DOI:
10.1039/C6RA01937D
(Paper)
RSC Adv., 2016,
6, 46952-46965
Alginate–marine collagen–agarose composite hydrogels as matrices for biomimetic 3D cell spheroid formation
Received
22nd January 2016
, Accepted 16th April 2016
First published on 19th April 2016
Abstract
Hydrogels are prototypical matrices for 3D cell culture, of which alginate hydrogels are extensively used. However, ionic crosslinking agents, such as Ca2+, are required to form alginate hydrogels, but introduce Ca2+-associated cytotoxicity and long-term stability issues. Collagen is a promising biomaterial for 3D cell culture scaffolds primarily due to its biocompatibility. In the present study, the authors designed and fabricated a calcium-free, physically crosslinked, efficient, and bioactive hydrogel composed of alginate, marine collagen, and agarose (AmCA) for use in 3D cell cultures. This AmCA hydrogel was assessed by FTIR, swelling property, scanning electron microscopy, phase contrast microscopy, cell proliferation, cell viability, confocal microscopy, transparency and RT-PCR analyses. The gel was found to exhibit excellent cytocompatibility with various tumor and non-tumor cells, to generate high yields of multicellular spheroids, and to promote cellular activity. Furthermore, the transparency of the AmCA hydrogel suggests it can be used without cell-tracking chemicals in morphological studies of cell cultures. Taken together, it would appear that the described physically crosslinked AmCA hydrogel could provide a novel platform for the development of customizable, transparent, biocompatible, functional, easy-to-produce, and cost-effective scaffolds for use in 3D cultures of various cell types.
Introduction
Cell culture systems are fundamental and essential tools for a wide range of research and clinical in vitro studies in the fields of life science, biotechnology, and biomedical science. However, conventional two-dimensional (2D) cell cultures are poor surrogates of complex three-dimensional (3D) natural milieu. Specifically, a variety of critical biological phenomena, such as gene expression and cellular survival, proliferation, adhesion, migration, development, and differentiation, have been shown to be more closely duplicated by 3D culture systems.1 Accordingly, 3D cell cultures have been attracting considerable research attention.
Scaffolding supports cells in 3D cultures and in tissue engineering applications, and of the many scaffolds developed for 3D cell cultures, hydrogels have been demonstrated to be one of the more efficient matrices.2 Hydrogels are composed of polymers constructed from crosslinked 3D polymer networks, and thus, are able to hold substantial amounts of water without being dissolved. Consequently, the unique properties of hydrogels, which include, extracellular matrix (ECM)-like viscoelasticity, interstitial flow, and diffusive transport characteristics, mimic the native tissue microenvironment. These properties mean that hydrogels are being viewed for use in a variety of biomedical applications, such as scaffolding for 3D cell cultures, 3D bioprinting, cell transplant therapy, drug delivery, and tissue engineering.1,3–5 To this end, various types of hydrogels have been proposed to improve their mechanical and functional properties, such as composite hydrogels including nanocomposite hydrogels.6–8
Alginate is a natural anionic, hydrophilic polysaccharide, and is one of the most important and commonly used materials to produce hydrogels. The alginates are chain-forming heteropolysaccharides comprised of blocks of (1–4)-linked β-D-mannuronic acid and α-L-guluronic acid, and in nature, are the major structural components of the cell wall and the intercellular matrix of marine brown algae (Phaeophycota). Furthermore, alginates can be readily engineered to fabricate 3D scaffolding materials with a broad range of biomedical applications due to their biocompatibility and biodegradability.9,10 The most commonly used means of gelling alginate in aqueous solution is to use a divalent cationic crosslinking agent, such as Ca2+, and calcium chloride (CaCl2) provides a convenient means of supplying the Ca2+.10,11 However, this Ca2+ may be cytotoxic,12,13 and the long-term stability of Ca2+-crosslinked alginate hydrogels are limited under physiological conditions, because the Ca2+ can be replaced by Na+, which results in crosslinking loss. Consequently, an uncontrolled dissolution ensues due to the release of Ca2+ into the surrounding media, and concomitant swelling of the hydrogel due to the penetration of water molecules attracted to the Na+ molecules.9,14 Although various intermolecular crosslinking methods, such as photocrosslinking, have been proposed to overcome this problem,15 these methods have also been found to be limited by the cytotoxic effects of ultraviolet (UV) light, chemical photoinitiators, and photogenerated species.16,17
Agarose is a neutral polysaccharide derived from several species of red marine algae (Rhodophyceae). It is a linear polymer consisting of repeated units of alternating 1,3-linked β-D-galactose and 1,4-linked 3,6-anhydro-α-L-galactose, which gives agarose the ability to form strong gels at even low concentrations.18 Furthermore, agarose is a reversible gelling agent, that solidifies or liquefies on cooling or heating, respectively, and thus, no specific counterions or additives are required to cause gelation.19 Agarose is commonly used in biomedical research, particularly in the molecular biology field, because it is inexpensive and nontoxic, and has excellent gelling characteristics, large pores (an average pore size of 100–300 nm) for effective transport of molecules through the gel, and controllable stiffness for tuning the mechanical properties of the scaffold.19–23 Agarose hydrogels have been demonstrated to support cell proliferation and maintain cellular phenotypes in many in vitro 3D culture studies.24 However, a serious drawback of pure agarose gels is that they have extremely low cellular invasion ability.25 Interestingly, it has been shown that agarose–collagen composite hydrogels can support cellular invasion, thereby suggesting the potential benefits of composite hydrogels to overcome this limitation of the pure agarose gel.25
Collagen constitutes 25 to 35% of protein in the human body, and is one of the most frequently used scaffold biomaterials. Collagen is the major structural component of the ECM and readily forms hydrogels, which have been widely used in biomedical research.26 Furthermore, collagen can form 3D networks of woven fibers by self-aggregation and crosslinking, and these networks resist tensile stress in multiple directions and support cell growth.27 In addition, its biocompatibility and biodegradability make it a near ideal choice for pharmaceutical and biotechnological applications.26,27 However, its poor mechanical strength has greatly restricted its extensive use as a scaffold for 3D cell culture or tissue engineering, despite the efforts of researchers to improve its mechanical properties by using crosslinkers, such as glutaraldehyde, genipin, and carbodiimide, or by forming composites with different kinds of natural polymers.25,28 In addition, outbreaks of bovine spongiform encephalopathy (BSE), transmissible spongiform encephalopathy (TSE), and foot-and-mouth disease (FMD) have increased health concerns regarding the use of mammalian collagen and its by-products, and its purification is difficult and expensive.
Marine collagen (MC) derived from marine organisms such as fish, seaweeds, sponges, and jellyfish, offers advantages over mammalian collagen, as it can be easily extracted, is water-soluble and safe because it is free of the risks of animal diseases and pathogens like those mentioned above, and is available in quantity.29,30 Accordingly, MC is receiving considerable attention as a mammalian collagen substitute from biomedical researchers and the cosmetic, food, and nutraceutical industries. However, few studies have been performed on the use of MC as a source of hydrogel scaffolds for 3D cell culture.31
Having considered these inherent properties of alginate, agarose, and MC, we fabricated a novel alginate–marine collagen–agarose (AmCA) composite hydrogel scaffold construct for use in 3D cell cultures, and evaluated its characteristics and efficacy in 3D cell cultures of different cell types, including tumor and non-tumor cells.
Materials and methods
Cell line, culture maintenance, and materials
For the tumor cell lines, we used adherent, non-hematologic tumor cells (A2780 cells) and non-adherent, hematologic tumor cells (EL4 cells). A2780 cells (a human epithelial ovarian cancer cell line) were obtained from Sigma-Aldrich (St. Louis, MO, USA), and EL4 cells (TIB-39™; a mouse thymic T cell lymphoma cell line) were purchased from the American Type Culture Collection (ATCC, Rockville, MD, USA). Both cell types were cultured in a RPMI-1640 medium containing 10% fetal bovine serum (FBS), 100 IU mL−1 penicillin, and 100 μg mL−1 streptomycin (all from Gibco/Invitrogen Life Technologies, Grand Island, NY, USA) at 37 °C in a 5% CO2 incubator. Subconfluent cells were harvested with trypsin–EDTA and used for further experiments. Media were replaced every third day.
For the non-tumor cell line, mouse thymic epithelial cells (1308.1 cells) were kindly donated by Dr Barbara B. Knowles (The Jackson Laboratory, Bar Harbor, ME, USA). The cells were cultured in Dulbecco’s modified Eagle’s medium (DMEM) containing 10% FBS, 100 U mL−1 penicillin, and 100 μg mL−1 streptomycin (all from Gibco/Invitrogen Life Technologies) at 37 °C in a 5% CO2 incubator.
The biomaterials used for fabricating hydrogels were sodium alginate (Sigma-Aldrich), agarose (high melting agarose, Affymetrix, Cleveland, OH, USA), rat-tail type I collagen (Sigma-Aldrich), and MC extracted from fish scales (Geltech, Busan, Korea). CaCl2 was purchased from Sigma-Aldrich, phosphate-buffered saline (PBS) without CaCl2 and MgCl2 from Gibco/Invitrogen Life Technologies, 4,6-diamidino-2-phenylindole (DAPI) from Vector Laboratories (Burlingame, CA, USA), and fluorescein isothiocyanate (FITC)–phalloidin from Promega (Madison, WI, USA).
Synthesis of alginate, agarose and alginate–collagen–agarose hydrogels
To fabricate calcium alginate hydrogels, cell suspensions were added to 2% alginate stock solution (1
:
1 v/v) at room temperature to obtain an alginate solution with a final concentration of 1% and a cell density of 5 × 105 cells per mL. Cell-alginate suspensions were then vortexed briefly to completely encapsulate the cells in alginate and transferred to 24-well tissue culture plates. Afterwards, different concentrations (100, 200, or 300 mM) of CaCl2 prepared from an autoclaved 1 M CaCl2 stock solution were added to wells to crosslink the cell-alginate suspension. These calcium alginate hydrogels were then incubated for 10–20 min at room temperature, rinsed three times with complete media, and incubated at 37 °C in a 5% CO2 humidified incubator for the scheduled time.
To fabricate agarose gels, high melting agarose was dissolved completely in deionized water (30 mg mL−1) by heating on a hot plate with occasional stirring to produce a 3% (w/v) agarose stock solution. Cells (5 × 105 cells per mL) were suspended in different concentrations of agarose solution (0.5, 1, 1.5 or 2%) at 35–40 °C to avoid cell damage. After gelation, encapsulated cells were cultured for the specified time period.
A schematic diagram of AmCA hydrogel preparation is shown in Fig. 1. Initially, stock solutions were prepared. Sodium alginate was dissolved at 50 mg mL−1 in deionized water by constant stirring overnight at room temperature to prepare a 5% alginate stock solution and then autoclaved before use. MC was completely dissolved by vortexing in nuclease-free water at room temperature to make a 25% stock solution. High melting point agarose was added at 20 mg mL−1 to deionized water, heated on a hot plate, and stirred occasionally until completely dissolved to make a 2% agarose stock solution. To prepare 1 mL of AmCA solution, 437 (or 337) μL of cell solution, resuspended in the culture medium (5 × 105 cells per mL), was mixed with 200 μL of 5% sodium alginate solution in a 1.5 mL Eppendorf tube at room temperature. This solution was then combined with 300 (or 400) μL of 25% MC stock solution at room temperature to obtain 7.5% (or 10%) MC/1% alginate solutions containing cells. These cell suspensions were then blended carefully with 62.5 μL of 2% agarose solution at 35–40 °C to avoid cell damage. The final concentrations of agarose, MC, and alginate in the AmCA solution were 0.125%, 7.5% or 10%, and 1%, respectively.
 |
| Fig. 1 Schematic representation of AmCA hydrogel fabrication. (A) Preparation of the AmCA hydrogel solution by mixing, in order, sodium alginate solution, cells, MC solution, and agarose solution. (B) The steps used to fabricate AmCA hydrogel. (C) Schematic diagram showing how AmCA hydrogels are generated and spheroids are formed. | |
Alginate–rat collagen–agarose (ArCA) hydrogels were fabricated in a similar manner using rat collagen instead of MC. Briefly, rat collagen was completely dissolved by vortexing in 0.1% acetic acid solution at room temperature to produce a 0.5% stock solution. To prepare 100 μL of ArCA solution, 63.75 μL of cells suspended in culture medium (5 × 105 cells per mL) were mixed with 20 μL of 5% sodium alginate solution in a 1.5 mL Eppendorf tube at room temperature. This alginate–cell solution was then combined with 10 μL of 0.5% rat collagen stock solution at room temperature to obtain a 0.05% rat collagen–1% alginate solution containing cells. Subsequently, this suspension was blended carefully with 6.25 μL of 2% agarose solution at 35 to 40 °C to avoid cell damage. The final concentrations of agarose, rat collagen, and alginate in the ArCA solution were 0.125%, 0.05%, and 1%, respectively.
For the gelation of AmCA and ArCA hydrogel solutions containing cells, they were vortexed briefly, pipetted into 1 mL syringes, and incubated at 4 °C for 5–10 min (Fig. 1C). The gelled hydrogels were then transferred to the wells of 24-well plates containing 1 mL of culture medium and incubated at 37 °C for the desired time (Fig. 1C). Media were refreshed every 2 days. The compositions of the synthesized hydrogels in this study are shown in Table 1.
Table 1 Composition of the prepared hydrogels
Sample |
Agarose (% w/v) |
Alginate (% w/v) |
MC (% w/v) |
Rat collagen (% w/v) |
CaCl2 (mM) |
Alginate hydrogel crosslinked with 100 mM calcium chloride. Alginate hydrogel crosslinked with 200 mM calcium chloride. Alginate hydrogel crosslinked with 300 mM calcium chloride. AmCA hydrogel containing 7.5% MC. AmCA hydrogel containing 10% MC. ArCA hydrogel containing 0.05% rat collagen. |
0.5% agarose |
0.5 |
— |
— |
— |
— |
1.0% agarose |
1.0 |
— |
— |
— |
— |
1.5% agarose |
1.5 |
— |
— |
— |
— |
2.0% agarose |
2.0 |
— |
— |
— |
— |
Alginate-100a |
— |
1.0 |
— |
— |
100 |
Alginate-200b |
— |
1.0 |
— |
— |
200 |
Alginate-300c |
— |
1.0 |
— |
— |
300 |
AmCA-7.5d |
0.125 |
1.0 |
7.5 |
— |
— |
AmCA-10e |
0.125 |
1.0 |
10.0 |
— |
— |
ArCA-0.05f |
0.125 |
1.0 |
— |
0.05 |
— |
FTIR spectroscopy
Fourier transform infrared spectroscopy (FTIR) spectra of 1% alginate, 0.125% agarose, 10% MC, and AmCA containing 10% MC were obtained using an attenuated total reflectance (ATR) technique using a Spectrum GX FTIR spectrometer (Perkin-Elmer, Waltham, MA, USA) at a resolution of 4 cm−1 in the range 4000 to 600 cm−1.
Swelling test
To determine equilibrium-swelling ratios, the ability of the AmCA hydrogel to absorb water was measured by incubating a test specimen in 300 μL of phosphate buffer saline (PBS, pH 7.4) or 300 μL of deionized water at 37 °C for 10, 20, 30, 40, 50, 60, 90, 120, 180 and 360 min. Prior to testing AmCA hydrogels containing 7.5% or 10% MC were oven-dried overnight at 40 °C. Hydrogel samples were then transferred to plates containing PBS or deionized water. At the end of the experiment, excess water was carefully removed using soft, lint-free paper and the gels were weighed. Water uptake (%) was calculated using:
Degree of swelling (%) = [(Wb − Wa)/Wa] × 100% |
where Wa is the initial weight of the hydrogel sample, and Wb is the weight of the swollen hydrogel sample.
Scanning electron microscopy
The shape and surface morphology of the AmCA hydrogel constructs containing 7.5% or 10% MC with or without cells were examined by scanning electron microscopy (SEM; JSM-6490LV, JEOL, Tokyo, Japan, accelerating voltage of 15 kV). Samples were gold-coated using an ion-sputter (E-1010, Hitachi, Tokyo, Japan).
Cell proliferation assay
To compare the proliferation of the A2780 and EL4 tumor cell lines (hematologic and non-hematologic, respectively) and of 1308.1 non-tumor cells in AmCA hydrogels (prepared with 7.5% or 10% MC) with that in 2D, these cells seeded at 1 × 104 cells per well in 96-well plates were incubated for 1, 3, 5, 7 and 10 days. In addition, to compare cell proliferation in AmCA hydrogels versus that in conventional calcium alginate hydrogels, A2780 cells seeded at 5 × 105 cells per well in 96-well plates were cultured for 3 and 5 days in AmCA hydrogel containing 7.5% MC or in 1% alginate hydrogels crosslinked with different concentrations of CaCl2 (100, 200, or 300 mM). Cell proliferation was determined at predetermined time points using the colorimetric water soluble tetrazolium salt-1 (WST-1)-based cell proliferation assay (EZ-Cytox assay kit, Daeil Lab Service, Seoul, Korea). Absorbance of the formazan dye, generated by the reaction of dehydrogenase with WST-1 in metabolically active cells, was measured using a microplate reader (Tecan, Männedorf, Switzerland) at 450 nm according to the manufacturer’s instructions.
Morphology, cell growth, and spheroid forming efficiency
To examine the growth efficiency of cells and spheroids, A2780, EL4 and 1308.1 cells (1 × 105 cells per mL) were encapsulated in AmCA hydrogels containing 7.5% (for A2780 and 1308.1) or 10% (for EL4) MC, and cultured at 37 °C in a 5% CO2 humidified incubator for the desired time. Culture media were refreshed every 2 days. The morphology and cell growth pattern were recorded and the number and size of spheroids were measured at desired time points under a phase contrast microscope. Spheroid forming efficiency was defined as the percentage of seeded cells that give rise to spheroids, assuming that one spheroid is derived from a single cell.
Encapsulation efficiencies of AmCA hydrogels
To compare the encapsulation efficiencies in AmCA and ArCA gels containing different concentrations of MC, EL4 cells (5 × 105 cells per mL) were encapsulated in ArCA (1% alginate/0.05% rat collagen/0.125% agarose), and AmCA containing 1% alginate, 0.125% agarose, and different concentrations of MC (0.05, 0.1, 0.2, 0.5, 1, 5, 10 or 15%) hydrogels using a 1 mL syringe as described in Fig. 1A. After encapsulation, the hydrogels were transferred to new 24-well plates for 24 h. The number of non-encapsulated cells were counted by trypan blue dye exclusion and plotted as a bar graph.
Cell viability assay
To examine cell viability in the 3D AmCA hydrogels, whole gel samples were analyzed using a calcein AM/EthD-I Live/Dead Viability/Cytotoxicity kit (Molecular Probes, Eugene, OR, USA). Calcein AM specifically stains living cells via their intracellular esterase activity while EthD-I stains dead cells that have lost plasma membrane integrity. The reagents were diluted according to the manufacturer’s protocol and incubated for 15–20 min in a serum-free medium before exposure to fluorescent light. The viability of A2780, EL4, and 1308.1 cells in AmCA hydrogels containing 7.5% (for A2780 and 1308.1) or 10% (for EL4) MC was determined after 7 or 14 days of culture by counting live (green) and dead (red) cells. Fluorescence images were obtained immediately after staining using a confocal microscope (FV1000 IX81, Olympus, Tokyo, Japan).
F-actin staining and confocal laser scanning microscopy
For confocal laser scanning microscopic analysis, A2780, EL4 and 1308.1 cells (1 × 105 cells per mL) were encapsulated in AmCA hydrogels containing 7.5% (for A2780 and 1308.1) or 10% (for EL4) MC, and cultured at 37 °C in a 5% CO2 humidified incubator for 3, 5 or 7 days. Cultured cells in hydrogels were washed with PBS and then fixed with cold 4% paraformaldehyde in 0.1 M phosphate buffer for 20 min. The fixative was then removed by washing the hydrogels three times for 5 min with cold PBS followed by permeabilization with 0.1% Triton X-100 in PBS for 5 min. After washing with cold PBS, they were incubated with 1% bovine serum albumin (BSA, Sigma-Aldrich) for 1 h at room temperature. Excess solution was then removed and hydrogels were incubated for 1 h at room temperature with FITC–phalloidin (Promega, diluted 1
:
150), rinsed in cold PBS, and mounted on glass slides using Vectashield® containing DAPI (Vector Laboratories). Cell fluorescence was observed using a confocal laser scanning microscope (Olympus). To examine 3D spheroid structure, a stack of confocal images spanning whole spheroids was collected using a step size of 1 μm. After thresholding, z-stack images were used to generate reconstructed 3D projection images.
Transparency test
To compare the transparency of AmCA hydrogels with that of conventional calcium alginate hydrogels, encapsulated A2780 cells (5 × 105 cells per mL) were cultured in calcium alginate hydrogels fabricated by crosslinking 1% sodium alginate solution with 100, 200 or 300 mM of CaCl2, and AmCA hydrogels containing 7.5% MC for 30 min and 24 h. Light transmission of each hydrogel was measured in a 96-well cell culture plate at 620 nm using a multi-well microplate reader (Tecan). Higher absorbance values indicate lower hydrogel transparencies.
RT-PCR
The protocol used for RNA extraction was a modification of the RNeasy-mini kit (Qiagen Valencia, CA, USA) procedure. Briefly, AmCA hydrogels containing cells were diced in a mixture of 1.5 mL QG buffer (Qiagen) and 2 mL RLT buffer (Qiagen). Samples were kept at room temperature until complete dissolution, and then stored at −20 °C. After defrosting, samples were homogenized and supplemented with 2 mL 70% ethanol. Subsequent RNA purification steps were performed as described by the manufacturer, and RNA quantity and quality were assessed using a Nanodrop 2000 (ThermoFisher Scientific, Waltham, MA, USA).
First-strand cDNA was obtained by reverse transcription using 2 μg of total RNA. The reaction was conducted in 25 μL buffer containing 0.5 μg oligo(dT) 12–18 primer (Promega), 50 mM Tris–HCl (pH 8.3), 75 mM KCl, 3 mM MgCl2, 40 mM dithiothreitol, 0.5 mM deoxynucleotide triphosphate mixture (Promega), 10 U RNase inhibitor (Promega), and 200 U Moloney murine leukemia virus (MMLV) reverse transcriptase (Promega). After incubation at 37 °C for 60 min, the reaction was stopped by heating at 70 °C for 5 min. The cDNA obtained was used as a template for PCR amplification using the following gene-specific primers: ICAM-1, (F) 5′-GAGAGTGGACCCAACTGGAA-3′, (R) 5′-CTTTGGGATGGTAGCTGGAA-3′; IL-7, (F) 5′-GCCTGTCACATCATCTGAGTGCC-3′, (R) 5′-CAGGAGGCATCCAGGAACTTCTG-3′; GM-CSF, (F) 5′-GTCACCCGCCTTGGAAGCAT-3′, (R) 5′-ACAGTCCGTTTCCGGAGTTGG-3′; TARC, (F) 5′-TGCTTCTGGGGACTTTTCTG-3′, (R) 5′-TGGCCTTCTTCACATGTTTG-3′; and GAPDH (F) 5′-TGGAGAAACCTGCCAAGTATG-3′, (R) 5′-TTGTCATACCAGGAAATGAGC-3′. PCR amplification of cDNA was performed in an automated thermal cycler (PC 320, Astec, Osaka, Japan) in a final volume of 25 μL containing 4 μL cDNA solution, 20 mM Tris–HCl (pH 8.4), 50 mM KCl, 1.5 mM MgCl2, 0.1% Triton X-100, 0.2 mM deoxynucleotide triphosphate mixture (Promega), 0.5 pmol of each primer, and 5 U Taq DNA polymerase (Promega). Amplified products were analyzed by electrophoresis in 2% agarose gel and visualized by ethidium bromide staining under UV light. Band intensities of PCR products were measured using an image analysis program (MetaMorph, Universal Imaging Corporation, Downingtown, PA, USA). Results were expressed as ratios versus GAPDH mRNA amplified from the same cDNA samples.
Statistical analysis
Results were expressed as means ± SD. The analysis was performed using the two-tailed student’s t-test. Statistical significance was accepted for p values < 0.05.
Results and discussion
FTIR analysis
FTIR analysis was used to analyze peak shifts caused by the interactions between blended materials. Fig. 2 shows the FTIR-ATR spectra of pure components and blends. The FTIR spectrum of MC showed characteristic absorption bands at 3286, 1634, and 1543 cm−1, corresponding to amide A, I, and II bands, representing N–H stretch, C
O stretch, and 60% N–H stretch plus 40% C–N stretch, respectively.32 The FTIR spectrum of alginate showed distinctive peaks at 3279 cm−1 (–OH stretch), 1603 and 1407 cm−1 (C
O asymmetric and symmetric stretch), and 1082 and 1016 cm−1 (C–O–C stretch).33 The FTIR spectrum of agarose displayed typical bands at 3285 cm−1 (–OH stretch), 1652 cm−1 (amide I band, representing C
O stretch) and 1071 cm−1 (glycosidic bonding).34 The FTIR spectrum of the AmCA hydrogel exhibited the peaks characteristic of parent molecules, and no modification-associated additional peaks (Fig. 2A).
 |
| Fig. 2 Physical characterization of the AmCA hydrogel. (A) FTIR spectra of 10% MC, 1% alginate, 0.125% agarose, and AmCA hydrogel containing 10% MC (AmCA-10). (B) SEM images of AmCA hydrogels without cells containing 7.5% (AmCA-7.5) or 10% (AmCA-10) MC showing pore size distributions. Original magnifications 100× (a and c), 300× (b and d). (C) Swelling kinetic curves of AmCA hydrogels in water and PBS. Swelling ratios of AmCA hydrogel containing 7.5% (a) or 10% (b) MC. Images of AmCA hydrogels in PBS at different exposure times (c). | |
This finding of no additional bonding between component polymers as determined by FTIR concurred with that of a previous study, in which a collagen–chitosan biopolymer composite was fabricated by freeze-drying, a physical crosslinking method.35
Microporous structure of AmCA hydrogels
SEM was performed to observe the ultrastructural features of AmCA hydrogels. Images of AmCA hydrogels exhibited distinct, highly interconnected pores of almost uniform size (Fig. 2B). The average pore sizes of AmCA hydrogels containing 7.5% and 10% MC were 66.6 ± 10 and 62 ± 8 μm, respectively. The interconnected porous structure of AmCA hydrogels resembled that of other natural polymer hydrogels, in which pores were produced by ice crystals.36
Swelling behavior
The ability of hydrogels to absorb aqueous solutions is critical, because this determines the ability of hydrogels to transfer nutrients, oxygen, and waste, avoid maceration, and maintain a moist environment.37 The swelling behavior of AmCA hydrogels was examined by measuring water uptake ratios. The water uptake ratios of these hydrogels were between 184% and 312% at 120 min after incubation, and the water uptake ratio of AmCA hydrogels containing 7.5% MC (152–312%) was greater than that of AmCA hydrogels containing 10% MC (55–187%) (Fig. 2C). These results show that AmCA hydrogels containing 7.5% or 10% MC absorb large quantities of water.38–40
Cell proliferation
To quantify the ability of AmCA hydrogels to facilitate cell proliferation, we used a WST-1 based colorimetric cell proliferation assay. A2780, EL4 and 1308.1 cells were successfully expanded and propagated in 3D AmCA hydrogels. The cell number in 2D cultures was significantly greater than that in 3D cultures for all three cell types during the first 3 days of culture (Fig. 3A–C). However, after 5 days of culture, numbers of A2780 and EL4 cells in 3D cultures exceeded those in 2D cultures, whereas the number of 1308.1 cells remained lower in 3D cultures (Fig. 3A–C). On day 7, all three cell types showed higher levels of proliferation in 3D than in 2D cultures, by 325.5% for A2780 cells, 467.4% for EL4 cells, and 155.3% for 1308.1 cells, and after 10 days their percentage increases versus 2D culture were even greater, by 907.3% for A2780 cells, 890.9% for EL4 cells, and 691.9% for 1308.1 cells (Fig. 3A–C). In fact, 2D cultured cells on day 10 showed a significant reduction in number, by 39.1% for A2780 cells, 50.7% for EL4 cells, and 45.0% for 1308.1 cells compared to those on day 1, whereas 3D cultured cells on day 10 showed a robust increase in number, by 804.7% for A2780 cells, 810.8% for EL4 cells, and 840.6% for 1308.1 cells relative to those on day 1 (Fig. 3A–C).
 |
| Fig. 3 Proliferation of (A) A2780, (B) EL4 and (C) 1308.1 cells on standard plastic tissue culture plates as compared with that in 3D AmCA hydrogels. Cells were imaged (left) and cell numbers were determined using a WST-1 assay (right) after culture for 1, 3, 5, 7 or 10 days. (D) Proliferation of A2780 cells in AmCA hydrogels were compared with that in 3D alginate hydrogels crosslinked with different concentrations of CaCl2 (100, 200, and 300 mM) after 3 or 5 days of cell culture. Results are presented as the means ± SD of three independent experiments. **p < 0.01 and ***p < 0.001 vs. the control. | |
Cells in culture usually grow according to a standard growth pattern. After seeding cells, growth proceeds from the lag phase, a period of slow growth, adaptation to the culture environment and preparation for fast growth, to the log phase, a period of rapid growth, exponential proliferation and nutrient consumption in the growth medium. Cells reach confluency at the end of the log phase due to nutrient depletion or lack of space; this final phase of growth is also referred to as the plateau or stationary phase, where the proliferation is greatly reduced or ceases. Overall, our results demonstrate that all the three cell types are slow-growing at an earlier stage, but grow much faster at a later stage in 3D culture than in 2D culture with some cell type-dependent variation (Fig. 3A–C). These results indicate that 3D cell culture using AmCA hydrogels substantially extends the log phase and delays the plateau phase onset as compared with 2D culture by providing a greater surface area to support cell attachment, colonization, and proliferation. Ideal 3D scaffold materials should create an environment suitable for cell proliferation. The present study shows that AmCA hydrogels provide support for cell survival and growth over extended times by producing an environment that facilitates the diffusion of oxygen, nutrients, metabolites, and soluble factors.
To compare the abilities of AmCA hydrogels and conventional calcium alginate hydrogels to facilitate cell growth, we measured cell proliferation using the WST-1 assay. At day 3, cells in calcium alginate hydrogels showed lower levels of proliferation than those cultured in AmCA hydrogels (by 42.76, 43.63, and 53.01% for 100, 200, and 300 mM of calcium chloride, respectively; Fig. 3D), and on day 5, calcium alginate hydrogel levels remained lower (by 12.36, 43.66, and 55.36%, respectively; Fig. 3D). These findings indicate that AmCA hydrogels provide environmental conditions that are more favorable for cell growth than calcium alginate hydrogels. The effects of MC on cell proliferation have not been examined, although its biocompatibility has been suggested.41–44 Of note, we previously reported that numbers of cells grown on MC-based nanofibrous scaffolds were significantly higher than on nanofibrous control scaffolds not containing MC.45 These results suggest that the presence of MC in AmCA hydrogels increased the proliferation of A2780, EL4 and 1308.1 cells, and provided an insight of the future use of MC as a scaffold material in regenerative medicine.29,46
Growth of cell spheroids in AmCA hydrogels
To evaluate the effects of AmCA hydrogels on the formation and growth of multicellular spheroids, we seeded A2780, EL4, or 1308.1 cells at 1 × 105 cells per mL into these hydrogels, and they were cultured for a desired time interval. All three cell types started to form multiple spheroids from day 3, and spheroid numbers then increased to peak on day 14. Spheroid sizes were measured at various time points. Fig. 4A shows phase contrast microscopy images of A2780 ovarian cancer cell spheroids formed in AmCA hydrogels after 14 days of culture. When A2780 spheroid sizes were measured on culture days 3, 5, 7, 10, and 14 under a phase contrast microscope, average spheroid diameters were 24.5 ± 7, 58.75 ± 12, 95.25 ± 12, 148 ± 37, and 211.75 ± 16 μm, respectively (Fig. 4B). These results show that 3D AmCA hydrogels can be used for the growth of A2780 cell spheroids at least for 2 weeks. In addition, the average spheroid diameter on day 14 was ∼250 μm, which is comparable to the size commonly used for spheroid-based drug-screening.47 The number of A2780 spheroids generated during 8 and 21 days in AmCA hydrogels was 41 ± 5 and 70 ± 5 spheroids for an initial seeding of 80 cells/80 μL (Fig. 4C). This observation indicates that the spheroid forming efficiency of AmCA hydrogels for A2780 cells is very high (51.3% and 87.5% on day 8 and day 21, respectively, Fig. 4C). We also analyzed the 3D morphology of A2780 spheroids formed in AmCA hydrogels. As shown in Fig. 4D, confocal micrographs of the nuclei and actin cytoskeleton staining of A2780 cells cultured in AmCA hydrogels for 3, 5 and 7 days showed well-organized structures of multicellular spheroids with smooth outlines and cells tightly attached to each other. The z-stack and reconstructed 3D projection images of spheroids in conjunction with the above-mentioned findings indicate AmCA hydrogels provided favorable 3D microenvironments for A2780 cells (Fig. 4D).
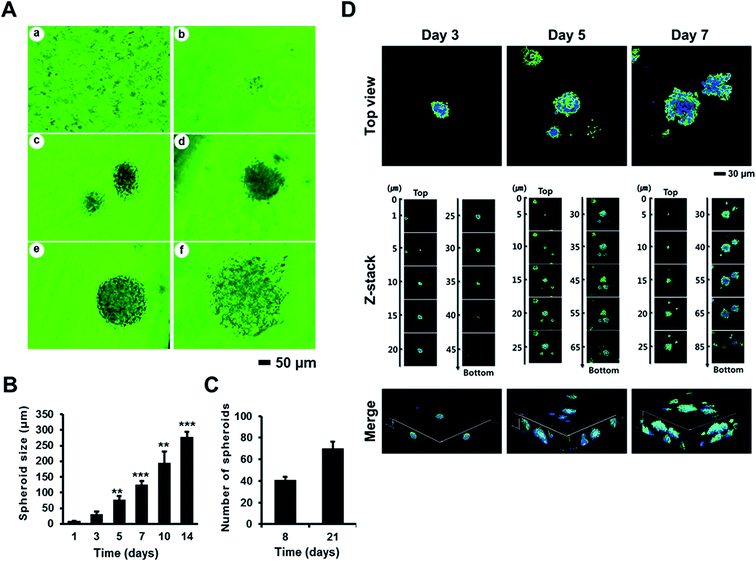 |
| Fig. 4 Growth of A2780 cell spheroids in AmCA hydrogels containing 7.5% MC. (A) Phase contrast microscopy images showing A2780 cell spheroids on culture days 1, 3, 5, 7, 10 and 14. (B) Sizes of A2780 cell spheroids depicted as a bar graph. (C) Numbers of A2780 spheroids at 8 and 21 days after cell seeding. (D) Morphology and distribution of A2780 spheroids in AmCA hydrogel containing 7.5% MC as determined by confocal microscopy on culture days 3, 5 and 7. Cellular actin and nuclei staining. Top views show actin and DAPI staining for cell spheroids (top row). Confocal z-stack sectioning of cell spheroids (middle row). A2780 spheroids were distributed throughout 3D hydrogels (bottom row). Results are presented as the means ± SD of three independent experiments. **p < 0.01, and ***p < 0.001 vs. the value on culture day 3. | |
We also measured the sizes of spheroids formed by EL4 (Fig. 5) and 1308.1 (Fig. 6) cells after 3, 5, 7, 10, and 14 days of culture in AmCA hydrogels under a phase contrast microscope. Average spheroid diameters observed for EL4 cells were 42.5 ± 10, 83.3 ± 7, 112.5 ± 12, 213.7 ± 35, and 316.9 ± 28 μm, respectively (Fig. 5), and for 1308.1 cells these were 39.1 ± 4, 85.9 ± 11, 146.6 ± 10, 218.6 ± 44, and 325.3 ± 29 μm, respectively (Fig. 6). These spheroid sizes were similar to those of A2780 cells as observed by confocal microscopy. In addition, 1308.1 cells were seeded at different cell densities into AmCA hydrogels to monitor spheroid growth. After 14 days in a culture of 1308.1 cells at seeding densities of 1 × 105, 2 × 105 and 5 × 105 cells per mL, the measured spheroid diameters were 367.2 ± 15, 262 ± 8, and 204.0 ± 7 μm, respectively (Fig. 6). These results indicate that spheroid size can be fine-tuned by optimizing culture parameters such as duration and cell seeding density.
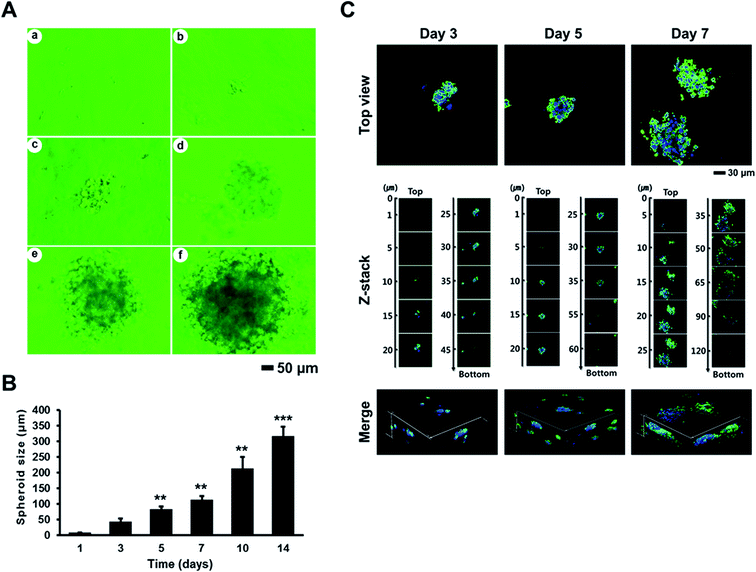 |
| Fig. 5 Growth of EL4 cell spheroids in AmCA hydrogels containing 10% MC. (A) Phase contrast microscopy images showing EL4 cell spheroids on culture days 1, 3, 5, 7, 10 and 14. (B) Sizes of the EL4 spheroids depicted as a bar graph. (C) Morphology and distribution of EL4 spheroids in AmCA hydrogel containing 10% MC observed by confocal microscopy on culture days 3, 5 and 7. Cellular actin and nuclei staining. Top views show the results of actin and DAPI staining for EL4 spheroids (top row). Confocal z-stack sectioning of 3D cultured EL4 cells (middle row). EL4 spheroids were distributed throughout the 3D hydrogels (bottom row). Results are presented as the means ± SD of three independent experiments. **p < 0.01, and ***p < 0.001 vs. culture day 3. | |
 |
| Fig. 6 Growth of 1308.1 cell spheroids in AmCA hydrogels containing 7.5% MC. (A) Phase contrast microscopy images showing 1308.1 cell spheroids on culture days 1, 3, 5, 7, 10 and 14. (B) Sizes of 1308.1 cell spheroids as depicted by a bar graph. (C) Phase contrast microscopy images showing 1308.1 spheroids seeded at different densities in AmCA hydrogel containing 7.5% MC on culture day 7 (left). Sizes of 1308.1 cell spheroids depicted as a bar graph (right). (D) Morphology and distribution of 1308.1 spheroids in AmCA hydrogel containing 7.5% MC as visualized by confocal microscopy on culture days 3, 5 and 7. Cellular actin and nuclei staining. Top views show actin and DAPI staining for 1308.1 spheroids (top row). Confocal z-stack sectioning of cell spheroids (middle row). Cell spheroids were distributed throughout 3D hydrogels (bottom row). Results are presented as the means ± SD of three independent experiments. **p < 0.01, and ***p < 0.001 vs. culture day 3. | |
Next, we evaluated cell growth and the formation of multicellular aggregates in AmCA and pure agarose hydrogels using EL4 cells. The cells cultured in AmCA hydrogels containing 10% MC showed significantly greater cell proliferation and spheroid formation than those in agarose gels of different concentrations (0.5, 1, 1.5, or 2%) after culture for 5 and 10 days (Fig. 7A). Fig. 7B shows SEM images of well-formed A2780, EL4, and 1308.1 spheroids in AmCA hydrogel matrices containing 7.5% or 10% MC after 8 (for A2780 and EL4 cells) and 16 (for 1308.1 cells) days of culture, indicating these hydrogels provide a suitable environment for cell growth and spheroid-formation.
 |
| Fig. 7 (A) Phase contrast microscopy images of agarose and AmCA hydrogels. EL4 cells were grown in AmCA hydrogels containing 10% MC and in pure agarose gels of different agarose concentrations for 5 or 10 days. (B) SEM images of well-formed spheroids in AmCA hydrogel after 8 (a–d) and 16 (e and f) days of culture. (C) The efficiency of EL4 cell encapsulation by AmCA hydrogels containing different MC concentrations and by the ArCA hydrogel. Phase contrast microscopy image showing encapsulated (arrowheads) and non-encapsulated (arrows) cells (left). The numbers of non-encapsulated EL4 cells in ArCA containing 0.05% rat collagen, and in AmCA containing different concentrations of MC are depicted as a bar graph (right). Results are presented as the means ± SD of three independent experiments. *p < 0.05, **p < 0.01, and ***p < 0.001 vs. the ArCA control. (D) Viability of A2780, EL4 and 1308.1 cells in AmCA hydrogels after 7 or 14 days of culture. Live cells are stained with calcein AM (green) and dead cells with ethidium homodimer-1 (EthD-1, red). | |
We seeded EL4 cells at 5 × 104 cells per gel in the ArCA hydrogel containing 0.05% rat collagen and in AmCA hydrogels containing MC at 0.05%, 0.1%, 0.2%, 0.5%, 1%, 2%, 5%, or 10%, and then cultured cells for 24 h. AmCA hydrogels at MC concentrations between 5 and 10%, and ArCA hydrogel containing 0.05% rat collagen were found to have a high encapsulation efficiency for EL4 cells (Fig. 7C). Furthermore, MC was found to concentration-dependently increase cell encapsulation efficiency, and AmCA hydrogels containing 10% MC were found to be optimal for the culture of EL4 cells in terms of the numbers of encapsulated cells observed, which suggests that MC contributes substantially to hydrogel cytocompatibility (Fig. 7C).
It is well known that 3D cultures of multicellular spheroids better mimic in vivo milieu than 2D cultures in terms of the expressions of genes associated with cell survival, proliferation, differentiation and treatment resistance.48,49 In particular, tumor spheroids more closely resemble avascular tumors containing layers of proliferating and quiescent cells.50,51 Consequently, the multicellular spheroid model has gained momentum as a tool for the establishment of efficient in vitro drug testing systems, and for reducing reliance on animal testing.
Viability of cells cultured in AmCA hydrogels
The status of A2780, EL4 and 1308.1 cells cultured in the AmCA hydrogels was investigated using a live–dead assay over a given time period. Live–dead staining revealed that most cells were alive after culture for 7 and 14 days, which may be attributed to the highly porous nature of the AmCA hydrogel (Fig. 7D). It was shown that viability of cells encapsulated in hydrogels is greatly affected by the viscosity of hydrogel solution.52,53 Thus, our results suggest AmCA hydrogels maintain cells in the long-term and that they are probably compatible with many other cell types.
Transparency of AmCA hydrogel
A2780 cells in a AmCA hydrogel containing 7.5% MC were seeded at a density of 5 × 104 cells per gel in 96-well plates. It was found that cells in the hydrogel were clearly observable by phase contrast microscopy (Fig. 8A). In fact, cells were imaged at a quality comparable to that obtained in 2D culture. In contrast, calcium alginate hydrogels crosslinked with CaCl2 solution (100, 200 and 300 mM) were opaque and cells were not visible (Fig. 8A). Light transmission measurements supported the markedly greater transparency of AmCA hydrogels (Fig. 8B and C). This result is in-line with that of a previous study in which alginate hydrogels crosslinked with calcium were found to be optically opaque,54 which supports our use of agarose-based physical crosslinking at temperatures that do not damage cells in designing AmCA hydrogels. This transparency of AmCA hydrogels could be used for the monitoring of living cells deep inside hydrogels by optical microscopy, and for biomedical applications, such as 3D bioprinting, to obtain cell images after staining with fluorescent dyes or fluorescent-labeled antibodies.55
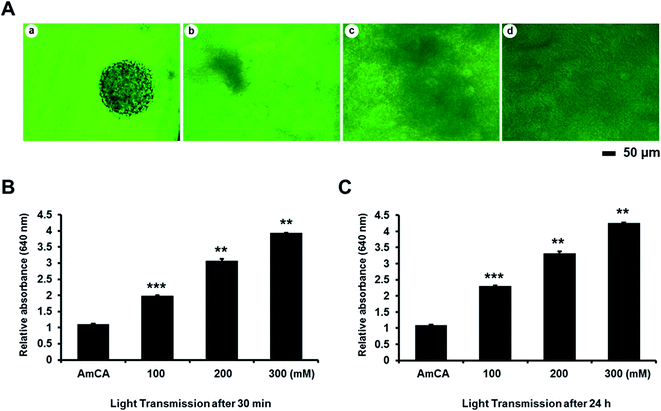 |
| Fig. 8 Phase contrast microscopy images showing the transparency of major types of 3D culture scaffolds. (A) Morphology of A2780 spheroids on culture day 2 after being grown in AmCA hydrogel containing 7.5% MC (a) or in calcium alginate hydrogels crosslinked with 100 mM (b), 200 mM (c), or 300 mM (d) CaCl2. Original magnification 200×. Light transmission measurements through AmCA and calcium alginate hydrogels after incubation for (B) 30 min and (C) 24 h. **p < 0.01, and ***p < 0.001 vs. AmCA hydrogel. | |
AmCA hydrogel culture stimulates cell function
To determine whether AmCA hydrogels promote cell functions, we investigated the expression of functionally important genes using 1308.1 cells, in which thymopoietic genes are crucial for cellular function. Total RNAs from 1308.1 cells cultured in AmCA hydrogel containing 7.5% MC were extracted using the RNeasy-mini kit (Qiagen), and the expression of thymopoietic genes, that is, ICAM-1, IL-7, TARC, and GM-CSF, was measured by RT-PCR. Furthermore, it has been well documented that thymopoiesis-related gene expression is enhanced when the functional activity of thymic epithelial cells is enhanced as during T cell development and regeneration.56 In the present study, the expressions of ICAM-1, IL-7, TARC and GM-CSF mRNA were found to be more upregulated in 3D than in 2D cultures (Fig. 9), which agrees with other reports on 3D based systems.57
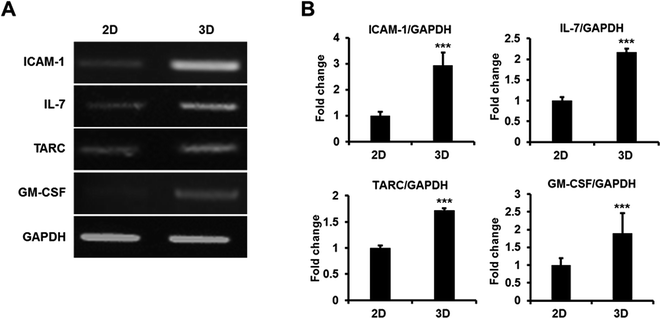 |
| Fig. 9 AmCA hydrogel enhanced thymopoietic gene expression in 1308.1 cells. (A) RT-PCR demonstrated enhanced expressions of thymopoietic genes. (B) Densitometry results are expressed as ratios of ICAM-1, IL-7, TARC and GM-CSF mRNA normalized vs. GAPDH mRNA. ***p < 0.001 vs. 2D control. | |
Conclusion
Greater effort is now being expended to develop physically crosslinked hydrogels to avoid using chemical crosslinking agents since they may have potential cellular toxicity and adversely affect hydrogel integrity. Moreover, the unreacted residues must be removed from gels prior to use. In summary, the present study shows that AmCA hydrogels offer a cytocompatible, transparent, biofunctional, easy-to-produce, customizable, and cost-effective 3D spheroid culture platform option. We believe our results have wide application in biomedical research and technology fields such as effective 3D cell culture of various normal and pathological cells, drug screening, and regenerative medicine applications.
Acknowledgements
This work was supported by the Pioneer Research Center Program of the Korean National Research Foundation funded by the Ministry of Science, ICT & Future Planning (Grant no. 201210130003).
References
- R. Edmondson, J. J. Broglie, A. F. Adcock and L. Yang, Assay Drug Dev. Technol., 2014, 12, 207–218 CrossRef CAS PubMed
. - M. W. Tibbitt and K. S. Anseth, Biotechnol. Bioeng., 2009, 103, 655–663 CrossRef CAS PubMed
. - S. W. Cho, I. K. Kim, S. H. Bhang, B. Joung, Y. J. Kim, K. J. Yoo, Y. S. Yang, C. Y. Choi and B. S. Kim, Eur. J. Heart Failure, 2007, 9, 974–985 CrossRef CAS PubMed
. - C. C. Lin and K. S. Anseth, Pharm. Res., 2009, 26, 631–643 CrossRef CAS PubMed
. - Y. Loo, A. Lakshmanan, M. Ni, L. L. Toh, S. Wang and C. A. Hauser, Nano Lett., 2015, 15, 6919–6925 CrossRef CAS PubMed
. - P. Fu, K. Xu, H. Song, G. Chen, J. Yang and Y. Niu, J. Mater. Chem., 2010, 20, 3869–3876 RSC
. - Z. Hu and G. Chen, Adv. Mater., 2014, 26, 5950–5956 CrossRef CAS PubMed
. - Z. Hu and G. Chen, J. Mater. Chem. A, 2014, 2, 13593–13601 CAS
. - K. Y. Lee and D. J. Mooney, Prog. Polym. Sci., 2012, 37, 106–126 CrossRef CAS PubMed
. - J. Sun and H. Tan, Materials, 2013, 6, 1285–1309 CrossRef CAS
. - C. K. Kuo and P. X. Ma, Biomaterials, 2001, 22, 511–521 CrossRef CAS PubMed
. - M. Buset, M. Lipkin, S. Winawer, S. Swaroop and E. Friedman, Cancer Res., 1986, 46, 5426–5430 CAS
. - N. Cao, X. B. Chen and D. J. Schreyer, ISRN Chem. Eng., 2012, 2012, 516461 Search PubMed
. - O. Jeon, C. Powell, S. M. Ahmed and E. Alsberg, Tissue Eng., Part A, 2010, 16, 2915–2925 CrossRef CAS PubMed
. - K. Y. Lee, E. Alsberg and D. J. Mooney, J. Biomed. Mater. Res., 2001, 56, 228–233 CrossRef CAS PubMed
. - N. E. Fedorovich, M. H. Oudshoorn, D. van Geemen, W. E. Hennink, J. Alblas and W. J. Dhert, Biomaterials, 2009, 30, 344–353 CrossRef CAS PubMed
. - O. Jeon, K. H. Bouhadir, J. M. Mansour and E. Alsberg, Biomaterials, 2009, 30, 2724–2734 CrossRef CAS PubMed
. - S. R. Van-Tomme, G. Storm and W. E. Hennink, Int. J. Pharm., 2008, 355, 1–18 CrossRef CAS PubMed
. - V. Normand, D. L. Lootens, E. Amici, K. P. Plucknett and P. Aymard, Biomacromolecules, 2000, 1, 730–738 CrossRef CAS PubMed
. - G. A. Griess, E. T. Moreno, R. A. Easom and P. Serwer, Biopolymers, 1989, 28, 1475–1484 CrossRef CAS PubMed
. - I. Gadjanski, S. Yodmuang, K. Spiller, S. Bhumiratana and G. Vunjak-Novakovic, Tissue Eng., Part A, 2013, 19, 2188–2200 CrossRef CAS PubMed
. - T. Marras-Marquez, J. Peña and M. D. Veiga-Ochoa, Carbohydr. Polym., 2014, 103, 359–368 CrossRef CAS PubMed
. - G. Xu, F. Yin, H. Wu, X. Hu, L. Zheng and J. Zhao, J. Tissue Eng., 2014, 5, 2041731413520438 Search PubMed
. - M. Ahearne and D. J. Kelly, Biomed. Mater., 2013, 8, 035004 CrossRef PubMed
. - T. A. Ulrich, A. Jain, K. Tanner, J. L. MacKay and S. Kumar, Biomaterials, 2010, 31, 1875–1884 CrossRef CAS PubMed
. - C. H. Lee, A. Singla and Y. Lee, Int. J. Pharm., 2001, 221, 1–22 CrossRef CAS PubMed
. - S. Gorgieva and V. Kokol, in Biomaterials Applications for Nanomedicine, ed. R. Pignatello, InTech, Rijeka, 2011, ch. 2, pp. 17–52 Search PubMed
. - J. J. Campbell, N. Davidenko, M. M. Caffarel, R. E. Cameron and C. J. Watson, PLoS One, 2011, 6, e25661 CAS
. - S. Yamada, K. Yamamoto, T. Ikeda, K. Yanagiguchi and Y. Hayashi, BioMed Res. Int., 2014, 2014, 302932 CrossRef PubMed
. - K. Yamamoto, K. Igawa, K. Sugimoto, Y. Yoshizawa, K. Yanagiguchi, T. Ikeda, S. Yamada and Y. Hayashi, BioMed Res. Int., 2014, 2014, 630757 Search PubMed
. - F. Subhan, M. Ikram, A. Shehzad and A. Ghafoor, J. Food Sci. Technol., 2015, 52, 4703–4707 CrossRef CAS PubMed
. - M. Jackson, L. P. Choo, P. H. Watson, W. C. Halliday and H. H. Mantsch, Biochim. Biophys. Acta, 1995, 1270, 1–6 CrossRef
. - M. G. Sankalia, R. C. Mashru, J. M. Sankalia and V. B. Sutariya, AAPS PharmSciTech, 2005, 6, E209–E222 CrossRef PubMed
. - B. Samiey and F. Ashoori, Chem. Cent. J., 2012, 6, 14 CrossRef CAS PubMed
. - L. L. Fernandes, C. X. Resende, D. S. Tavares, G. A. Soares, L. O. Castro and J. M. Granjeiro, Polimeros, 2011, 21, 1–6 CAS
. - H. Tan, C. R. Chu, K. A. Payne and K. G. Marra, Biomaterials, 2009, 30, 2499–2506 CrossRef CAS PubMed
. - J. A. Burdick, C. Chung, X. Jia, M. A. Randolph and R. Langer, Biomacromolecules, 2005, 6, 386–391 CrossRef CAS PubMed
. - L. Pescosolido, T. Vermonden, J. Malda, R. Censi, W. J. Dhert, F. Alhaique, W. E. Hennink and P. Matricardi, Acta Biomater., 2011, 7, 1627–1633 CrossRef CAS PubMed
. - P. B. Kajjari, L. S. Manjeshwar and T. M. Aminabhavi, AAPS PharmSciTech, 2012, 13, 1147–1157 CrossRef CAS PubMed
. - C. Lee, J. Shin, J. S. Lee, E. Byun, J. H. Ryu, S. H. Um, D. I. Kim, H. Lee and S. W. Cho, Biomacromolecules, 2013, 14, 2004–2013 CrossRef CAS PubMed
. - S. Huang and X. Fu, J. Controlled Release, 2010, 142, 149–159 CrossRef CAS PubMed
. - X. Li, J. Wang, G. Su, Z. Zhou, J. Shi, L. Liu, M. Guan and Q. Zhang, J. Biomed. Mater. Res., Part A, 2012, 100, 396–405 CrossRef PubMed
. - M. M. Chen, Y. Q. Huang, H. Guo, Y. Liu, J. H. Wang, J. L. Wu and Q. Q. Zhang, J. Appl. Polym. Sci., 2014, 131, 40998 Search PubMed
. - H. Cao, M. M. Chen, Y. Liu, Y. Y. Liu, Y. Q. Huang, J. H. Wang, J. D. Chen and Q. Q. Zhang, Colloids Surf., B, 2015, 136, 1098–1106 CrossRef CAS PubMed
. - D. J. Choi, S. M. Choi, H. Y. Kang, H. J. Min, R. Lee, M. Ikram, F. Subhan, S. W. Jin, Y. H. Jeong, J. Y. Kwak and S. Yoon, J. Biotechnol., 2015, 205, 47–58 CrossRef CAS PubMed
. - A. Kuzan, A. Smulczyńska-Demel, A. Chwiłkowska, J. Saczko, A. Frydrychowski and M. Dominiak, Adv. Clin. Exp. Med., 2015, 24, 385–392 CrossRef PubMed
. - J. Friedrich, C. Seidel, R. Ebner and L. A. Kunz-Schughart, Nat. Protoc., 2009, 4, 309–324 CrossRef CAS PubMed
. - F. Hirschhaeuser, H. Menne, C. Dittfeld, J. West, W. Mueller-Klieser and L. A. Kunz-Schughart, J. Biotechnol., 2010, 148, 3–15 CrossRef CAS PubMed
. - H. Page, P. Flood and E. G. Reynaud, Cell Tissue Res., 2013, 352, 123–131 CrossRef PubMed
. - O. S. Aljitawi, D. Li, Y. Xiao, D. Zhang, K. Ramachandran, L. Stehno-Bittel, P. Van Veldhuizen, T. L. Lin, S. Kambhampati and R. Garimella, Leuk. Lymphoma, 2014, 55, 378–391 CrossRef CAS PubMed
. - M. Rimann, S. Laternser, A. Gvozdenovic, R. Muff, B. Fuchs, J. M. Kelm and U. Graf-Hausner, J. Biotechnol., 2014, 189, 129–135 CrossRef CAS PubMed
. - H. J. Kong, M. K. Smith and D. J. Mooney, Biomaterials, 2003, 24, 4023–4029 CrossRef CAS PubMed
. - L. Dini, E. Panzarini, M. A. Miccoli, V. Miceli, C. Protopapa and P. A. Ramires, Tissue Cell, 2005, 37, 479–487 CrossRef CAS PubMed
. - C. R. Jackson, P. M. V. Raja and S. Prakash, J. Biotechnol. Biomater., 2012, 6, 002 Search PubMed
. - A. Datar, P. Joshi and M. Y. Lee, Biosensors, 2015, 5, 647–663 CrossRef PubMed
. - H. J. Choi, T. D. Yoon, I. Muhammad, M. H. Jeong, J. Lee, S. Y. Baek, B. S. Kim and S. Yoon, Biochem. Biophys. Res. Commun., 2012, 425, 250–255 CrossRef CAS PubMed
. - M. Mohtashami and J. C. Zúñiga-Pflücker, J. Immunol., 2006, 176, 730–734 CrossRef CAS
.
|
This journal is © The Royal Society of Chemistry 2016 |
Click here to see how this site uses Cookies. View our privacy policy here.