-equivalence chelate ring.
There is some additional information from a comparison of the infrared spectra of the Schiff base ligand, corresponding MoO(O2) complex and Fe3O4@APTMS/CM-salophMoO2 (Fig. 1).
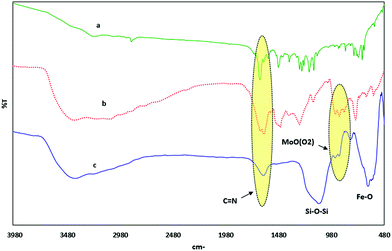 |
| Fig. 1 FT-IR spectrum of (a) 5-CM-saloph Schiff base ligand, (b) MoO(O2) Schiff base complex, (c) Fe3O4@APTMS/CM-salophMoO(O2). | |
The FT-IR spectrum of CM-saloph Schiff base ligand exhibit the C
N stretching vibration intense band at 1654 cm−, which shift to 1632 and 1630 cm−1 in the corresponding CM-salophMoO(O2) complex and Fe3O4@APTMS/CM-salophMoO(O2) respectively, indicating coordination of azomethine nitrogen.26 Also the ν(Mo
O) and ν(O–O) vibrations of CM-salophMoO(O2) complex appear around 929 cm−1 and 860 cm−1 respectively.27 The appearance of two bands in Fig. 1(c) at 1100 and 592 cm−1 can be assigned to Si–O–Si and Fe–O stretching, respectively. Comparison of all the FT-IR data suggest that the MoO(O2) group is bonded to the Schiff base ligand and then supported successfully on Fe3O4@APTMS.
The diffuse reflectance spectra (DRS) of the MoO(O2) complex and Fe3O4@APTMS/CM-salophMoO(O2) in Fig. 2 show two bands at 368 and 435 nm to assigned n–π* of Schiff base ligand and ligand-to metal charge transfer transitions, respectively.14,28 So the comparison of diffuse reflectance spectra (DRS) of the Fe3O4@APTMS/CM-salophMoO(O2) and CM-salophMoO(O2) show that there is not any geometrical change in oxo–peroxomolybdenum(VI) complex after supporting on Fe3O4@APTMS NPs.
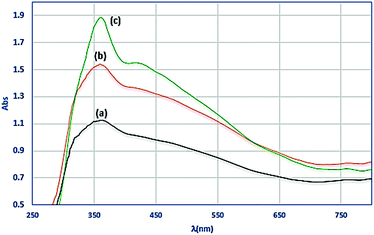 |
| Fig. 2 DRS spectrum of (a) salophMoO(O2) complex (b) Fe3O4@APTMS/CM-salophMoO(O2), (c) Fe3O4@APTMS/CM-salophMoO after catalysis. | |
The atomic absorption analysis gives the Mo content of heterogeneous catalyst 3.5 wt%.
As shown in Fig. 3, the X-ray diffraction pattern of crystalline structures of Fe3O4 NPs and core–shell magnetic Fe3O4@APTMS/CM-salophMoO(O2) show characteristic diffraction peaks correspond to (220), (311), (400), (422), (511) and (440) reflections of inverse spinel Fe3O4 NPs, respectively. It showed characteristic peaks, and the relative intensity matched well with those of standard Fe3O4 nanoparticles (reference JCPDS card no. 87-2334). The week broad peak appeared in the range from 2θ = 19 to 27 indicates the existence of amorphous silica. The crystal size of Fe3O4 NPs and core–shell magnetic Fe3O4@APTMS/CM-salophMoO(O2) were determined by using the Debye–Scherrer (d{hkl} = 0.94λ/β
cos
θ) equation where β is the half-width of the highest intensity X-ray diffraction lines, d is the average crystalline diameter, 0.94 is the Scherrer constant, θ is the Bragg angle in degree and λ is the X-ray wavelength. Here, the (311) peak of the highest intensity was selected and d311 obtained 25 nm for Fe3O4 NPs. Comparing between X-ray diffraction pattern of Fe3O4 NPs and Fe3O4@APTMS/CM-salophMoO(O2) shows that the coating of silica and salophMoO(O2) complex on Fe3O4 NPs did not significantly affect the structure of NPs.
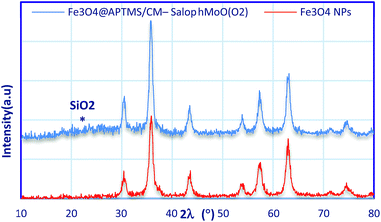 |
| Fig. 3 Small angle X-ray diffraction pattern of: (blue) Fe3O4@APTMS/CM-salophMoO(O2), (red) Fe3O4 nanoparticles. | |
As shown in Fig. 4, the plots of magnetization versus magnetic field show the absence of hysteresis phenomenon and indicate that product has superparamagnetism at room temperature. Fe3O4 and Fe3O4@APTMS/CM-salophMoO(O2) show the magnetic saturation is 55.6 and 38.12 emu g−1, respectively. These results indicated that the magnetization of Fe3O4 decreased considerably with the increase of SiO2 and Mo(O)2 Schiff base complex. However, the Fe3O4@APTMS/CM-salophMoO2 can still be separated from the solution by using an external magnetic field.
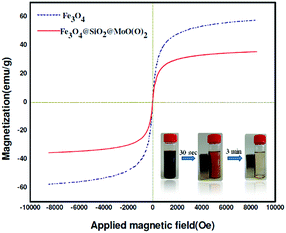 |
| Fig. 4 Magnetization curves at 298 K for Fe3O4 (dot) and Fe3O4@APTMS/CM-salophMoO(O2) (solid). | |
Thermogravimetric analysis have been used to understanding of thermal stability of supported catalyst. As shown in Fig. 5, the decomposition of the CM-salophMoO(O2) complex and Fe3O4@APTMS/CM-salophMoO(O2) catalyst were began 150 °C and 360 °C respectively. These criteria indicate that the Fe3O4@APTMS/CM-salophMoO(O2) catalyst is thermally stable than CM-salophMoO(O2).
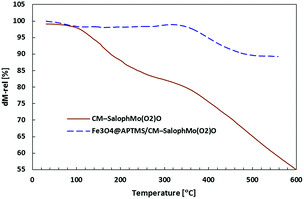 |
| Fig. 5 TG curve of Fe3O4@APTMS/CM-salophMoO(O2) (dash) and CM-salophMoO(O2) (solid). | |
Fig. 6 illustrates the SEM (Fig. 6(a and b)) and TEM (Fig. 6(c and d)) images of Fe3O4 and Fe3O4@APTMS/CM-salophMoO2. The size and morphology of the nanoparticles were observed using transmission electron microscopy (TEM). TEM images show that the particle size has changed after immobilization of complex on modified MNPs. The synthesized catalysts are well dispersed and most of the nanoparticles are almost spherical in shape. The average particle size estimated about 29 nm and 41 nm for Fe3O4 and Fe3O4@APTMS/CM-salophMoO2 respectively.
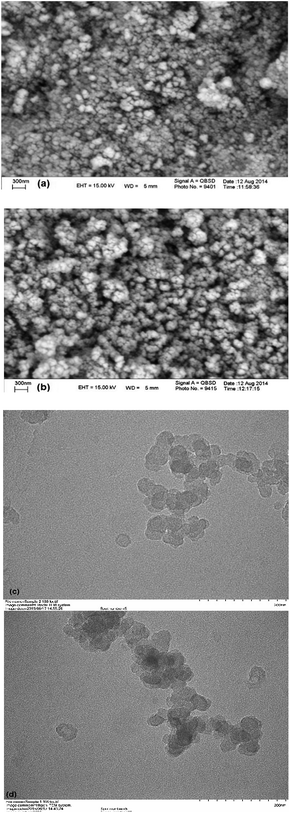 |
| Fig. 6 SEM images of (a) Fe3O4 NPs, (b) Fe3O4@APTMS/CM-salophMoO(O2) and TEM images of (c) Fe3O4 NPs, (d) Fe3O4@APTMS/CM-salophMoO(O2). | |
Epoxidation catalytic reactivity of CM-salophMoO(O2) (4) and Fe3O4@APTMS/CM-salophMoO(O2) (8)
Catalytic reactivity of heterogeneous, Fe3O4@APTMS/CM-salophMoO(O2) and homogeneous, CM-salophMoO(O2) catalysts were tested for the selective epoxidation of cyclooctene, cyclohexene, styrene, indene, α-pinene, 1-hepten, 1-octene, 1-dodecen and trans-stilben using tert-butylhydroperoxide (70% aqueous) as oxidant. The catalytic activities of Fe3O4@APTMS/CM-salophMoO(O2) were optimized for epoxidation of cyclooctene through investigation of the influence of solvent, the reaction temperature, the molar ratio of [TBHP]/[cyclooctene] and the time of epoxidation reaction. For selecting the best solvent, dichloromethane, methanol, acetonitrile, dichloroethane were employed as solvents. The optimizing results in Table 1 show that dichloroethane to be the best solvent. Apparently, highly coordinating solvents, such as CH3OH, cause a significant decrease in the catalytic activity since they compete with TBHP for binding to the Mo center.29 It seems that the lower reflux reaction temperature is the important reason of lowest conversion in CH2Cl2 (60%). The conversion increased with increasing the reaction temperature from 25 °C to 80 °C (Fig. 7).
Table 1 Optimization of Fe3O4@APTMS/CM-salophMoO(O2) catalytic condition, solvent = 5 ml; duration = 30 min with the molar ratio of cyclooctene
:
oxidant are 1
:
2
Solvent |
Amount of catalyst (g) |
T (°C) |
Oxidant |
Conversion (%)/selectivity (%) |
1,2-DCE |
Without cat. |
80 |
TBHP |
26/100 |
1,2-DCE |
0.005 |
80 |
TBHP |
56/100 |
1,2-DCE |
0.01 |
80 |
TBHP |
75/100 |
1,2-DCE |
0.02 |
80 |
TBHP |
97/100 |
1,2-DCE |
0.025 |
80 |
TBHP |
97/100 |
1,2-DCE |
0.02 |
70 |
TBHP |
87/100 |
1,2-DCE |
0.02 |
60 |
TBHP |
77/100 |
1,2-DCE |
0.02 |
40 |
TBHP |
60/100 |
CH3CN |
0.02 |
80 |
TBHP |
82/100 |
CH3OH |
0.02 |
Reflux |
TBHP |
60/100 |
CH2Cl2 |
0.02 |
Reflux |
TBHP |
58/100 |
1,2-DCE |
0.02 |
80 |
UHP |
55/100 |
1,2-DCE |
0.02 |
80 |
NaIO4 |
34/100 |
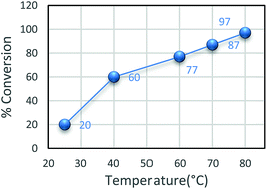 |
| Fig. 7 The effect of reaction temperature on conversion of cyclooctene. | |
Fig. 8 shows that the nanoparticles have good separation and dispersion by ultrasonication. After of ultrasonication the catalytic reactivity was tested and conversion increases during ultrasonication time form 32–100%.
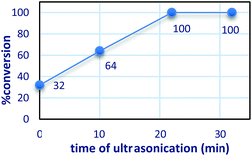 |
| Fig. 8 Effect of catalyst dispersion by ultrasonication before catalytic process. | |
The percent of conversion increased with increasing the molar ratio of [TBHP]
:
[cyclooctene] from 0.5 to 2 and the conversion of cyclooctene was maximum at 2
:
1 molar ratio of [TBHP]
:
[cyclooctene] (Fig. 9).
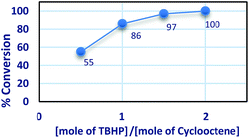 |
| Fig. 9 Effect of [TBHP]/[cyclooctene] ratio on cyclooctene conversion at 80 °C. | |
In this research work, different oxidants such as NaIO4, UHP (urea–H2O2) and TBHP (tert-butylhydroperoxide) were examined in epoxidation of cyclooctene in variable solvents. TBHP has some advantageous: (i) it can give high percent of conversion; (ii) environment-friendly; (iii) it has good thermal stability. Table 1 shows that TBHP as the oxygen donor has the highest conversion in the optimized reaction condition. Hence, typical catalytic reaction conditions involve 1,2-DCE (5 ml) solutions at 80 ± 2 °C, alkene (5 mmol), TBHP (12.5 mmol) with the molar ratio of cyclooctene
:
TBHP and catalyst (0.0074 mmol), 0.02 g for Fe3O4@APTMS/CM-salophMoO(O2) stirred for 30 min for cyclooctene and 60 min for the other olefins. The final solution exhibited no color. So, no presence of metal was detected in the solution after using heterogeneous catalyst, (it was confirmed by atomic absorption spectroscopy). The product distributions in the epoxidation of various alkenes using net complex and heterogeneous catalysts are shown in Fig. 11. Generally, one of the main problems of homogeneous transition metal complexes as catalysts is the formation of oxo and peroxo dimeric and other polymeric species. The formation of these compounds can deactivate catalysts irreversibly. As shown in Fig. 11, Fe3O4@APTMS/CM-salophMoO(O2) gives higher percent of conversion of alkenes than the CM-salophMoO(O2) complex.
Fig. 10 shows the chromatograms of the cyclooctene oxidation proceeds with a moderate 100% selectivity for epoxidation with 97% conversion in 30 min for Fe3O4@APTMS/CM-salophMoO(O2) catalyst. In the event that the CM-salophMoO(O2) complex shows 75% conversion in the same condition. This trend was illustrated for all alkenes in Fig. 11. Catalytic reactions were not affected by the presence or absence of light. The catalytic oxidation of substrate with TBHP in the absence of catalysts (blank run) occurs with low conversion (∼26%). We were able to separate supermagnetically nanocatalyst by using external magnetic field and use the catalyst at least five successive times without significant decrease in conversion (Table 2).
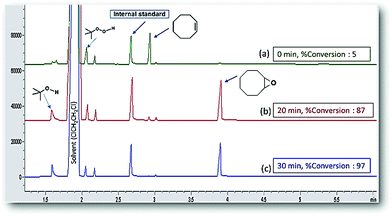 |
| Fig. 10 Typical chromatogram taken during of catalytic process, reaction time (a) 0 min, (b) 20 min and (c) 30 min with 100% selectivity. | |
 |
| Fig. 11 Product distributions in the epoxidation of various alkenes using net complex and heterogeneous complex. | |
Table 2 The results obtained from catalyst reuse in the epoxidation of cyclooctenea
Run |
% conversiona |
% epoxya |
% Mo leachedb |
Catalytic condition, solvent = 5 ml; duration = 30 min with the molar ratio of cyclooctene : oxidant are 1 : 2. Detected by atomic absorption spectroscopy. |
1 |
100 |
100 |
0 |
2 |
100 |
100 |
0 |
3 |
99 |
100 |
0 |
4 |
99 |
100 |
0 |
5 |
99 |
100 |
0 |
Scheme 2 shows a proposed mechanism via an interaction between the olefin HOMO π(C
C) and the unoccupied σ*(O–O) orbital of peroxo complex.40,41 In the first step, the electron density is redistributed from the C–C bonding olefin orbital to σ*(O–O) orbital via breaking of O–O bond and then epoxide is generated. After that, proton of TBHP is transferred to the terminal of oxo atom of the MoO2 and t-BuOO− is coordinated to Mo(VI) as a Lewis acidic metal center. Finally, forming of hydrogen bond between the coordinated t-BuOO− and OH, releases catalyst and alcohol. Table 3 compares the efficiency of our catalyst with some found in the literature. The illustrated Fe3O4@APTMS/CM-salophMoO(O2) catalytic system is one of the fastest magnetically recoverable catalytic system for epoxidation of alkenes. Our novel catalyst showed higher catalytic activity than the [MoO(O2)2(H2O) (ONnDodec3)],30 [MoO(O2)2(HMPT)],31 [Mo(O)(O2)2(bipy)]32 and [MoO2(py)2]-MCM41(ref. 34) for the epoxidation of cyclooctene. So the comparison of epoxidation reaction time of our catalyst (30 min) with the other reports (4 h),35 (24 h),37 (5 h)38 and (8 h)39 is many considerable.
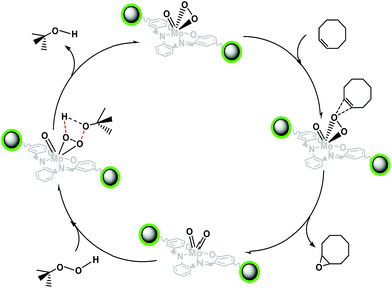 |
| Scheme 2 Proposed mechanism for alkene epoxidation by the Fe3O4@APTMS/CM-salophMo(O2)O. | |
Table 3 Comparison of literature reports on the epoxidation of cyclooctene under various conditions
Catalyst |
Reaction conditions |
% epoxy |
Ref. |
[MoO(O2)2(H2O)(ONnDodec3)] |
H2O2/CH3CN/24 h |
75 |
30 |
[MoO(O2)2(HMPT)] |
H2O2/DCE/5 h |
14 |
31 |
[Mo(O)(O2)2(bipy)] |
UHP/C4mim(PF6)/8 h |
90 |
32 |
[Mo(O)(O2)2(dmpz)2] |
H2O2/CH3Cl/18 h |
86 |
33 |
[MoO2(py)2]-MCM41 |
TBHP/CHCl3/7 h |
89 |
34 |
MoO2acpyAmpMCM-41 |
TBHP/CHCl3/4h |
99 |
35 |
[Mo(O)(O2)2(L-L)]SMNPs |
TBHP/CH3Cl/6 h |
96 |
36 |
MoO2-thio-SCMNPs |
TBHP/CHCl3/24 h |
100 |
37 |
Mag-Mo-nanocatalyst |
TBHP/CCl4/5 h |
99 |
38 |
MoO2(L)(EtOH)ZBS-PVPA |
TBHP/DCE/8h |
100 |
39 |
Fe3O4@APTMS/CM-salophMoO(O2) |
TBHP/DCE/30 min |
97 |
This work |
Conclusions
As a part of our progressing studies on the catalyst, Novel ancillary branch coated of oxo–peroxo Mo(VI) tetradentate Schiff base complex on superparamagnetic nanoparticles was prepared successfully and characterized by physico-chemical methods. The solids containing immobilized Mo(VI) tetradentate Schiff base complex has been studied as efficient heterogeneous nanocatalyst for chemoselective epoxidation of olefins. Oxidation result of Fe3O4@APTMS/CM-salophMoO(O2) was comparable with net CM-salophMoO(O2). In this report the conversion of heterogeneous catalyst was greater then the homogenous catalyst for all of olefins epoxidation. Also the Fe3O4@APTMS/CM-salophMoO(O2) can be separated by using a small external magnetic field from the reaction system and reused for at least 5 times without significant decrease in conversion. Our novel catalyst showed higher catalytic activity than the other Mo(VI) complexes reported in literature.30–39 The illustrated superparamagnetic catalyst shows excellent conversion (97%) in 30 min.
Acknowledgements
Authors are grateful to the Research Council of Mohaghegh-e-Ardabili University for their financial support.
Notes and references
-
(a) K. A. Srinivas, A. Kumar and S. M. S. Chauhan, Chem. Commun., 2002, 2456–2457 RSC;
(b) M. R. dos Santos, J. R. Diniz, A. M. Arouca, A. F. Gomes, F. C. Gozzo, S. M. Tamborim, A. L. Parize, P. A. Z. Suarez and B. A. D. Neto, ChemSusChem, 2012, 5, 716–726 CrossRef CAS PubMed;
(c) D. M. Boghaei, A. Bezaatpour and M. Behzad, J. Mol. Catal. A: Chem., 2006, 245, 12–16 CrossRef CAS;
(d) J. Rahchamani, M. Behzad, A. Bezaatpour, V. Jahed, G. Dutkiewicz, M. Kubicki and M. Salehi, Polyhedron, 2011, 30, 2611–2618 CrossRef CAS.
-
(a) A. Lazar, W. R. Thiel and A. P. Singh, RSC Adv., 2014, 4, 14063–14073 RSC;
(b) G. Fioroni, F. Fringuelli, F. Pizzo and L. Vaccaro, Green Chem., 2003, 5, 425–428 RSC;
(c) M. C.-Y. Tang, K.-Y. Wong and T. H. Chan, Chem. Commun., 2005, 50, 1345–1347 RSC.
- J. A. Brito, M. Go'mez, G. Muller, H. Teruel, J. Clinet, E. Duñach and M. A. Maestro, Eur. J. Inorg. Chem., 2004, 4278–4285 CrossRef CAS.
- A. V. Biradar, M. K. Dongare and S. B. Umbarkar, Tetrahedron Lett., 2009, 50, 2885–2888 CrossRef CAS.
- R. J. Cross, P. D. Newman, R. D. Peacock and D. Stirling, J. Mol. Catal. A: Chem., 1999, 144, 273–284 CrossRef CAS.
- V. Conte, F. Di Furia and G. Modena, in Organic Peroxides, ed. W. Ando, Wiley, Chichester, 1992, pp. 559–598 Search PubMed.
- R. Curci and J. O. Edwards, in Catalytic Oxidations with Hydrogen Peroxide as Oxidant, ed. G. Strukul, Kluwer, Dordrecht, 1992, pp. 57–60 Search PubMed.
- J. A. L. da Silva, J. J. R. F. da Silva and A. J. L. Pombeiro, Coord. Chem. Rev., 2011, 255, 2232–2248 CrossRef CAS.
-
(a) K. A. Jorgensen, Chem. Rev., 1989, 89, 431–458 CrossRef;
(b) J. M. Brégeault, J. Chem. Soc., Dalton Trans., 2003, 3289–3302 RSC.
-
(a) K. J. Balkus Jr, A. K. Khanrnamedova, K. M. Dixon and F. Bedioui, Appl. Catal., A, 1996, 143, 159–173 CrossRef;
(b) R. Belal and B. Meunier, J. Mol. Catal., 1988, 44, 187–190 CrossRef CAS;
(c) M. Salavati-Niasari, M. Shakouri-Arani and F. Davar, Microporous Mesoporous Mater., 2008, 116, 77–85 CrossRef CAS;
(d) K. O. Xaviera, J. Chackoa and K. K. Mohammed Yusuff, Appl. Catal., A, 1996, 258, 251–259 CrossRef.
- K. J. Ballus Jr, A. K. Khanmamedova, K. M. Dixon and F. Bedioui, Appl. Catal., A, 1996, 143, 159–173 CrossRef.
-
(a) A. Bezaatpour, M. Amiri and V. Jahed, J. Coord. Chem., 2011, 64, 1837–1847 CrossRef CAS;
(b) A. Bezaatpour, React. Kinet., Mech. Catal., 2014, 112, 453–465 CrossRef CAS.
- A. Hu, S. Liu and W. Lin, RSC Adv., 2012, 2, 2576–2580 RSC.
-
(a) A. Bezaatpour, M. Behzad, V. Jahed, M. Amiri, Y. Mansoori, Z. Rajabalizadeh and S. Sarvi, React. Kinet., Mech. Catal., 2012, 107, 367–381 CrossRef CAS;
(b) M. Bagherzadeh and M. Zare, Coord. Chem., 2013, 66, 2885–2900 CrossRef CAS.
- C. Baleizão, B. Gigante, D. Das, M. Álvaro, H. Garcia and A. Corma, Catalysis, 2004, 223, 106–113 CrossRef.
- C. A. McNamara, M. J. Dixion and M. Bradely, Chem. Rev., 2002, 102, 3275–3300 CrossRef CAS PubMed.
- H. V. Bekkum and J. C. Vander Waal, J. Mol. Catal. A: Chem., 1997, 124, 137–146 CrossRef.
- S. Imamura, H. Sakai, M. Shono and H. Kanai, Catalysis, 1998, 177, 72–81 CrossRef CAS.
- Y. S. Lin and C. L. Haynes, Chem. Mater., 2009, 21, 3979–3986 CrossRef CAS.
- M. Mahmoudi, S. Sant, B. Wang, S. Laurent and T. Sen, Adv. Drug Delivery Rev., 2011, 63, 24–46 CrossRef CAS PubMed.
- M. Sevilla, T. Valdés-Solis, P. Tartaj and A. B. Fuertes, J. Colloid Interface Sci., 2009, 340, 230–236 CrossRef CAS PubMed.
- T. Joseph, D. Srinivas, C. S. Gopinath and S. B. Halligudi, Catal. Lett., 2002, 83, 209–214 CrossRef CAS.
- Z. G. Peng, K. Hidajat and M. S. Uddin, J. Colloid Interface Sci., 2004, 271, 277–283 CrossRef CAS PubMed.
- L. Chen, B. Li and D. Liu, Catal. Lett., 2014, 144, 1053–1061 CrossRef CAS.
- A. Z. El-Sonbati, A. A. El-Bindary and I. G. Rashed, Spectrochim. Acta, Part A, 2002, 58, 1411–1424 CrossRef CAS.
- W. E. Hill, N. Atabay, C. A. McAuliffe, F. P. McCullough and S. M. Razzoki, Inorg. Chim. Acta, 1979, 35, 35–41 CrossRef CAS.
- N. Gharah, S. Chakraborty, A. K. Mukherjee and R. Bhattacharyya, Inorg. Chim. Acta, 2009, 362, 1089–1100 CrossRef CAS.
- M. Bagherzadeh, M. Zare, V. Amani, A. Ellern and L. K. Woo, Polyhedron, 2013, 53, 223–229 CrossRef CAS.
- F. E. Kühn, M. Groarke, E. Bencze, E. Herdtweck, A. Prazeres, A. M. Santos, M. J. Calhorda, C. C. Romão, I. S. Gonçalves, A. D. Lopes and M. Pillinger, Chem.–Eur. J., 2002, 8, 2370–2383 CrossRef.
- G. Wahl, D. Kleinhenz, A. Schorm, J. Sundermeyer, R. Stowasser, C. Rummey, G. Bringmann, C. Fickert and W. Kiefer, Chem.–Eur. J., 1999, 5, 32–37 CrossRef.
- M. Quenard, V. Bonmarin and G. Gelbard, Tetrahedron Lett., 1987, 28, 2237–2238 CrossRef CAS.
- M. Herbert, F. Montilla, R. Moyano, A. Pastor, E. Álvarez and A. Galindo, Polyhedron, 2009, 28, 3929–3934 CrossRef CAS.
- M. Herbert, F. Montilla and A. Galindo, J. Mol. Catal. A: Chem., 2011, 338, 111–120 CAS.
- A. Sakthivel, Y. Sakthivel, F. M. Pedro, A. S. T. Chiang and F. E. Kuhn, Appl. Catal., A, 2005, 281, 267–273 CrossRef CAS.
- M. Masteri-Farahani, F. Farzaneh and M. Ghandi, J. Mol. Catal. A: Chem., 2006, 248, 53–60 CrossRef CAS.
- S. Shylesh, J. Schweizer, S. Demeshko, V. Schünemann, S. Ernst and W. R. Thiel, Adv. Synth. Catal., 2009, 351, 1789–1795 CrossRef CAS.
- M. Mohammadikish and M. Masteri-Farahani, J. Magn. Magn. Mater., 2014, 354, 317–323 CrossRef CAS.
- Y. Shokouhimehr, J. Piao, Y. Kim and M. Jang, Angew. Chem., 2007, 119, 7169–7176 CrossRef.
- Y. Li, X. Fu, B. Gong, X. Zou, X. Tu and J. Chen, J. Mol. Catal. A: Chem., 2010, 322, 55–62 CrossRef CAS.
- I. V. Yudanov, C. D. Valentin, P. Gisdakis and N. Rosch, J. Mol. Catal. A: Chem., 2000, 158, 189–197 CrossRef CAS.
- M. Bagherzadeh, M. Amini, H. Parastar, M. Jalali-Heravi, A. Ellern and L. K. Woo, Inorg. Chem. Commun., 2012, 20, 86–89 CrossRef CAS.
Footnote |
† Electronic supplementary information (ESI) available: Fig. S1: 1H NMR of N,N-bis(5-chloromethyl-salicylidene)-1,2-phenylenediamine (5-CM-salop), Fig. S2: 1H NMR of 5-CM-salophMo(O2)O complex Fig. S3: 13C NMR of 5-CM-salophMo(O2)O complex. See DOI: 10.1039/c5ra27751e |
|
This journal is © The Royal Society of Chemistry 2016 |
Click here to see how this site uses Cookies. View our privacy policy here.