DOI:
10.1039/C6RA00143B
(Paper)
RSC Adv., 2016,
6, 25302-25310
Organosoluble and high Tg polyimides from asymmetric diamines containing N-amino and N-aminophenyl naphthalimide moieties†
Received
4th January 2016
, Accepted 1st March 2016
First published on 2nd March 2016
Abstract
To investigate the asymmetric effect of the naphthalimide moiety on the thermal, mechanical, optical properties and water uptake of polyimides (PIs), two novel asymmetric diamines, 4-(4-aminophenoxy)-N-amino-1,8-naphthalimide 3 and 4-(4-aminophenoxy)-N-(4-aminophenyl)-1,8-naphthalimide 4 were synthesized. Two series of PIs 5b–e and 6a–e were prepared from diamines 3 and 4 by solution polymerization method, respectively. The resulting polyimides demonstrated good solubility, high glass transition temperature (Tg) of 311–421 °C and good thermal stability based on the 5% weight loss temperature (T5%) of 435–563 °C in nitrogen. These PIs also exhibited good mechanical properties with tensile strengths of 107.3–172.2 MPa, tensile moduli of 2.8–4.9 GPa, and elongations at break of 2.9–9.1%. The water uptake of PIs was in the range of 0.33–1.96%. The polyimides displayed UV-vis absorption maxima and an intense fluorescent intensity in the range of 361–371 and 480–700 nm, respectively.
1. Introduction
Polyimides (PIs), an important type of high temperature polymer, have found applications in adhesives, composite matrices, optoelectronics and aerospace due to their excellent thermal, mechanical and electrical properties as well as outstanding chemical resistance.1–3 However, the wide applications for PIs are often limited due to their poor organosolubility as well as high melt viscosity, derived from the rigid backbones and strong inter-chain interactions. Some structural modifications, such as the incorporation of flexible ether linkages4–11 and use of geometrically or molecularly asymmetric diamine monomers,12–18 resulted into better solubility and processability of polyimides. Generally, it has been noticed that ether linkages lead to low glass transition temperature (Tg) of PIs due to reduced chain stiffness. On the other hand, the combination of a rigid and bulky naphthalimide ring with ether linkage into the polymer chains can increase Tg and thermal stability of PIs by decreasing segmental mobility of polymer chain without deteriorating their solubility.19,20
In the past few decades, several research groups reported poly(ether imide)s having a N–N linkage (I) between naphthalimide and phthalimide groups in the polymer backbone (Fig. 1). These PIs exhibited good solubility, very high Tg, excellent thermal stability and mechanical properties.21–24 These PIs with N–N linkage were mainly prepared from symmetric bis(N-amino naphthalimide)s (II) which acted as diamines when reacted with aromatic dianhydrides. However, poor reactivity and solubility of these symmetric diamines in common organic solvents, confined the synthesis of corresponding bisimide (I) based PIs to one-step polymerization method.22,23
 |
| Fig. 1 Different structures of naphthalimide based diamines and bisimide units in polyimide. | |
The introduction of a benzene ring into the N-amino naphthalimide moiety may overcome the problem of poor reactivity of diamine and offers bisimide (III) based PIs by conventional two-step polymerization (Fig. 1). In addition, structurally more rigid bisimide (III) based PIs are also expected to show higher thermal stability and mechanical properties than PIs containing bisimide (I).
As far we know, there are limited reports about the asymmetric diamines containing naphthalimide group. For a better comparison to investigate the effect of bisimides (I) and (III) on polyimides properties, we designed two novel asymmetric diamines 3 and 4, and synthesized their corresponding PIs 5b–e and 6a–e, respectively. The properties of both series of PIs, such as solubility, thermal, mechanical and optical properties as well as water uptake are studied.
2. Experimental
2.1. Materials
4-Bromo-1,8-naphthalic anhydride was purchased from Aladdin Chemical Co. and used as received. 4-Amino-benzenephenol was purified by two times recrystallization from hot water. Pyromellitic dianhydride (PMDA) and p-phenylenediamine (p-PDA) were sublimed before use. 4,4′-Oxydiphthalic anhydride (ODPA), 4,4′-biphthalic dianhydride (BPDA), 3,3′,4,4′-benzophenone tetracarboxylic dianhydride (BTDA), 4,4′-bisphenol A dianhydride (BPADA) and other reagents used in this study were purchased from Shanghai Chemical Reagent Plant and used without further purification.
2.2. Instrumentation
The 1H NMR spectra were measured at 400 MHz on a Bruker 400 AVANCE III spectrometer, using dimethyl sulfoxide-d6 (DMSO-d6) as solvent. Melting points were determined on an XT4-100B melting point apparatus (Beijing Keyi Elec-opti instrument) and were uncorrected. The Fourier transform infrared spectra (FT-IR) were obtained with a Thermo Nicolet 6700 FT-IR spectrometer, where the sample was prepared with KBr pellets. The UV-vis spectra were recorded on a PerkinElmer Lambda 950 spectrophotometer at room temperature in the absorption mode. The fluorescence spectra were obtained from a Horiba Fluoromax 4 spectrofluorometer. The inherent viscosities were measured with an Ubbelodhe viscometer at 30 ± 0.1 °C in m-cresol or N-methyl-2-pyrrolidone (NMP) at a concentration of 0.5 g dL−1. Thermogravimetric analysis (TGA) was performed on a Perkin-Elmer Diamond TG/DTA instrument at a heating rate of 10 °C min−1 in the temperature range of 50 to 800 °C under nitrogen atmosphere (flow rate of 50 mL min−1). Differential scanning calorimetry (DSC) was carried out using Mettler Toledo DSC at a heating rate of 20 °C min−1 in the temperature range of 50 to 450 °C under nitrogen atmosphere with 50 mL min−1 gas flow, and Tg was reported as temperature at the middle of the thermal transition in the second heating scan after deleting thermal history. The tensile strength measurements were taken using five PI film samples by an Instron model 5567 at a crosshead speed of 5 mm min−1 at room temperature, and the results were averaged. The specimen gauge lengths were 50 mm and the specimen widths were 10 mm. To achieve ground state geometry, energy minimized structures were drawn by Gaussian software using quantum chemistry method. The wide-angle X-ray diffraction (WAXD) measurement of the polyimide films was undertaken on a Bruker D8 Advance with Cu Kα radiation (40 kV, 40 mA) from 5 to 50°. Water uptake was measured according to Japanese Industrial Standard (JIS) K 6911: a specimen was dried in an oven at 50 °C for 24 h and was cooled to room temperature in a desiccator over silica gel. After the dry specimen was weighted by using a Mettler Toledo Analytical Balance AL204, it was soaked in deionized water at 25 °C for 24 h. After the specimen was taken out from the water, the external water was removed, and the specimen was weighted in 1 min. Three specimens were used for each polymer, and the water uptake was reported as the average. The water uptake (WU) was determined by the weighing of the changes in vacuum-dried film specimens before and after immersion in deionized water at 25 °C using the following eqn (1): |
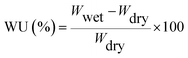 | (1) |
where Wdry is the initial weight of the film samples and Wwet is the weight of the film samples after immersion in deionized water. The water uptake of the polyimides is the mean data of three parallel samples. The qualitative solubility of polymers was tested with 20 mg of a sample in 2 mL of the solvent. Limited oxygen index (LOI) of the polymers can be calculated by using Van Krevelen and Hoftyzer eqn (2), which have char yield as decisive factor.where CR = char yield at 800 °C.
2.3. Monomer synthesis
2.3.1 4-Bromo-N-amino-1,8-naphthalimide 1. A mixture of 4-bromo-1,8-naphthalic anhydride (2.7701 g, 10.0 mmol), hydrazine hydrate (1.5010 g, 30.0 mmol) and ethanol (50 mL) was stirred at 80 °C for 4 h under nitrogen atmosphere. Reaction mixture was cooled to room temperature, the yellow precipitates were filtered off, washed with ethanol (50 mL) and dried in vacuum at 80 °C for 24 h. 4-Bromo-N-amino-1,8-naphthalimide 1 was purely obtained as a yellow solid (2.7613 g, 95%). Melting point: 218 °C; FT-IR (KBr): 3345, 3316, 1701, 1652, 1586, 1386, 775 cm−1; 1H NMR (DMSO-d6): 8.58 (d, 1H, J = 7.2 Hz), 8.55 (d, 1H, J = 8.4 Hz), 8.34 (d, 1H, J = 7.6 Hz), 8.22 (d, 1H, J = 7.6 Hz), 8.01 (t, 1H, J = 8.0 Hz), 5.81 (s, 2H).
2.3.2 4-Bromo-N-(4-aminophenyl)-1,8-naphthalimide 2. A mixture of 4-bromo-1,8-naphthalic anhydride (1.0421 g, 3.8 mmol), p-PDA (0.5020 g, 4.6 mmol) and ethanol (30 mL) was refluxed for 6 h under nitrogen atmosphere. Reaction mixture was filtered in hot state to remove excess p-PDA and further washed with ethanol (50 mL) and dried in vacuum at 80 °C for 16 h. 4-Bromo-N-(4-aminophenyl)-1,8-naphthalimide 2 was purely obtained as a greyish white solid (1.3042 g, 95%). Melting point: 260 °C; FT-IR (KBr): 3472, 3373, 1706, 1655, 1615, 1569, 1587, 1515, 1369, 1242, 783 cm−1; 1H NMR (DMSO-d6): 8.55 (d, 2H, J = 8.0 Hz), 8.31 (d, 1H, J = 7.8 Hz), 8.22 (d, 1H, J = 7.8 Hz), 8.0 (t, 1H, J = 7.8 Hz), 6.95 (d, 2H, J = 8.5 Hz), 6.64 (d, 2H, J = 8.5 Hz), 5.27 (s, 2H); 13C NMR (DMSO-d6): 163.9, 163.8, 149.0, 132.9, 132.0, 131.8, 131.4, 130.3, 129.6, 129.4, 129.3, 129.1, 123.9, 123.2, 114.1.
2.3.3 4-(4-Aminophenoxy)-N-amino-1,8-naphthalimide 3. 4-Bromo-N-amino-1,8-naphthalimide 1 (10.7110 g, 36.8 mmol), 4-aminophenol (8.4323 g, 77.3 mmol) and anhydrous potassium carbonate (10.6712 g, 77.3 mmol) were mixed in a 500 mL flask containing a mixture (200 mL) of acetonitrile and N,N-dimethylformamide (4
:
1). The reaction mixture was heated at 120 °C for 16 h. The solvents were evaporated from reaction mixture under reduced pressure and distilled water was added to obtain product. The crude product was filtered and washed with hot water to remove excess 4-aminophenol. The resulting pure brown precipitate of 4-(4-aminophenoxy)-N-amino-1,8-naphthalimide 3 (11.1801 g, 95%) were dried in oven at 80 °C for 24 h. Melting point: 229–232 °C. FT-IR (KBr): 3349, 3220, 1654, 1591, 1502, 1377, 1235, 1069, 1016, 951, 844, 784 cm−1; 1H NMR (DMSO-d6): 8.72 (d, 1H, J = 8.4 Hz), 8.55 (d, 1H, J = 7.2 Hz), 8.38 (d, 1H, J = 8.3 Hz), 7.89 (t, 1H, J = 7.8 Hz), 6.97 (d, 2H, J = 8.6 Hz), 6.85 (d, 1H, J = 8.3 Hz), 6.69 (d, 2H, J = 8.6 Hz), 5.76 (s, 2H), 5.21 (s, 2H); 13C NMR (DMSO-d6): 161.4, 160.9, 160.5, 147.4, 144.0, 133.5, 131.8, 128.9, 127.9, 127.2, 123.3, 122.2, 122.0, 115.4, 115.0, 109.7.
2.3.4 4-(4-Aminophenoxy)-N-(4-aminophenyl)-1,8-naphthalimide 4. 4-Bromo-N-(4-aminophenyl)-1,8-naphthalimide 2 (15.0120 g, 40.9 mmol), 4-aminophenol (8.9204 g, 81.7 mmol) and anhydrous potassium carbonate (11.2917 g, 81.7 mmol) were mixed in a 500 mL flask containing a mixture (200 mL) of acetonitrile and N,N-dimethylformamide (4
:
1). The reaction mixture was heated at 120 °C for 16 h. The solvents were evaporated from reaction mixture under reduced pressure and distilled water was added to obtain product. The crude product was filtered and washed with hot water to remove excess 4-aminophenol. The resulting pure brown precipitate of 4-(4-aminophenoxy)-N-(4-aminophenyl)-1,8-naphthalimide 4 (15.9013 g, 99%) were dried in oven at 80 °C for 24 h. Melting point: 265–266 °C. FT-IR (KBr): 3410, 3384, 3344, 3227, 1697, 1649, 1609, 1588, 1518, 1503, 1397, 1378, 1363, 1239, 1208, 784, 488 cm−1; 1H NMR (DMSO-d6): 8.73 (d, 1H, J = 8.3 Hz), 8.53 (d, 1H, J = 7.0 Hz), 8.37 (d, 1H, J = 8.2 Hz), 7.89 (t, 1H, J = 7.8 Hz), 6.98 (d, 2H, J = 8.3 Hz), 6.92 (d, 1H, J = 8.4 Hz), 6.86 (d, 2H, J = 8.5 Hz), 6.69 (d, 2H, J = 8.3 Hz), 6.63 (d, 2H, J = 8.2 Hz), 5.23 (s, 4H); 13C NMR (DMSO-d6): 164.5, 163.9, 161.0, 148.9, 147.3, 144.2, 133.4, 131.8, 129.7, 128.7, 127.2, 124.3, 123.4, 123.1, 122.1, 116.13, 115.4, 114.2, 109.8.
2.4. Polymer synthesis
2.4.1 Polymerization of 5b–e. Polyimides 5b–e were synthesized from reaction of monomer 3 with various aromatic dianhydrides by one-step polymerization in m-cresol in the presence of a catalytic amount of benzoic acid at 190 °C (Scheme 2). The synthetic procedure for polyimides 5e is employed as an example to illustrate the general synthetic route. Diamine 3 (1.2771 g, 4.0 mmol) was dissolved in m-cresol (13 mL) in a two-necked flask equipped with a nitrogen inlet and a distillation head. BPADA (2.0811 g, 4.0 mmol) and benzoic acid (0.2500 g, 2.0 mmol) were added to the diamine solution. Initially reaction mixture was heated at 100 °C for 2 h followed by 190 °C for 30 h. Water was continuously distilled off from the reaction mixture. Then the reaction mixture was cooled to room temperature and subsequently poured into ethanol (300 mL). The precipitated polymer was collected, filtered and washed with boiling ethanol. After drying under a high vacuum at 80 °C for 12 hours; polyimide 5e was obtained as a grey powder (3.0712 g, 96%). The inherent viscosity of 5e was 0.57 dL g−1 measured at a concentration of 0.5 g dL−1 in m-cresol at 30 °C. The polymer films were prepared from polyimide 5b–e solutions in m-cresol. A typical procedure for film casting was used as follows: the purified and dried polymer (0.8–1.0 g) was dissolved in 15–18 mL of m-cresol (some of the polymers were required to heat for better solubility). The solution was filtered to remove dust particles and casted on a levelled clean glass plate. The casting films were dried in an oven at 80 °C for 8 h, 150 °C 1 h, 200 °C 1 h, 250 °C 1 h and finally at 300 °C for 1 h. The film was removed from the glass by immersion in water and dried at 100 °C for 2 h.
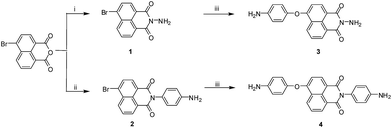 |
| Scheme 1 Synthesis of diamine monomers. Reagents: i, hydrazine, EtOH; ii, p-phenylenediamine, EtOH; iii, 4-aminophenol, K2CO3, CH3CN/DMF. | |
 |
| Scheme 2 Synthesis of polyimides from asymmetric diamines 3 and 4. | |
2.4.2 Polymerization of 6a–e. Polyimides 6a–e were synthesized from reaction of monomer 4 with various aromatic dianhydrides by two-step polymerization in NMP (Scheme 2). The synthetic procedure for polyimides 6e is employed as an example to illustrate the general synthetic route. Diamine 4 (1.5814 g, 4.0 mmol) and NMP (13 mL) were charged into a 50 mL two necked flask with magnetic stirring. After the diamine was dissolved, BPADA (2.0813, 4.0 mmol) and was added slowly. The mixture was stirred at room temperature for 24 h to afford a viscous solution of poly(amic acid) (PAA). The PAA solution was filtered to remove dust particles and the inherent viscosity of PAA was 0.96 dL g−1, measured at a concentration of 0.5 g dL−1 in NMP at 30 °C. The PAA solution was diluted to 10 wt%, casted on a levelled clean glass plate and the casting films were dried in an oven at 80 °C for 2 h, 150 °C 1 h, 200 °C 1 h, 250 °C 1 h and finally at 300 °C 1 h. The film was removed from the glass by immersion in water and dried at 100 °C for 2 h.
3. Results and discussion
3.1. Synthesis of monomers
4-Bromo-N-amino-1,8-naphthalimide 1 and 4-bromo-N-(4-aminophenyl)-1,8-naphthalimidewith 2 were prepared from 4-bromo-1,8-naphthalic anhydride in ethanol with high yield (95%) in the first step as shown in Scheme 1. Then the nucleophilic displacement of 1 and 2 with 4-aminophenol proceeded very smoothly in the presence of potassium carbonate as base to synthesize asymmetric diamines 3 and 4. Chemical structures of the targeted monomers were characterized with 1H NMR, FT-IR and 13C NMR spectroscopy.
The 1H NMR spectra of diamines 3 and 4 are shown in Fig. 2. In diamine 3, the amino group of attached to the naphthalimide moiety exhibited chemical shift at 5.75 ppm while amino attached to benzene ring on the other end of diamine monomer showed at 5.21 ppm (Fig. 2). In 1H NMR spectrum of 4, both amino groups showed chemical shift at 5.23 ppm owing to comparatively similar proton environment (Fig. 2). 13C NMR spectroscopy also supported with the structures of diamines 2 and 4 (Fig. S1†). The FT-IR spectrum of the diamines 3 and 4 showed characteristic C
O absorptions of naphthalimide ring at 1645–1720 cm−1 and 3316–3453 cm−1 of the amino (N–H) stretching vibrations (Fig. S2†). Spectroscopic results clearly supported the structure of diamines.
 |
| Fig. 2 1H NMR spectra of diamines 3 and 4 in DMSO-d6. | |
3.2. Synthesis of polyimides
Hay and co-workers reported that, N,N′-diamino-1,4,5,8-naphthalenetetracarboxylic bisimide which acted as symmetric diamine, was failed to synthesize polyimides by one and two-step polymerization methods, due to the insolubility of symmetric diamine in NMP at ambient temperature, and insolubility of oligomers which formed in the early stages of the polymerization reaction.23 However, diamines 3 and 4 have good solubility in NMP owing to their asymmetric structure. Polymerization of diamine 3 was performed with several aromatic dianhydrides by one-step polymerization method using m-cresol to achieve high molecular weight polyimides 5b–e, while two-step polymerization method failed due to poor reactivity of the amino group attached to the imide group in diamine 3. When PMDA was used, precipitation occurred due to poor solubility of polymer 5a. In comparison, the reactivity of the diamine 4 was sufficiently high enough to give high molecular weight polyimides 6a–e by conventional two-step polymerization. However, for diamine 4, one-step polymerization was failed due to the poor solubility of polyimides in m-cresol (solid weight content ∼20 wt%).
Hence, two different polymerization methods were employed on the basis of the difference in reactivity of diamines, and solubility of their corresponding polyimides. The inherent viscosities of polyimides 5b–e and polyamic acids of PIs 6a–e, were found in the range of 0.57–0.95 and 0.61–0.97 dL g−1 at 30 °C, respectively. All the polyimides could be cast into flexible and tough films.
The polyimides were characterized with 1H NMR, FT-IR and 13C NMR spectroscopy. In the 1H NMR spectrum of polyimide 5e, NH2 signal of diamine and amide proton were absent which showed successful imidization of polyimide (Fig. 3). In the FT-IR spectra of PI 5e and 6e, characteristic peaks of asymmetric C
O stretching of phthalimide and naphthalimide were observed at 1788, 1774 and 1770 cm−1 (Fig. 4). In the spectrum of PI 5e, peaks at 1733, 1720 and 1697 cm−1 were attributed to symmetric C
O stretching of the two phthalimides (hydrazine-based imide group and conventional imide group) and naphthalimide group, respectively. However, PI 6e derived from diamine 4, have similar environment around two phthalimide rings, and showed symmetric C
O stretching of phthalimides and naphthalimide at 1712 and 1667 cm−1, respectively. Imide group of polyimides also indicated peaks 1342 and 1357 cm−1 (C–N stretching) and 727 cm−1 (C
O bending).22,25 In addition, in the spectrum of 5e, the peak at 1180 cm−1 was assigned to the N–N stretching.
 |
| Fig. 3 1H NMR spectrum of polyimide 5e in CDCl3. | |
 |
| Fig. 4 FTIR spectra of polyimide 5e and 6e. | |
3.3. Solubility
The solubility behaviour of the polyimide is summarized in Table 1. The polyimides 5b–e based on diamine 3 showed improved solubility in m-cresol, NMP, N,N-dimethylacetamide (DMAc) and dimethyl sulfoxide (DMSO) as compared to 6a–e based on diamine 4. Polyimides based on rigid dianhydrides (PMDA, BPDA, BTDA) were relatively poor soluble in tested organic solvents. PI 5e based on BPADA with two flexible ether linkages and diamine 3, showed excellent solubility among all polyimides. In comparison, polyimide 6e was relatively less soluble. The better solubility of polyimides 5b–e was attributed to non-coplanar conformation of naphthalimide and phthalimide rings of bisimide unit (I), which were greatly twisted due to steric repulsion of the C
O groups (Fig. 5), broke up the conjunction along the backbone, and hindered the formation of intermolecular change–transfer complexes.22 In polyimides 6a–e, benzene ring between two imides rings of bisimide (III) polymer main chain not only maintained the steric repulsion of the carbonyl groups but also increased rigidity in polyimides. Such increased rigidity of polymer chain may be caused the comparative decrease in the solubility of PIs 6a–e. Moreover, the strong intermolecular π–π stacking interactions among naphthalimide moiety and aromatic rings may be also increased the chain packing.26 All of these polyimides derived from asymmetric diamines 3 and 4, exhibited better solubility than reported symmetric bis(N-amino naphthalimide).22 Asymmetric structure of diamine in polyimide main unit disturbed chain packing and intermolecular interactions of the polymer and increased the solubility.
Table 1 The inherent viscosity and solubility behavior of the polyimides
PI |
ηinha (dL g−1) |
Solventsb |
m-Cresol |
NMP |
DMAc |
DMSO |
THF |
CH3Cl |
The inherent viscosities of PIs (5b–e in m-cresol) and PAAs (6a–e in NMP) were measured at a concentration of 0.5 g dL−1 at 30 °C. ++ soluble at room temperature; + soluble on heating; ± partially soluble on heating; − insoluble on heating. THF: tetrahydrofuran. |
5b |
0.95 |
++ |
++ |
± |
+ |
− |
− |
5c |
0.84 |
++ |
++ |
± |
+ |
− |
− |
5d |
0.64 |
++ |
++ |
± |
+ |
− |
± |
5e |
0.57 |
++ |
++ |
++ |
+ |
± |
++ |
6a |
0.61 |
+ |
± |
− |
± |
− |
− |
6b |
0.88 |
+ |
± |
± |
− |
− |
− |
6c |
0.67 |
+ |
± |
− |
− |
− |
− |
6d |
0.97 |
++ |
± |
± |
± |
− |
− |
6e |
0.96 |
++ |
++ |
+ |
+ |
− |
− |
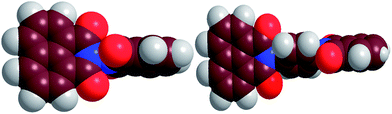 |
| Fig. 5 Energy minimized structures of asymmetric moiety (I) and (III). | |
3.4. Thermal and mechanical properties
Differential scanning calorimetry (DSC) and thermal gravimetric analysis (TGA) were performed to investigate the thermal properties of the polyimide. The Tg of the PIs, which is one of the key parameters of polymers when considering the high-temperature devices fabrication and optical applications, was determined by the DSC analysis. All studied PIs based on asymmetric diamine 3 exhibited high Tg (>311 °C) with no melting endotherms.
In comparison, PIs 6a–e based on diamine 4 showed higher Tg (>338 °C) and better thermal stability. PI 6b comprised of BPDA presented Tg at of 421 °C while in case of 6a, there was no Tg observed until 450 °C. Evidently, the increase in Tg of 6a–e was attributed to the more rigid and highly packed structure due to three aromatic rings (naphthalimide–benzene–phthalimide) unit in the polymer along with the non-coplanarity (Fig. 5). Moreover, the strong intermolecular π–π stacking interactions driven by naphthalimide moiety with aromatic rings may also cause the increase of Tg in the polyimides.26 DSC curves of PIs (5b, 5e, 6b, 6e) are represented in Fig. 6. In comparison, polyimides derived from symmetric bis(N-amino naphthalimide)s showed higher Tg (up to 438 °C) and comparable thermal stability to polyimides from asymmetric diamines (3 and 4).22–24 The 5% weight-loss temperature T5% values of PIs 5b–e were observed at 435–481 °C in nitrogen atmosphere, which were inferior to the thermal stability of PIs 6a–e derived from diamine 4 (Fig. 6) and may be attributed to the low bond energy of the N–N bond (the bond energy of the N–N bond is 159 kJ mol−1, whereas that of the C–C bond is 332 kJ mol−1).27
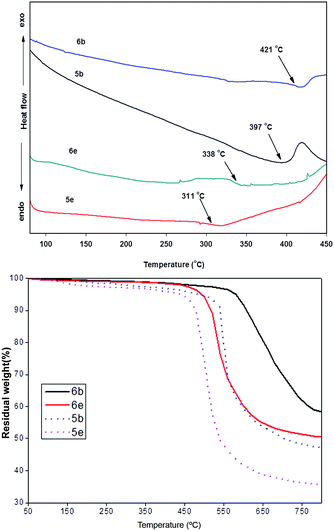 |
| Fig. 6 DSC and TGA curves of polyimides 5b, 5e, 6b and 6e in N2. | |
The limited oxygen index (LOI) is the minimum concentration of oxygen expressed as a percentage that will support combustion of a polymer – the higher the LOI, the lower the flammability. The LOI value can be used to evaluate the flame retardancy of polymeric materials. Since air comprises about 21% oxygen by volume, any material with a limiting oxygen index less than this will burn easily in air, while those with a higher LOI will not tend to burn.
LOI values of PIs 5b–e and 6a–e calculated from their char yield were found higher than 31.7 and 37.7, respectively (Table 2). On the basis of LOI values, these phthal-naphthalimide based polyimides can be classified as self-extinguishing polymers.
Table 2 Thermal properties of polyimide films
PI |
Tga (°C) |
T5%b (°C) |
Char yieldc (%) |
LOId |
Obtained from the DSC as baseline shift in the second heating scan at a heating rate of 20 °C min−1 in nitrogen. N.D.; not determined. Temperature at which 5% weight loss recorded by TGA at a heating rate of 10 °C min−1 in nitrogen. Residual weight retention at 800 °C. Limited oxygen index. |
5b |
397 |
481 |
47.0 |
36.3 |
5c |
368 |
472 |
44.1 |
35.2 |
5d |
327 |
461 |
43.9 |
35.0 |
5e |
311 |
435 |
35.5 |
31.7 |
6a |
N.D. |
563 |
53.0 |
38.6 |
6b |
421 |
537 |
58.3 |
40.8 |
6c |
390 |
501 |
56.1 |
39.9 |
6d |
377 |
494 |
56.6 |
40.1 |
6e |
338 |
476 |
50.5 |
37.7 |
The mechanical properties of the polyimides are tabulated in Table 3. All of the polyimides were able to cast as transparent, flexible, and tough film with good mechanical strength. PIs 6a–e demonstrated high tensile moduli of 2.8–4.9 GPa and tensile strengths of 107.3–172.2 MPa and elongations at break of 2.9–9.1%. In comparison, PIs 5b–e showed lower tensile moduli of 3.7–3.9 GPa, tensile strengths of 110.6–136.0 MPa and elongations at break of 4.9–6.0%. Polyimide 6a, based on rigid dianhydride PMDA and diamine 4, displayed highest tensile modulus (4.9 GPa), and PI 6e derived from BPADA with two flexible ether linkages and diamine 4, showed longest elongation at break (9.1%). However, PIs 5e exhibited lower elongation at break (6.0%). It was also noted that for the polyimides containing same diamine 4, the tensile strengths and tensile moduli decreased with increasing the flexibility of the dianhydride unit.
Table 3 Water uptake and mechanical properties of polyimide films
PI |
Initial modulus (GPa) |
Strength at break (MPa) |
Elongation (%) |
Water uptakea (%) |
Water uptake of polyimide films were measured immersing films of these polyimides in deionized water at 25 °C for 24 h. |
5b |
3.9 |
128.0 |
5.5 |
1.04 |
5c |
3.4 |
110.6 |
4.9 |
1.81 |
5d |
3.8 |
136.0 |
6.9 |
1.62 |
5e |
3.9 |
133.3 |
6.0 |
1.96 |
6a |
4.9 |
117.0 |
2.9 |
0.33 |
6b |
4.7 |
172.2 |
5.2 |
0.61 |
6c |
4.1 |
153.4 |
8.5 |
0.86 |
6d |
3.7 |
109.5 |
3.7 |
0.76 |
6e |
2.8 |
107.3 |
9.1 |
1.13 |
3.5. Wide-angle X-ray diffraction
The crystallinity and chain packing of the polyimide films were analyzed by wide-angle X-ray diffraction (WAXD). Regardless of the dianhydrides and diamines employed, the WAXD patterns of all the PI films were broad without obvious peak features, indicating the amorphous nature of PIs (Fig. 7). The amorphous nature of the polyimides could be attributed to the introduction of asymmetric diamine and bulky naphthalimide group in the polymer chain backbone, which resulted the poor intermolecular packing. The mean intermolecular distances (d-spacing) of these PIs could be calculated from the maxima of the broad diffraction peaks by Bragg's equation. PIs 5b–e exhibited the diffraction peak at 2θ = 17.2–19.9° with d-spacing of 4.8–5.2 Å, whereas PIs 6a–e demonstrated diffraction peak at 2θ = 17.3–24.2° with d-spacing of 3.7–5.1 Å.
 |
| Fig. 7 Wide-angle X-ray diffractograms of PI films. | |
On comparing the pattern for dianhydrides, it could be observed that the d-spacing are greater for less rigid dianhydrides such as BPADA, which offered loose chain packing and aggregation. Moreover, X-ray data indicated that polyimides 6a–e based on diamine 4 have relatively denser polymer chain packing than PIs 5b–e. WXRD result is consistent with other findings from Tg, solubility and mechanical properties.
3.6. Water uptake
The water uptake of polyimides might be related to several factors including the chemical structures, the introduction of functional groups, the geometrical packing of the polymer chains as well as film-processing parameters, and so forth in which the chemical structure and the presence of functional groups in polymer might be major concern factors.28 The bulky group could loosen the packing of the polyimide backbone chains, resulting in the increase of free volume in the polymers that should ensure the polymer to entrap some of the water molecules.28 The water uptake (WU) of PIs was determined in deionized water at 25 °C for 24 h (Table 3). Polyimides 5b–e with loosen chain packing showed water uptake in the range of 1.04–1.96% while PIs 6a–e with tight chain packing showed in the range of 0.33–1.13%. PI 5e and 6e based on BPADA with two ether kinks showed relatively high water uptake due to the increase of free volume of polymer and interaction of ether linkage with water molecules.
3.7. Optical properties
3.7.1 UV-vis absorption. The optical properties of the studied polyimides in solution state were analysed by UV-vis spectroscopy. The UV-visible spectra of diamines 3 and 4 were taken by dissolving in NMP (1 × 10−5 mol L−1). Diamine 3 exhibited absorption with the lowest energy absorption maximum at ca. 374 nm, while diamine 4 at 368 nm (Fig. S3†). It is apparent that the wavelength of maximum absorption is related to the π → π* transition resulting from the conjugation between the aromatic ring of donor group 4-aminophenylether to the bay position of naphthalene imide core. All polyimides 5b–e showed typical naphthalimide absorption peak at 371 nm in the UV-vis absorption spectra using NMP at dilute concentration (1 × 10−5 mol L−1) as shown in Fig. 8. In comparison, polyimide 6a–e showed shift of offset broad absorption bands to shorter wavelength (∼361 nm), which may be attributed to better chain packing as compared to 5b–e (Fig. 8).
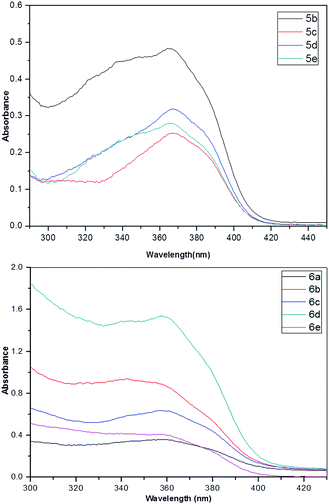 |
| Fig. 8 UV-Vis absorption spectra for polyimides 5b–e and 6a–e in NMP (1 × 10−5 mol L−1) at room temperature. | |
3.7.2 Photoluminescence (PL) study. It is believed that the development of emission color in 1,8-naphthalimides is dependent on the presence of a strong electron-donating substitute on the 4-position of the naphthalimide ring.26,29 When hydrogen is replaced by an electron-donating group, the fluorescent quantum yield is gradually increased and the emission wavelength is red-shifted from blue to green region.29 Polyimides films 6a–e showed broader emission spectra in the range of 480–650 nm with intense fluorescence at ∼576 nm. In contrast, polyimides 5b–e showed a broader emission range of 485–700 nm with intense fluorescent at ∼541 nm (Fig. 9). The nucleophilicity of the 4-aminophenylether moiety decreases from BPADA based PI to PMDA based PI and intensity of fluorescence increases in same pattern. The strong electron deficiency of the corresponding dianhydride moiety may be curtailed the electrophilicity of 4-amino moiety in the diamine. This change in fluorescence intensity among the PIs can also be attributed to the charge transfer (CT) interactions.29,30 CT may occur between the electron-donor (diamine) and electron-acceptor (dianhydride) fragments of the PIs. Polyimide 5e and 6e comprised of BPADA, the lesser denser molecular aggregation, showed the strongest fluorescent intensity, while polyimide 6a from PMDA is the weakest. This suggests that the CT fluorescence in polyimides 5b–e is sensitive to the orientation of local polymer structures.30
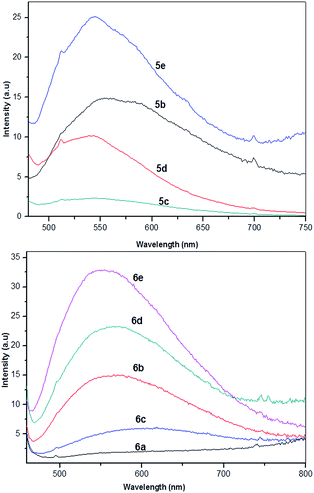 |
| Fig. 9 Fluorescence emission spectra of the polyimides 5b–e and 6a–e thin films at λex of 465 and 460 nm, respectively. | |
4. Conclusion
Two novel asymmetric diamines 3 and 4 were synthesized and employed to react with several aromatic anhydrides to yield two series of new polyimides 5b–e and 6a–e through one and two-step polymerization method. Both series of polyimides showed good solubility in various polar and nonpolar solvents due to asymmetric and non-coplanar bisimides linkages (I and III) in polyimide main chain. PIs 6a–e exhibited excellent thermal stability, high Tg values and good mechanical properties as compared to PIs 5b–e. Such improved properties were attributed to the more rigid and highly packed structure of bisimide moiety (III) in the polymer along with the non-coplanarity. The polymers also have strong fluorescence.
Acknowledgements
This work was financially supported by National Natural Science Foundation of China (Grant No. 51403225) and the program for Ningbo Municipal Science and Technology Innovative Research Team (Grant No. 2015B11002).
Notes and references
- D. J. Liaw, K. L. Wang, Y. C. Huang, K. R. Lee, J. Y. Lai and C. S. Ha, Prog. Polym. Sci., 2012, 37, 907–974 CrossRef CAS.
- K. Chen, X. Chen, K. Yaguchi, N. Endo, M. Higa and K. Okamoto, Polymer, 2009, 50, 510–518 CrossRef CAS.
- B. Kraftschik, W. J. Koros, J. R. Johnson and O. Karvan, J. Membr. Sci., 2013, 428, 608–619 CrossRef CAS.
- S. H. Hsiao and P. C. Huang, J. Polym. Res., 1997, 4, 183–190 CrossRef CAS.
- Y. Guan, D. Wang, Z. Wang, G. Dang, C. Chen, H. Zhou and X. Zhao, RSC Adv., 2014, 4, 50163–50170 RSC.
- Y. Shao, Y. Li, X. Zhao, T. Ma, C. Gong and F. Yang, Eur. Polym. J., 2007, 43, 4389–4397 CrossRef CAS.
- F. Yang, J. Zhao, Y. Li, S. Zhang, Y. Shao, H. Shao, T. Ma and C. Gong, Eur. Polym. J., 2009, 45, 2053–2059 CrossRef CAS.
- C. S. Wang and T. S. Leu, Polymer, 2000, 41, 3581–3591 CrossRef CAS.
- H. A. Kang, I. S. Chung, M. Kakimoto and S. Y. Kim, Polym. J., 2001, 33, 284–289 CrossRef CAS.
- S. Tamai, A. Yamaguchi and M. Ohta, Polymer, 1996, 37, 3683–3692 CrossRef CAS.
- C. P. Yang, S. H. Hsiao and K. L. Wu, Polymer, 2003, 44, 7067–7078 CrossRef CAS.
- S. H. Hsiao and K. H. Lin, J. Polym. Sci., Part A: Polym. Chem., 2005, 43, 331–341 CrossRef CAS.
- Z. Cheng, Q. Xia, X. Wang, J. Dong and Q. Zhang, High Perform. Polym., 2013, 25, 929–937 CrossRef.
- Q. Bu, S. Zhang, H. Li, Y. Li, C. Gong and F. Yang, Polym. Degrad. Stab., 2011, 96, 1911–1918 CrossRef CAS.
- S. H. Hsiao and K. H. Lin, Polymer, 2004, 45, 7877–7885 CrossRef CAS.
- C. H. Lin, S. L. Chang and P. W. Cheng, J. Polym. Sci., Part A: Polym. Chem., 2011, 49, 1331–1340 CrossRef CAS.
- C. L. Chung, W. F. Lee, C. H. Lin and S. H. Hsiao, J. Polym. Sci., Part A: Polym. Chem., 2009, 47, 1756–1770 CrossRef CAS.
- I. S. Chung and S. Y. Kim, Macromolecules, 2000, 33, 3190–3193 CrossRef CAS.
- W. Li, S. Zhang, G. Chen and Q. Zhang, Polymer, 2007, 48, 3082–3089 CrossRef CAS.
- H. B. Zheng and Z. Y. Wang, J. Polym. Sci., Part A: Polym. Chem., 1999, 37, 3227–3231 CrossRef CAS.
- J. Yan, Z. Wang, L. Gao and M. Ding, Macromolecules, 2006, 39, 7555–7560 CrossRef CAS.
- H. Ghassemi and A. S. Hay, Macromolecules, 1993, 26, 5824–5826 CrossRef CAS.
- H. Ghassemi and A. S. Hay, Macromolecules, 1994, 27, 3116–3118 CrossRef CAS.
- H. Ghassemi and A. S. Hay, Macromolecules, 1994, 27, 4410–4412 CrossRef CAS.
- J. Yan, Z. Wang, C. Lv, H. Yang, Z. Shang, L. Gao and M. Ding, J. Appl. Polym. Sci., 2008, 110, 706–711 CrossRef CAS.
- S. V. Bhosale, C. H. Jani and S. J. Langford, Chem. Soc. Rev., 2008, 37, 331–342 RSC.
- M. A. Bohn and T. M. Klapötke, Z. Naturforsch., B: J. Chem. Sci., 2004, 59, 148–152 CAS.
- K. L. Mittal, Polyimides and other high temperature polymers: synthesis, characterization and applications, CRC Press, 2009 Search PubMed.
- J. A. Gan, Q. L. Song, X. Y. Hou, K. Chen and H. Tian, J. Photochem. Photobiol., A, 2004, 162, 399–406 CrossRef CAS.
- S. C. Hsu, W. T. Whang and C. S. Chao, Thin Solid Films, 2007, 515, 6943–6948 CrossRef CAS.
Footnote |
† Electronic supplementary information (ESI) available. See DOI: 10.1039/c6ra00143b |
|
This journal is © The Royal Society of Chemistry 2016 |
Click here to see how this site uses Cookies. View our privacy policy here.