DOI:
10.1039/C6RA02733D
(Paper)
RSC Adv., 2016,
6, 46853-46863
Novel A–(π–D–π–A)1–3 branched fluorophores displaying high two-photon absorption†
Received
29th January 2016
, Accepted 4th May 2016
First published on 5th May 2016
Abstract
A series of novel acceptor–π–donor–π–acceptor branched fluorophores with a 1,3,5-triazine core (LB1, LB2, LB3, DB1, DB2, DB3, and EQ3) were synthesized and characterized by infrared, hydrogen-1 nuclear magnetic resonance, carbon-13 nuclear magnetic resonance, mass spectrometry, and elemental analysis. Their photophysical properties in different solvents were systematically investigated including linear absorption, single-photon excited fluorescence, two-photon absorption, and frequency up-converted fluorescence. When the number of branches increases, the spectral positions of the linear absorption and the single-photon excited fluorescence show red shifts, while the fluorescence quantum yields decrease. When the polarity of solvents increases, the spectral positions of the single-photon excited fluorescence and the Stokes shifts also show red shifts, while the linear absorption wavelengths slightly change and the fluorescence quantum yields decrease. Under the excitation of a femtosecond laser (690–890 nm, 80 MHz, 140 fs), all these compounds emit intense green frequency up-converted fluorescence, and the two-photon absorption cross-sections in THF are 236, 1149, 2250, 207, 1015, 2028, and 546 GM for LB1, LB2, LB3, DB1, DB2, DB3, and EQ3, respectively. The quantum chemical calculations based on density functional theory provide supporting evidence that significant enhancement of the two-photon absorption cross-section can be achieved by sufficient electronic coupling between the strong charge transfer acceptor–π–donor–π–acceptor quadrupolar branches through the 1,3,5-triazine core.
Introduction
Two-photon absorption (TPA) is a process in which two photons are simultaneously absorbed by a medium via a virtual state under intense laser pulses. Since Göppert-Mayer's ground-breaking theoretical prediction in 1931 1 and the experimental verification reported by Kaiser and Garrett in 1961,2 TPA has aroused considerable interest due to its potential extensive applications in many fields, including fluorescence imaging,3 fluorescent microscopy,4 three-dimensional optical data storage,5 three-dimensional microfabrication,6 frequency up-converted lasing,7 optical power limiting,8 and photodynamic therapy.9 To realize these applications, novel materials with large TPA cross-sections (σ) in the visible, near-infrared, and telecommunication wavelengths are desired.
σ values determine the efficiency of TPA process. At the molecular level, materials which are likely to exhibit large σ must have polarizable electrons spread over a long distance. Theoretical studies suggest that effective electronic delocalization and increased intramolecular charge transfer are the critical factors to improve σ values. Several molecular design strategies have been developed based on these. Among them, the basic structural motifs are D–π–A,10 D–π–D/A–π–A,11 and D–π–A–π–D/A–π–D–π–A12 (D = donor, A = acceptor, π = conjugated bridge). Recently, great efforts have been focused on the design and synthesis of multi-branched TPA materials as increased dimensionality and branched structures might enhance σ comparing with the monomer counterparts. However, up to now, the mechanism of such enhancement is still not very clear. Though many reported data support the cooperative enhancement, the enhanced extents are quite different, some of which are very small.13 The experimental and theoretical investigations show that the type of cores/π-bridges/peripheries, donor/acceptor strength, conjugation length, and geometry have significant influence on the TPA properties in multi-branched materials. Consequently, more efforts should be devoted to the optimization of multi-branched structures using suitable building blocks and configuration, so as to figure out the relationship between structures and TPA properties and make progress toward real applications.
Many heterocyclic moieties, which are either electron-donating such as pyrrole14 and carbazole15 or electron-withdrawing such as benzothiazole,16 oxadiazole,17 and pyrazine,18 have been used as cores, nodes, or end groups in the design of multi-branched TPA materials. 1,3,5-Triazine is a strong π-electron deficient heterocycle with its ionization potential value of 11.67 eV. Because of its high electron affinity, it can be used as an effective acceptor. In addition, the structural symmetry and the spatial coplanarity make it easily functionalized with a variety of desired molecules and obtain excellent chemical and physical performance for the applications in optoelectronics and photonics. For example, Liu et al.19 reported a series of photosensitizers utilizing 1,3,5-triazine as π-spacers, which exhibit good photovoltaic performances of organic solar cells. An et al.20 synthesized a series of conjugated asymmetric donor-substituted 1,3,5-triazines, which can be not only good host materials for organic light-emitting diodes with a high triplet energy up to 3.0 eV, but also blue-light-emitting materials with the quantum efficiency up to 0.35 in solid film. Pyridine is another interesting aromatic heterocycle. It possesses a π-conjugated electron system and can also act as an effective acceptor due to the deficiency of electron density on the ring C atoms. Furthermore, the lone pair of electrons on the N atom of pyridine is not delocalized, thus it is easy to form pyridinium salts, which greatly improves water solubility and makes its derivatives suitable for biological applications such as fluorescent probes for live cell imaging.21 The nature of π-bridges also plays an important role in increasing σ values. Alkene π-bridge is most widely studied, and it has been suggested that alkene π-bridged materials have superior TPA properties over the alkyne π-bridged counterparts.22
To our knowledge, till now, multi-branched TPA materials are normally designed by linking several D–π–A dipolar molecules through a common core. Little attention is paid to the incorporation of D–π–A–π–D/A–π–D–π–A quadrupolar molecules into multi-branched structures. Compared with D–π–A dipolar molecules, D–π–A–π–D/A–π–D–π–A quadrupolar molecules have been experimentally23 and theoretically24 demonstrated to exhibit enhanced σ values that are approximately an order of magnitude greater than those of dipolar analogues. In addition, quadrupolar molecules have stronger fluorescence relative to corresponding dipolar molecules, most likely due to cancellation of D–A dipoles which otherwise would tend to induce quenching of fluorescence. Such augmented fluorescence are useful, for example, in imaging technologies that rely on TPA.11e,25
In this work, we designed and synthesized a series of novel one-branched (LB1, DB1), two-branched (LB2, DB2), and three-branched (LB3, DB3, EQ3) molecules, in which 1,3,5-triazine, pyridine, and formyl groups are used as electron acceptors and methoxy groups as electron donors, and they form A–π–D–π–A conjugation along the extended 1,4-phenylenedivinylene/styrene π-bridge. The single- and two-photon absorption and fluorescence properties were systematically investigated and compared. In addition, HOMO–LUMO calculations were carried out to provide information on the electronic charge redistribution occurring in those molecules upon excitation.
Results and discussion
Synthesis
The target compounds (LB1–LB3, DB1–DB3, EQ3) were synthesized according to Scheme 1.
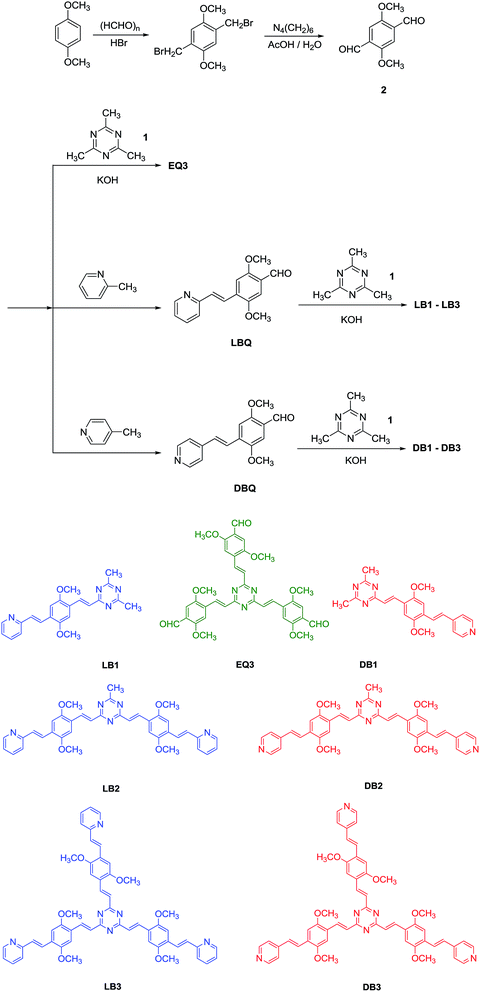 |
| Scheme 1 Synthesis of the target compounds (LB1–LB3, DB1–DB3, EQ3). | |
2,4,6-Trimethyl-1,3,5-triazine (1) was prepared by trimerization of ethyl acetimidate in the presence of about 8 mole per cent of glacial acetic acid based on the imidate content. 2,5-Dimethoxy-1,4-benzenedicarboxaldehyde (2) was synthesized by reactions of 1,4-dimethoxybenzene with paraformaldehyde and hydrogen bromide (33% solution in acetic acid), then further with hexamethylenetetramine, followed by hydrolysis in acetic acid. 2 was condensed with 2-methylpyridine or 4-methylpyridine in acetic anhydride and acetic acid solutions to form two new important aldehyde intermediates (LBQ, DBQ). Finally, the alkaline condensation of the respective aldehyde (2, LBQ, DBQ) with 1 in methanol gave seven new target compounds (LB1–LB3, DB1–DB3, EQ3) in moderate yields. Because of three same reaction sites in 1, the molar ratio of 1 to the aldehydes and the feeding sequence of them were considered as the key factors to obtain better yields for the products with the different number of branches.
All the target compounds (LB1–LB3, DB1–DB3, EQ3) were purified by column chromatography on silica gel and characterized by 1H and 13C NMR, IR, MS, and elemental analysis. They are well-defined all-trans isomers. The chemical shifts of trans-vinyl protons with the coupling constants of 16.0–16.6 Hz can be assigned in the 1H NMR spectra. As the branch number increases, the protons in methoxy group, benzene ring, and vinyl group linked directly to the triazine ring shift a little to the lower field. The singlets for methyl protons of the one- and two-branched compounds at 2.56 ppm (LB1, DB1) and 2.62 ppm (LB2, DB2) disappear completely in the three-branched compounds (LB3, DB3). The representative 1H NMR spectra (LB3, DB3) and assignments are shown in Fig. S1 (ESI†).
In the FT-IR spectra, the characteristic absorption peaks of C
C, C–O–C, and C
O are observed at 1611–1628 cm−1, 1209–1212 cm−1 (1039–1043 cm−1) and 1679 cm−1, respectively. It can be seen that seven target compounds exhibit a very strong band at 1500–1536 cm−1, which is assigned to the skeleton vibration absorption of the triazine ring. And the wavenumber value of this band decreases from 1536 (1534) cm−1 to 1518 (1517) cm−1 and 1503 (1500) cm−1 when going from LB1 (DB1) to LB2 (DB2) and LB3 (DB3). The presence of a band at 971–990 cm−1, which corresponds to the out-of-plane bending mode of the trans vinyl group, further supports the E-configurations of the C–C double bonds.
In the ESI-MS spectra of DB2 and DB3, both signals of [M + H]+ and [M + 2H]2+ are detected. In the case of LB2 and LB3, only the signal of [M + H]+ is detected. The possible reason is that the alkalinity of 4-pyridine is higher than that of 2-pyridin.
Single-photon absorption and emission properties
The single-photon related photophysical properties of the target compounds (LB1–LB3, DB1–DB3, EQ3) in various solvents are listed in Table 1. Their UV-visible absorption and single-photon excited fluorescence (SPEF) spectra in THF are shown in Fig. S2 (ESI†) and Fig. 1.
Table 1 Single- and two-photon related photophysical properties of the target compounds (LB1–LB3, DB1–DB3, EQ3)
Compound |
Solvent |
λabsmaxa (nm) |
10−4εb (mol−1 L cm−1) |
λSPEFmaxc (nm) |
Δvd (cm−1) |
Φe |
λTPEFmaxf (nm) |
σg (GM) |
σ/MWh |
Maximum linear absorption wavelength, c = 1 × 10−5 mol L−1. Molar extinction coefficient. Maximum single-photon excited fluorescence wavelength, c = 1 × 10−7 mol L−1. Stokes shift. Fluorescence quantum yield. Maximum two-photon excited fluorescence wavelength, c = 1 × 10−3 mol L−1. Two-photon absorption cross-section, 1 GM = 1 × 10−50 cm4 s per photon. Reduced TPA cross-section, i.e., two-photon absorption cross-section divided by molecular weight. The numbers in parentheses are relative values. |
LB1 |
THF |
408 |
4.73 |
479 |
3633 |
0.91 |
508 |
236 |
0.631 (1.0) |
CH2Cl2 |
409 |
4.71 |
490 |
4042 |
0.85 |
520 |
215 |
0.575 (1.0) |
CH3CN |
406 |
5.98 |
496 |
4469 |
0.54 |
|
|
|
LB2 |
THF |
421 |
5.37 |
483 |
3049 |
0.53 |
524 |
1149 |
1.838 (2.9) |
CH2Cl2 |
421 |
5.47 |
499 |
3713 |
0.42 |
535 |
1046 |
1.674 (2.9) |
CH3CN |
420 |
8.32 |
504 |
3968 |
0.08 |
|
|
|
LB3 |
THF |
425 |
8.96 |
487 |
2996 |
0.34 |
530 |
2250 |
2.568 (4.1) |
CH2Cl2 |
426 |
7.38 |
504 |
3633 |
0.21 |
546 |
2031 |
2.318 (4.0) |
CH3CN |
426 |
9.64 |
511 |
3905 |
0.02 |
|
|
|
DB1 |
THF |
406 |
4.36 |
478 |
3710 |
0.86 |
506 |
207 |
0.553 (1.0) |
CH2Cl2 |
408 |
4.62 |
488 |
4018 |
0.74 |
519 |
186 |
0.497 (1.0) |
CH3CN |
404 |
5.84 |
494 |
4510 |
0.48 |
|
|
|
DB2 |
THF |
422 |
4.92 |
482 |
2950 |
0.47 |
520 |
1015 |
1.624 (2.9) |
CH2Cl2 |
421 |
5.38 |
495 |
3551 |
0.34 |
527 |
952 |
1.523 (3.1) |
CH3CN |
420 |
7.55 |
503 |
3929 |
0.05 |
|
|
|
DB3 |
THF |
424 |
8.12 |
484 |
2924 |
0.31 |
525 |
2028 |
2.315 (4.2) |
CH2Cl2 |
426 |
7.22 |
500 |
3474 |
0.18 |
539 |
1881 |
2.147 (4.3) |
CH3CN |
425 |
9.21 |
509 |
3883 |
0.02 |
|
|
|
EQ3 |
THF |
329 |
5.13 |
482 |
9648 |
0.67 |
519 |
546 |
0.839 |
CH2Cl2 |
328 |
6.47 |
487 |
9954 |
0.48 |
523 |
428 |
0.657 |
CH3CN |
325 |
4.84 |
498 |
10 689 |
0.10 |
|
|
|
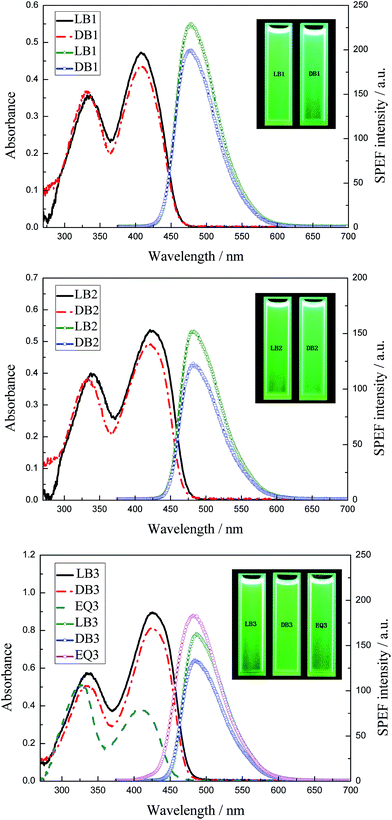 |
| Fig. 1 UV-visible absorption spectra (left, c = 1 × 10−5 mol L−1) and single-photon excited fluorescence spectra (right, c = 1 × 10−7 mol L−1) of the target compounds (LB1–LB3, DB1–DB3, EQ3) in THF. Insets are the fluorescence photographs in CH2Cl2. | |
All the target compounds exhibit two major absorption bands: the peak at about 325–340 nm is assigned to the π–π* electronic transition caused by styrene, while the other peak at about 404–426 nm indicates considerable charge transfer character. The absorption maxima (λabsmax) and shapes of the compounds show weak solvent polarity dependencies. As can be seen from Table 1, the differences among λabsmax in various solvents do not exceed 4 nm. When the number of branches increases, the λabsmax in THF is bathochromically shifted from 408 nm of LB1 (406 nm of DB1) to 421 nm of LB2 (422 nm of DB2), and to 425 nm of LB3 (424 nm of DB3), respectively. Similar results are obtained in the other solvents. The molar extinction coefficients (ε) also increase monotonically and obviously following the order: LB1 < LB2 < LB3 and DB1 < DB2 < DB3. These give a clear indication that a certain coupling between the branches results in charge redistribution and extended π-delocalization. Compared LB1 with DB1, LB2 with DB2, and LB3 with DB3, respectively, they display similar absorption profiles and very close absorption peaks in the same solvent, except for the enhancement in ε for LB1–LB3 relative to DB1–DB3 (LB1 > DB1, LB2 > DB2, LB3 > DB3). The λabsmax of EQ3 is hypsochromically shifted by about 95 nm than those of the other three-branched compounds (LB3, DB3), which indicates that EQ3 has weaker intramolecular charge transfer (ICT) than LB3 and DB3.
Excited by the maximum absorption wavelengths, all the target compounds undergo single-photon emission processes with the maximal peaks at 478–511 nm in different solvents. It is observed that none of them has multiple peaks, which indicates that the emission occurs from the lowest excited state (S1) with the largest oscillator strength. In contrast to the absorption properties, the SPEF maxima (λSPEFmax) display dependencies on solvent polarity. The empirical parameter ET(30) is widely used to describe solvent polarity quantitatively. The polarity of the selected solvents can be sequenced as THF (ET(30) = 37.4) < CH2Cl2 (ET(30) = 40.7) < CH3CN (ET(30) = 45.6).26 Upon increasing solvent polarity, both the λSPEFmax and the Stokes shift (Δv) show red shifts. These facts can be attributed to that the excited state (S1) may possess higher polarity than that of the ground state (S0), as a result, solute/solvent interaction at S1 is stronger than that at S0, which leads to lower the energy gap between S0 and S1 greatly. Similar to the absorption properties, the λSPEFmax is also red-shifted with the increase of branches owing to the cross-conjugation effect. However, there is a corresponding drop of the Δv. For example, the Δv in THF decreases from 3633 cm−1 to 3049 and 2996 cm−1 when going from LB1 to LB2 and LB3, which implies that the polarity of the branched compounds at the excited state is LB1 > LB2 > LB3 and DB1 > DB2 > DB3. Compared LB1 with DB1, LB2 with DB2, and LB3 with DB3, respectively, the emission peak positions are almost the same, while the fluorescence intensities increase following the order: LB1 > DB1, LB2 > DB2, and LB3 > DB3, which may be derived from the enhanced absorbance of LB1–LB3 relative to DB1–DB3. The fluorescence of EQ3 is also in the cyan-green region, with a peak at 482 nm in THF, 487 nm in CH2Cl2, and 498 nm in CH3CN, which does not vary significantly as the λabsmax does in comparison with LB3 and DB3. But its emission peak is broader and higher than those of LB3 and DB3, indicating its stronger fluorescence emission ability.
The fluorescence quantum yields (Φ) were measured in different solvents. Fluorescein in 0.1 mol L−1 sodium hydroxide was used as a standard (Φ = 0.9 27). All the target compounds show the positive solvatokinetic effect. Upon increasing solvent polarity, the Φ decreases. Especially, the Φ dramatically decreases to 0.08 for LB2, 0.05 for DB2, and 0.02 for LB3 and DB3 in highly polar CH3CN. Another noteworthy observation is that the Φ of the one-branched, two-branched, and three-branched compounds can be sequenced as LB1 > DB1 > EQ3 > LB2 > DB2 > LB3 > DB3. Since the target compounds possess electron donors and acceptors joined by flexible bonds, the main reason for these results should be the enhancement of the twisted intramolecular charge transfer (TICT) effect.28 The TICT state is non-emissive because of rapid transfer to nonradiative triplets.
By comparing the single-photon related photophysical data in Table 1, we can find that the linear spectral behaviors (λabsmax, λSPEFmax, Δv, and Φ) of the two-branched compounds are closer to those of the three-branched compounds, while more differences with those of the one-branched compounds. These imply that the electronic structure of LB2 (DB2) is more similar to LB3 (DB3), rather than to LB1 (DB1).
Two-photon properties
The two-photon related photophysical properties of the target compounds (LB1-LB3, DB1–DB3, EQ3) are listed in Table 1. Pumped by femtosecond laser pulses at 2.27 × 107 W cm−2 under different excitation wavelengths (690–890 nm), their two-photon excited fluorescence (TPEF) spectra in THF and CH2Cl2 (c = 1 × 10−3 mol L−1) were recorded. The representative TPEF spectra of LB3 and DB3 in THF are shown in Fig. 2.
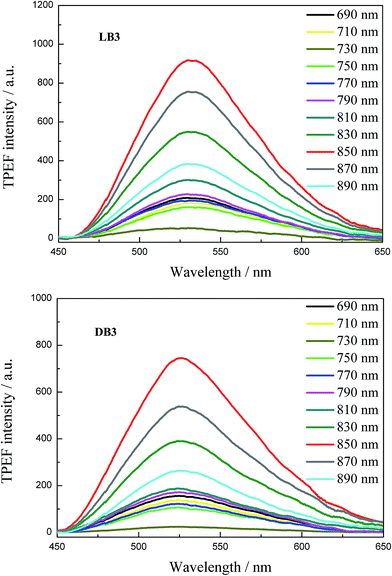 |
| Fig. 2 Two-photon excited fluorescence spectra of LB3 and DB3 in THF (c = 1 × 10−3 mol L−1) pumped by femtosecond laser pulses at 2.27 × 107 W cm−2 under different excitation wavelengths. | |
All the target compounds emit intense frequency up-converted fluorescence with the maximum TPEF wavelengths (λTPEFmax) at 508 nm (THF) and 520 nm (CH2Cl2) for LB1, 524 nm (THF) and 535 nm (CH2Cl2) for LB2, 530 nm (THF) and 546 nm (CH2Cl2) for LB3, 506 nm (THF) and 519 nm (CH2Cl2) for DB1, 520 nm (THF) and 527 nm (CH2Cl2) for DB2, 525 nm (THF) and 539 nm (CH2Cl2) for DB3, and 519 nm (THF) and 523 nm (CH2Cl2) for EQ3, respectively. Because 1,3,5-triazine and pyridine (formyl) serve as electron acceptors, methoxy groups as electron donors, and 1,4-phenylenedivinylene (styrene) as π-bridge in these compounds, the ICT effect should be considered. The single peaks in the TPEF spectra indicate that the state responsible for the fluorescence emission is not the locally excited state (LE) but the ICT state. From Table 1, it is obvious that the λTPEFmax is red-shifted by 28–43 nm compared with the corresponding λSPEFmax in THF and CH2Cl2. This can be attributed to the reabsorption effect. For the TPEF measurements, concentrated solutions (1 × 10−3 mol L−1) were used instead of dilute solutions (1 × 10−7 mol L−1), the reabsorption of the shorter wavelength fluorescence can no longer be neglected. Except for such a difference in the peak positions, there are many similarities between TPEF spectra and SPEF spectra, such as the peak shapes, the influence rule of branches, and the influence rule of solvent polarity, which imply that both the TPEF emission and the SPEF emission for these given compounds are from the same excited state, though their initial Frank–Condon states may be different. The difference between TPEF and SPEF is mainly the excitation process: two-photon absorption vs. single-photon absorption.
As shown in Fig. S2 (ESI†), in the wavelength range of 500–900 nm, all the target compounds are highly transparent without any absorption, which indicates that there are no molecular energy levels corresponding to an electron transition in this spectral range. Upon excitation from 690–890 nm, it is impossible to produce SPEF. Fig. 3a and b show TPEF spectra of LB3 and DB3 in THF under different pump powers with 850 nm excitation wavelength. Fig. 3c and d show logarithmic plots of the fluorescence integral (Iout) versus different pump powers (Iin). The slope value is 1.98 for LB3 and 1.99 for DB3, respectively. In the other cases (LB1, LB2, DB1, DB2, EQ3), the output intensities of fluorescence are also linearly dependent on the square of the input laser intensities. These confirm that TPA is the main excitation mechanism of the up-converted fluorescence emission.
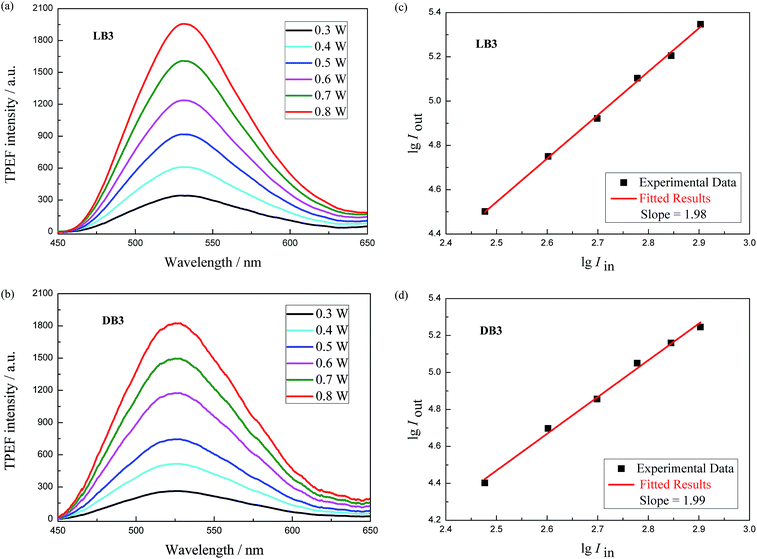 |
| Fig. 3 (a and b) Two-photon excited fluorescence spectra of LB3 and DB3 in THF (c = 1 × 10−3 mol L−1) under different pump powers at 850 nm. (c and d) Logarithmic plots of the fluorescence integral (Iout) of LB3 and DB3 versus different excitation intensities (Iin). | |
σ is obtained by comparing the TPEF intensity of the sample with that of a reference compound using the following eqn (1):29
|
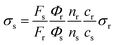 | (1) |
where the subscripts s and r denote the sample and the reference compound.
F and
Φ represent the TPEF integral intensity and the SPEF quantum yield.
n and
c are the refractive index and the concentration of the solution. In this work, we selected fluorescein in 0.1 mol L
−1 sodium hydroxide (
c = 1 × 10
−3 mol L
−1) as the reference (
σ = 36 GM
29).
It is accepted that σ is proportional to the imaginary part of the third-order polarizability,30 and if the frequency (w) is neglected, the third-order polarizability, γ, can be expressed as the eqn (2):31,32
|
 | (2) |
Therefore, σ predominantly depends on the transition dipole moment between the ground state and the first excited state (Mge), the transition energy between the ground state and the first excited state (Ege), and the dipole moment difference between the ground state and the first excited state (Δμge).
Fig. 4 shows the σ values of the target compounds in the femtosecond regime over the range of 690–890 nm. All the seven compounds display good TPA performances. The optimal excitation wavelengths are 810 nm (LB1, DB1, EQ3) and 850 nm (LB2, DB2, LB3, DB3) in both THF and CH2Cl2, which are located in the vicinity of twice the single-photon absorption maxima except for EQ3. This phenomenon indicates that the absorption of the ICT state play a dominant role. As shown in Fig. 4 and listed in Table 1, the σ values in THF present only a little enhancement when compared with those in CH2Cl2, whereas the effect of branching on σ is remarkable. For example, in THF, the ratio of σ is 1.0
:
4.9
:
9.5 (LB1
:
LB2
:
LB3) and 1.0
:
4.9
:
9.8 (DB1
:
DB2
:
DB3), respectively, which is greatly larger than that between the branch numbers (1
:
2
:
3). The reduced TPA cross-section (σ/MW), which is defined as σ divided by the molecular weight, also varies in a proportion of 1.0
:
2.9
:
4.1 (LB1
:
LB2
:
LB3) and 1.0
:
2.9
:
4.2 (DB1
:
DB2
:
DB3), respectively. This means that the unit molecular weight enables σ to enhance as the number of branches increases. Such dramatic enhancement for LB2 (DB2) and LB3 (DB3) should be attributed to the sufficient electronic coupling between the branches.33 Their individual branches, which have a A–π–D–π–A quadrupolar character, can not only lead to strong intra-branch charge transfer, but also facilitate inter-branch electronic communication through the 1,3,5-triazine core. Inspection of the σ values (LB1 cf. DB1, LB2 cf. DB2, LB3 cf. DB3) reveals that the strength of peripheral electron acceptors exerts an influence on the TPA properties. It is reasonable to conclude the strength of the pyridine acceptor according to the chemical shifts of the protons in vinyl group linked directly to pyridine (δ–CH
CH–Py). On the basis of the δ–CH
CH–Py which shifts downfield, 2-pyridine has a little stronger electron-withdrawing ability than 4-pyridine, so the σ values of LB1–LB3 increase slightly relative to DB1–DB3. Compared with EQ3, the σ values of LB3 and DB3 are almost four times larger. This may be ascribed to two factors. One is that the strength of the peripheral acceptor can be arrayed as pyridine > CHO. The other is that the branches of LB3 and DB3 have a longer conjugation length than those of EQ3 by the introduction of a vinyl group and pyridine. These two factors greatly increase ICT and the degree of electron delocalization.
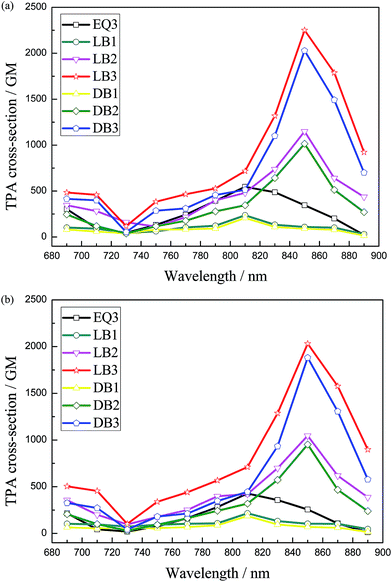 |
| Fig. 4 Two-photon absorption cross-sections of the target compounds (LB1–LB3, DB1–DB3, EQ3) in THF (a) and CH2Cl2 (b) under different excitation wavelengths. | |
To further understand the TPA properties of the target compounds, the quantum chemical calculations based on density functional theory (DFT) were performed using Gaussian 09 program. The nonlocal density functional of B3LYP with 6-31G basis sets was used for the calculations. Fig. S3 and S4 (ESI†) show the optimized geometries and the frontier molecular orbitals of the seven compounds, respectively.
All the compounds exhibit good coplanarity and strong ICT. In the case of the one-branched (LB1, DB1) and two-branched (LB2, DB2) compounds, most of the electron clouds of the HOMO are distributed in 1,4-phenylenedivinylene π-bridges, while the electron clouds of the LUMO are further delocalized on the 1,3,5-triazine core. A more pronounced charge density shift is observed in the three-branched compounds (LB3, DB3). Their electron clouds of the HOMO are essentially located on the one branch, while in the LUMO, the π-electrons are completely transferred to the other two branches and the 1,3,5-triazine core. Though EQ3 also has three branches, the degree of electron delocalization is not as high as that in LB3 and DB3. The enhancement of ICT may result in the decrease of Ege and the increase of Mge. From eqn (2), the smaller Ege and the larger Mge is, the higher γ will be, and finally leading to the increase of σ.
Conclusions
A series of novel A–(π–D–π–A)1–3 branched fluorophores built from a 1,3,5-triazine core were synthesized and characterized by IR, 1H NMR, 13C NMR, MS and elemental analysis. Structure–property relationships were derived from the comprehensive study of the single- and two-photon absorption and photoluminescence properties of these compounds. Using a femtosecond laser as the pump source, the A–(π–D–π–A)3 compounds bearing pyridine end groups (LB3, DB3) are apparently effective in achieving large two-photon responses. Their σ values in THF are 2250 GM and 2028 GM, respectively, which are higher than those of many known D–π–A three-branched compounds.34 The design of multi-branched compounds with enhanced σ mainly requires the exploitation of cores, peripheries, nodes, and π-bridges. Investigation of the TPA properties of the target compounds reveals that significant enhancement of σ can be achieved by sufficient electronic coupling between the strong charge transfer A–π–D–π–A quadrupolar branches through the 1,3,5-triazine core. We will further functionalize these compounds to increase hydrophilicity, and study their potential use in two-photon fluorescence bioimaging.
Experimental section
Materials and instruments
2,4,6-Trimethyl-1,3,5-triazine (1)35 and 2,5-dimethoxy-1,4-benzenedicarboxaldehyde (2)36 were synthesized according to the literature procedures. Other materials were commercially available and were used without further purification.
Melting points were measured on an X-4 micromelting point apparatus without correction. 1H and 13C NMR spectra were collected on a Bruker AVANCE III 500 apparatus, with TMS as internal standard and DMSO-d6 as solvent. FT-IR spectra were recorded on a Thermo Nicolet 6700 spectrometer using KBr pellets. Mass spectra were taken on a Therm LCQ TM Deca XP plus ion trap mass spectrometry instrument. Elemental analyses were conducted on a Thermo Finnigan Flash EA 1112 apparatus.
The linear absorption spectra were measured on a Shimadzu UV-2550 UV-visible spectrophotometer. The SPEF spectra measurements were performed using a RF-5301PC fluorescence spectrophotometer with the maximum absorption wavelengths as the excitation wavelengths. The fluorescence quantum yields were determined using fluorescein in 0.1 mol L−1 sodium hydroxide as the standard. The TPEF spectra were measured using a femtosecond Ti:sapphire laser (Chameleon Ultra II) as pump source with a pulse width of 140 fs and a repetition rate of 80 MHz, and tuned by step of 20 nm in the wavelength range of 690–890 nm.
2,5-Dimethoxy-4-[(1E)-2-(2-pyridinyl)ethenyl]benzaldehyde (LBQ)
A mixture of 2-methylpyridine (0.93 g, 10 mmol), 2 (1.94 g, 10 mmol), acetic anhydride (3 mL), and acetic acid (1.5 mL) was heated under reflux for 6 h, then cooled to room temperature. After concentrated hydrochloric acid (15 mL) was added, the mixture was filtered. The filtrate was neutralized with 30% aqueous sodium hydroxide solution (30 mL) and gave a precipitate. The precipitate was purified by column chromatography on silica gel using petroleum ether/ethyl acetate (10
:
1) as eluent to give bright yellow needle crystals (1.69 g, 62.8%). Mp 110–112 °C; 1H NMR (500 MHz, DMSO-d6): δ = 10.34 (s, 1H), 8.62 (d, J = 3.9 Hz, 1H), 7.99 (d, J = 16.2 Hz, 1H), 7.83 (t, J = 7.6 Hz, 1H), 7.61 (d, J = 16.2 Hz, 1H), 7.60 (s, 1H), 7.55 (d, J = 7.8 Hz, 1H), 7.31 (dd, J1 = 7.5 Hz, J2 = 4.8 Hz, 1H), 7.28 (s, 1H), 3.99 (s, 3H), 3.89 (s, 3H); FT-IR (KBr): ν = 3051, 2921, 2872, 1670, 1603, 1490, 1466, 1406, 1214, 1123, 1041, 970, 870, 770, 685 cm−1; ESI-MS: m/z (%): 270.1 (100) [M + H]+; elemental analysis calcd (%) for C16H15NO3: C 71.36, H 5.61, N 5.20; found: C 71.48, H 5.69, N 5.41.
2,5-Dimethoxy-4-[(1E)-2-(4-pyridinyl)ethenyl]benzaldehyde (DBQ)
This compound was synthesized using a procedure similar to that described for LBQ, with 4-methylpyridine instead of 2-methylpyridine. Bright yellow needle crystals. Yield 71.3%. Mp 144–146 °C; 1H NMR (500 MHz, DMSO-d6): δ = 10.34 (s, 1H), 8.59 (d, J = 5.9 Hz, 2H), 7.70 (d, J = 16.6 Hz, 1H), 7.59 (s, 1H), 7.58 (d, J = 5.9 Hz, 2H), 7.54 (d, J = 16.6 Hz, 1H), 7.29 (s, 1H), 3.98 (s, 3H), 3.89 (s, 3H); FT-IR (KBr): ν = 3064, 2947, 2873, 1681, 1600, 1485, 1469, 1408, 1215, 1124, 1035, 971, 879, 771, 691 cm−1; ESI-MS: m/z (%): 269.8 (100) [M + H]+; elemental analysis calcd (%) for C16H15NO3: C 71.36, H 5.61, N 5.20; found: C 71.59, H 5.78, N 5.47.
2-[(1E)-2-[2,5-Dimethoxy-4-[(1E)-2-(4,6-dimethyl-1,3,5-triazin-2-yl)ethenyl]phenyl]ethenyl]pyridine (LB1)
To a mixture of 1 (0.55 g, 4.5 mmol), potassium hydroxide (0.3 g), and methanol (30 mL) was added a solution of LBQ (0.81 g, 3 mmol) in methanol (30 mL) dropwise. The reaction mixture was refluxed for 20 h and then the solvent was removed. The residue was purified by column chromatography on silica gel using petroleum ether/ethyl acetate (11
:
1) as eluent to give a yellow crystalline powder (0.65 g, 57.9%). Mp 154–156 °C; 1H NMR (500 MHz, DMSO-d6): δ = 8.61 (d, J = 4.1 Hz, 1H), 8.43 (d, J = 16.1 Hz, 1H), 8.00 (d, J = 16.2 Hz, 1H), 7.83 (t, J = 7.3 Hz, 1H), 7.55 (d, J = 7.4 Hz, 1H), 7.51 (d, J = 16.2 Hz, 1H), 7.50 (s, 1H), 7.46 (s, 1H), 7.32 (d, J = 16.1, 1H), 7.30 (dd, J1 = 7.2 Hz, J2 = 4.9 Hz, 1H), 3.97 (s, 3H), 3.94 (s, 3H), 2.56 (s, 6H); 13C NMR (500 MHz, DMSO-d6): δ 175.56, 170.63, 155.01, 152.24, 151.38, 149.59, 136.88, 135.52, 129.59, 127.91, 126.01, 125.94, 123.95, 122.85, 122.46, 110.80, 109.67, 56.20, 56.17, 25.17; FT-IR (KBr): ν = 3064, 2930, 2831, 1628, 1584, 1536, 1467, 1409, 1375, 1212, 1041, 986, 850, 773 cm−1; ESI-MS: m/z (%): 375.3 (100) [M + H]+; elemental analysis calcd (%) for C22H22N4O2: C 70.57, H 5.92, N 14.96; found: C 70.67, H 6.17, N 15.18.
4-[(1E)-2-[2,5-Dimethoxy-4-[(1E)-2-(4,6-dimethyl-1,3,5-triazin-2-yl)ethenyl]phenyl]ethenyl]pyridine (DB1)
This compound was synthesized using a procedure similar to that described for LB1, with DBQ instead of LBQ. Bright yellow crystalline powder. Yield 66.1%. Mp 195–197 °C; 1H NMR (500 MHz, DMSO-d6): δ = 8.59 (d, J = 6.0 Hz, 2H), 8.43 (d, J = 16.1 Hz, 1H), 7.73 (d, J = 16.6 Hz, 1H), 7.61 (d, J = 5.9 Hz, 2H), 7.52 (s, 1H), 7.46 (d, J = 16.6 Hz, 1H), 7.45 (s, 1H), 7.33 (d, J = 16.1, 1H), 3.96 (s, 3H), 3.94 (s, 3H), 2.56 (s, 6H); 13C NMR (500 MHz, DMSO-d6): δ 175.56, 170.59, 152.18, 151.32, 150.08, 144.37, 135.39, 127.85, 127.49, 126.88, 126.26, 124.30, 120.82, 110.81, 110.00, 56.25, 56.17, 25.17; FT-IR (KBr): ν = 3048, 2924, 2835, 1627, 1591, 1534, 1465, 1413, 1375, 1211, 1041, 987, 854, 802 cm−1; ESI-MS: m/z (%): 375.3 (100) [M + H]+; elemental analysis calcd (%) for C22H22N4O2: C 70.57, H 5.92, N 14.96; found: C 70.71, H 6.12, N 15.11.
2,2′-[(6-Methyl-1,3,5-triazine-2,4-diyl)bis[(1E)-2,1-ethenediyl(2,5-dimethoxy-4,1-phenylene)-(1E)-2,1-ethenediyl]]bispyridine (LB2)
This compound was synthesized using a procedure similar to that described for LB1 except that the molar ratio between LBQ and 1 was changed to 2.5
:
1. Orange-yellow crystalline powder. Yield 42.8%. Mp 236–238 °C; 1H NMR (500 MHz, DMSO-d6): δ = 8.60 (d, J = 4.0 Hz, 2H), 8.48 (d, J = 16.1 Hz, 2H), 8.00 (d, J = 16.2 Hz, 2H), 7.80 (t, J = 7.6 Hz, 2H), 7.52 (d, J = 7.5 Hz, 2H), 7.51 (s, 2H), 7.49 (d, J = 16.2 Hz, 2H), 7.47 (s, 2H), 7.34 (d, J = 16.1, 2H), 7.27 (dd, J1 = 7.3 Hz, J2 = 5.0 Hz, 2H), 3.99 (s, 6H), 3.96 (s, 6H), 2.62 (s, 3H); 13C NMR (500 MHz, DMSO-d6): δ 175.64, 170.89, 155.04, 152.27, 151.42, 149.61, 136.90, 135.42, 129.60, 127.92, 126.28, 125.98, 124.06, 122.87, 122.48, 110.77, 109.70, 56.26, 56.22, 25.42; FT-IR (KBr): ν = 3053, 2921, 2851, 1627, 1585, 1518, 1467, 1408, 1370, 1211, 1042, 978, 853, 772 cm−1; ESI-MS: m/z (%): 626.5 (100) [M + H]+; elemental analysis calcd (%) for C38H35N5O4: C 72.94, H 5.64, N 11.19; found: C 73.14, H 5.78, N 11.43.
4,4′-[(6-Methyl-1,3,5-triazine-2,4-diyl)bis[(1E)-2,1-ethenediyl(2,5-dimethoxy-4,1-phenylene)-(1E)-2,1-ethenediyl]]bispyridine (DB2)
This compound was synthesized using a procedure similar to that described for LB1 except that LBQ was replaced with DBQ and the molar ratio between DBQ and 1 was changed to 2.5
:
1. Orange-yellow crystalline powder. Yield 46.5%. Mp 247–249 °C; 1H NMR (500 MHz, DMSO-d6): δ = 8.56 (d, J = 6.0 Hz, 4H), 8.48 (d, J = 16.0 Hz, 2H), 7.70 (d, J = 16.5 Hz, 2H), 7.56 (d, J = 6.0 Hz, 4H), 7.54 (s, 2H), 7.46 (s, 2H), 7.44 (d, J = 16.5 Hz, 2H), 7.37 (d, J = 16.0, 2H), 3.98 (s, 6H), 3.96 (s, 6H), 2.62 (s, 3H); 13C NMR (500 MHz, DMSO-d6): δ 175.69, 170.86, 152.21, 151.40, 149.72, 144.81, 135.33, 127.80, 127.48, 127.25, 126.60, 124.47, 120.98, 110.88, 110.13, 56.30, 56.25, 25.42; FT-IR (KBr): ν = 3051, 2933, 2832, 1626, 1593, 1517, 1465, 1411, 1370, 1212, 1043, 971, 853, 805 cm−1; ESI-MS: m/z (%): 626.3 (100) [M + H]+, 313.9 (10) [M + 2H]2+; elemental analysis calcd (%) for C38H35N5O4: C 72.94, H 5.64, N 11.19; found: C 73.09, H 5.86, N 11.38.
2,2′,2′′-[1,3,5-Triazine-2,4,6-triyltris[(1E)-2,1-ethenediyl(2,5-dimethoxy-4,1-phenylene)-(1E)-2,1-ethenediyl]]trispyridine (LB3)
This compound was synthesized using a procedure similar to that described for LB1 except that the molar ratio between LBQ and 1 was changed to 6
:
1 and 1 was added to LBQ instead of the latter being added to the former. Orange-yellow crystalline powder. Yield 38.4%. Mp 252–254 °C; 1H NMR (500 MHz, DMSO-d6): δ = 8.61 (d, J = 4.1 Hz, 3H), 8.56 (d, J = 16.0 Hz, 3H), 8.02 (d, J = 16.2 Hz, 3H), 7.81 (t, J = 7.6 Hz, 3H), 7.56 (s, 3H), 7.53 (d, J = 7.6 Hz, 3H), 7.51 (d, J = 16.2 Hz, 3H), 7.50 (s, 3H), 7.40 (d, J = 16.0, 3H), 7.28 (dd, J1 = 7.4 Hz, J2 = 5.0 Hz, 3H), 4.02 (s, 9H), 3.99 (s, 9H); 13C NMR (500 MHz, DMSO-d6): δ 170.98, 155.05, 152.29, 151.43, 149.63, 136.93, 135.49, 129.62, 127.96, 126.26, 125.99, 124.16, 122.88, 122.64, 110.65, 109.72, 56.27, 56.24; FT-IR (KBr): ν = 3052, 2934, 2830, 1625, 1584, 1503, 1466, 1407, 1373, 1211, 1041, 979, 855, 773 cm−1; ESI-MS: m/z (%): 877.3 (100) [M + H]+; elemental analysis calcd (%) for C54H48N6O6: C 73.95, H 5.52, N 9.58; found: C 74.21, H 5.76, N 9.89.
4,4′,4′′-[1,3,5-Triazine-2,4,6-triyltris[(1E)-2,1-ethenediyl(2,5-dimethoxy-4,1-phenylene)-(1E)-2,1-ethenediyl]]trispyridine (DB3)
This compound was synthesized using a procedure similar to that described for LB1 except that LBQ was replaced with DBQ, the molar ratio between DBQ and 1 was changed to 6
:
1, and 1 was added to DBQ instead of the latter being added to the former. Orange-yellow crystalline powder. Yield 43.2%. Mp 283–285 °C; 1H NMR (500 MHz, DMSO-d6): δ = 8.61 (d, J = 5.8 Hz, 6H), 8.56 (d, J = 16.0 Hz, 3H), 7.77 (d, J = 16.6 Hz, 3H), 7.62 (d, J = 5.8 Hz, 6H), 7.59 (s, 3H), 7.49 (d, J = 16.6 Hz, 3H), 7.50 (s, 3H), 7.43 (d, J = 16.0, 3H), 4.01 (s, 9H), 4.00 (s, 9H); 13C NMR (500 MHz, DMSO-d6): δ 170.97, 152.18, 151.36, 150.08, 144.40, 135.14, 127.82, 127.52, 126.94, 126.70, 124.49, 120.84, 110.64, 110.05, 56.30, 56.26; FT-IR (KBr): ν = 3053, 2932, 2831, 1624, 1593, 1500, 1464, 1410, 1374, 1211, 1042, 990, 855, 804 cm−1; ESI-MS: m/z (%): 877.3 (20) [M + H]+, 439.2 (100) [M + 2H]2+; elemental analysis calcd (%) for C54H48N6O6: C 73.95, H 5.52, N 9.58; found: C 74.19, H 5.68, N 9.83.
4,4′,4′′-[1,3,5-Triazine-2,4,6-triyltri-(1E)-2,1-ethenediyl]tris[2,5-dimethoxybenzaldehyde] (EQ3)
This compound was synthesized using a procedure similar to that described for LB1 except that LBQ was replaced with 2, the molar ratio between 2 and 1 was changed to 6
:
1, and 1 was added to 2 instead of the latter being added to the former. Yellow crystalline powder. Yield 53.7%. Mp > 300 °C; 1H NMR (500 MHz, DMSO-d6): δ = 10.38 (s, 3H), 8.56 (d, J = 16.1 Hz, 3H), 7.79 (s, 3H), 7.60 (d, J = 16.1 Hz, 3H), 7.35 (s, 3H), 4.03 (s, 9H), 3.96 (s, 9H); 13C NMR (500 MHz, DMSO-d6): δ 191.83, 170.86, 155.79, 151.67, 130.58, 129.57, 125.39, 125.25, 112.23, 109.12, 56.54, 56.09; FT-IR (KBr): ν = 3070, 2928, 2860, 1679, 1611, 1504, 1409, 1377, 1209, 1123, 1039, 982, 872, 785, 689, 628 cm−1; ESI-MS: m/z (%): 652.2 (100) [M + H]+; elemental analysis calcd (%) for C36H33N3O9: C 66.35, H 5.10, N 6.45; found: C 66.59, H 5.18, N 6.67.
Acknowledgements
We are grateful to the financial supports from the Natural Science Foundation of Zhejiang province, China (Grant No. LY15B030006) and the National Natural Science Foundation of China (Grant No. 21103151).
References
- M. Göppert-Mayer, Ann. Phys., 1931, 9, 273–295 CrossRef.
- W. Kaiser and C. G. B. Garrett, Phys. Rev. Lett., 1961, 7, 229–231 CrossRef CAS.
-
(a) C. Barsu, R. Cheaib, S. Chambert, Y. Queneau, O. Maury, D. Cottet, H. Wege, J. Douady, Y. Bretonniere and C. Andraud, Org. Biomol. Chem., 2010, 8, 142–150 RSC;
(b) P. T. C. So, C. Y. Dong, B. R. Masters and K. M. Berland, Annu. Rev. Biomed. Eng., 2000, 2, 399–429 CrossRef CAS PubMed;
(c) D. R. Larson, W. R. Zipfel, R. M. Williams, S. W. Clark, M. P. Bruchez, F. W. Wise and W. W. Webb, Science, 2003, 300, 1434–1436 CrossRef CAS PubMed;
(d) W. Li, M. D. Yang, L. P. Wang, W. J. Zhu, L. N. Ye, J. Y. Wu, Y. P. Tian and H. P. Zhou, Dyes Pigm., 2014, 107, 133–139 CrossRef CAS;
(e) H. J. Cui, H. Chen, Y. Pan and W. Y. Lin, Sens. Actuators, B, 2015, 219, 232–237 CrossRef CAS.
-
(a) R. H. Röckel, J. Cao, W. R. Zipfel, W. W. Webb and M. R. Hansen, Science, 1997, 276, 2039–2042 CrossRef;
(b) A. Diaspro and M. Robello, J. Photochem. Photobiol., B, 2000, 55, 1–8 CrossRef CAS;
(c) F. Helmchen and W. Denk, Nat. Methods, 2005, 2, 932–940 CrossRef CAS PubMed;
(d) N. Takahashi, Biol. Pharm. Bull., 2015, 38, 656–662 CrossRef CAS PubMed;
(e) L. Blazquez-Llorca, E. Hummel, H. Zimmerman, C. Zou, S. Burgold, J. Rietdorf and J. Herms, J. Microsc., 2015, 259, 129–136 CrossRef CAS PubMed.
-
(a) A. S. Dvornikov, E. P. Walker and P. M. Rentzepis, J. Phys. Chem. A, 2009, 113, 13633–13644 CrossRef CAS PubMed;
(b) Z. Q. Yan, B. Xu, Y. J. Dong, W. J. Tian and A. W. Li, Dyes Pigm., 2011, 90, 269–274 CrossRef CAS;
(c) K. D. Belfield and K. J. Schafer, Chem. Mater., 2002, 14, 3656–3662 CrossRef CAS;
(d) L. Li, P. Wang, Y. L. Hu, G. Lin, Y. Q. Wu, W. H. Huang and Q. Z. Zhao, Spectrochim. Acta, Part A, 2015, 139, 243–252 CrossRef CAS PubMed;
(e) R. Woods, S. Feldbacher, D. Zidar, G. Langer, V. Satzinger, V. Schmidt, N. Pucher, R. Liska and W. Kern, Opt. Mater. Express, 2014, 4, 486–498 CrossRef.
-
(a) S. Kawata, H. B. Sun, T. Tanaka and K. Takada, Nature, 2001, 412, 697–698 CrossRef CAS PubMed;
(b) B. H. Cumpston, S. P. Ananthavel, S. Barlow, D. L. Dyer, J. E. Ehrlich, L. L. Erskine, A. A. Heikal, S. M. Kuebler, I.-Y. S. Lee, D. Mccord-Maughon, J. Qin, H. Rockel, M. Rumi, X.-L. Wu, S. R. Marder and J. W. Perry, Nature, 1999, 398, 51–54 CrossRef CAS;
(c) W. Zhou, S. M. Kuebler, K. L. Braun, T. Yu, J. K. Cammack, C. K. Ober, J. W. Perry and S. R. Marder, Science, 2002, 296, 1106–1109 CrossRef CAS PubMed;
(d) E. Kapyla, T. Sedlacik, D. B. Aydogan, J. Viitanen, F. Rypacek and M. Kellomaki, Mater. Sci. Eng., C, 2014, 43, 280–289 CrossRef CAS PubMed;
(e) O. Kufelt, A. El-Tamer, C. Sehring, M. Meissner, S. Schlie-Wolter and B. N. Chichkov, Acta Biomater., 2015, 18, 186–195 CrossRef CAS PubMed.
-
(a) Y. Ren, Q. Fang, W. T. Yu, H. Lei, Y. P. Tian, M. H. Jiang, Q. C. Yang and T. C. W. Mak, J. Mater. Chem., 2000, 10, 2025–2030 RSC;
(b) A. Abbotto, L. Beverina, R. Bozio, S. Bradamante, C. Ferrante, G. A. Pagani and R. Signorini, Adv. Mater., 2000, 12, 1963–1967 CrossRef CAS;
(c) X. J. Tang, L. Z. Wu, L. P. Zhang and C. H. Tung, Chem. Phys. Lett., 2002, 356, 573–576 CrossRef CAS;
(d) Q. Chen, C. F. Zhang, B. Jiang, X. Y. Wang, Y. J. Liu, Y. Cao and M. Xiao, Opt. Express, 2012, 20, 9135–9143 CrossRef CAS PubMed;
(e) F. Todescato, I. Fortunati, S. Gardin, E. Garbin, E. Collini, R. Bozio, J. J. Jasieniak, G. Della Giustina, G. Brusatin and S. Toffanin, Adv. Funct. Mater., 2012, 22, 337–344 CrossRef CAS.
-
(a) P. A. Bouit, G. Wetzel, G. Berginc, B. Loiseaux, L. Toupet, P. Feneyrou, Y. Bretonniere, K. Kamada, O. Maury and C. Andraud, Chem. Mater., 2007, 19, 5325–5335 CrossRef CAS;
(b) I. Fuks-Janczarek, J. Ebothe, R. Miedzinski, R. Gabanski, A. H. Reshak, M. Lapkowski, R. Motyka, I. V. Kityk and J. Suwinski, Laser Phys., 2008, 18, 1056–1069 CrossRef CAS;
(c) Y. H. Jiang, Y. C. Wang, B. Wang, J. B. Yang, N. N. He, S. X. Qian and J. L. Hua, Chem.–Asian J., 2011, 6, 157–165 CrossRef CAS PubMed;
(d) R. S. Price, G. Dubinina, G. Wicks, M. Drobizhev, A. Rebane and K. S. Schanze, ACS Appl. Mater. Interfaces, 2015, 7, 10795–10805 CrossRef CAS PubMed;
(e) T. C. Lin, F. L. Guo, M. H. Li and C. Y. Liu, Chem.–Asian J., 2013, 8, 2102–2110 CrossRef CAS PubMed.
-
(a) I. Roy, T. Y. Ohulchanskyy, H. E. Pudavar, E. J. Bergey, A. R. Oseroff, J. Morgan, T. J. Dougherty and P. N. Prasad, J. Am. Chem. Soc., 2003, 125, 7860–7865 CrossRef CAS PubMed;
(b) K. Ogawa and Y. Kobuke, Anti-Cancer Agents Med. Chem., 2008, 8, 269–279 CrossRef CAS;
(c) F. S. Meng, B. Li, S. X. Qian, K. C. Chen and H. Tian, Chem. Lett., 2004, 33, 470–471 CrossRef CAS;
(d) C. Q. Tang, P. Hu, E. Ma, M. D. Huang and Q. D. Zheng, Dyes Pigm., 2015, 117, 7–15 CrossRef CAS;
(e) X. Q. Shen, S. Li, L. Li, S. Q. Yao and Q. H. Xu, Chem.–Eur. J., 2015, 21, 2214–2221 CrossRef CAS PubMed.
-
(a) Y. L. Deng, Y. H. Sun, D. X. Cao, Y. Chen, Z. Q. Liu and Q. Fang, J. Mol. Struct., 2011, 1000, 145–149 CrossRef CAS;
(b) S. Yao, K. J. Schafer-Hales and K. D. Belfield, Org. Lett., 2007, 9, 5645–5648 CrossRef CAS PubMed;
(c) Z.-Q. Liu, Q. Fang, D. Wang, D.-X. Cao, G. Xue, W.-T. Yu and H. Lei, Chem.–Eur. J., 2003, 9, 5074–5084 CrossRef CAS PubMed;
(d) Y.-C. Wang, H. Zhou, J.-L. Ding, Q. Chen, H.-B. Xiao, X.-M. Tao and S.-X. Qian, Chin. Phys. Lett., 2010, 27, 38201–38204 CrossRef;
(e) D. D. Li, Q. Zhang, X. S. Sun, N. Q. Shao, R. Li, S. L. Li, H. P. Zhou, J. Y. Wu and Y. P. Tian, Dyes Pigm., 2014, 102, 79–87 CrossRef CAS.
-
(a) C. W. Li, K. Yang, Y. Feng, X. Y. Su, J. Y. Yang, X. Jin, M. Shui, Y. X. Wang, X. R. Zhang, Y. L. Song and H. Y. Xu, J. Phys. Chem. B, 2009, 113, 15730–15733 CrossRef CAS PubMed;
(b) X. M. Wang, D. Wang, G. Y. Zhou, W. T. Yu, Y. F. Zhou, Q. Fang and M. H. Jiang, J. Mater. Chem., 2001, 11, 1600–1605 RSC;
(c) N. Xie and Y. Chen, J. Photochem. Photobiol., A, 2007, 189, 253–257 CrossRef CAS;
(d) K. D. Belfield, S. Yao, A. R. Morales, J. M. Hales, D. J. Hagan, E. W. V. Stryland, V. M. Chapela and J. Percino, Polym. Adv. Technol., 2005, 16, 150–155 CrossRef CAS;
(e) M. Rumi, J. E. Ehrlich, A. A. Heikal, J. W. Perry, S. Barlow, Z. Y. Hu, D. McCord-Maughon, T. C. Parker, H. Röckel, S. Thayumanavan, S. R. Marder, D. Beljonne and J.-L. Brédas, J. Am. Chem. Soc., 2000, 122, 9500–9510 CrossRef CAS.
-
(a) T.-H. Huang, D. Yang, Z.-H. Kang, E.-L. Miao, R. Lu, H.-P. Zhou, F. Wang, G.-W. Wang, P.-F. Cheng, Y.-H. Wang and H.-Z. Zhang, Opt. Mater., 2013, 35, 467–471 CrossRef CAS;
(b) J.-F. Xing, W.-Q. Chen, X.-Z. Dong, T. Tanaka, X.-Y. Fang, X.-M. Duan and S. Kawata, J. Photochem. Photobiol., A, 2007, 189, 398–404 CrossRef CAS;
(c) K. Susumu, J. A. N. Fisher, J. Zheng, D. N. Beratan, A. G. Yodh and M. J. Therien, J. Phys. Chem. A, 2011, 115, 5525–5539 CrossRef CAS PubMed;
(d) R. Signorini, C. Ferrante, D. Pedron, M. Zerbetto, E. Cecchetto, M. Slaviero, I. Fortunati, E. Collini, R. Bozio, A. Abbotto, L. Beverina and G. A. Pagani, J. Phys. Chem. A, 2008, 112, 4224–4234 CrossRef CAS PubMed;
(e) H. Yuan, Y. X. Zhao and F. P. Wu, Chem. Mater., 2012, 24, 1371–1377 CrossRef CAS.
-
(a) L. Porrès, C. Katan, O. Mongin, T. Pons, J. Mertz and M. Blanchard-Desce, J. Mol. Struct., 2004, 704, 17–24 CrossRef;
(b) L. Yang, F. Gao, J. Liu, X. L. Zhong, H. R. Li and S. T. Zhang, J. Fluoresc., 2011, 21, 545–554 CrossRef CAS PubMed;
(c) H. H. Chen, Z. Q. Liu, D. X. Cao, Y. L. Deng, Y. H. Sun and F. Y. Li, Dyes Pigm., 2013, 96, 563–568 CrossRef CAS.
-
(a) A. Abbotto, L. Beverina, R. Bozio, A. Facchetti, C. Ferrante, G. A. Pagani, D. Pedron and R. Signorini, Chem. Commun., 2003, 2144–2145 RSC;
(b) Q. Q. Li, J. Huang, A. Zhong, C. Zhong, M. Peng, J. Liu, Z. G. Pei, Z. L. Huang, J. G. Qin and Z. Li, J. Phys. Chem. B, 2011, 115, 4279–4285 CrossRef CAS PubMed.
-
(a) B. Dumat, G. Bordeau, E. Faurel-Paul, F. Mahuteau-Betzer, N. Saettel, M. Bombled, G. Metgé, F. Charra, C. Fiorini-Debuisschert and M.-P. Teulade-Fichou, Biochimie, 2011, 93, 1209–1218 CrossRef CAS PubMed;
(b) N. Zhao, Y. H. Zhang, X. Liu, X. Q. Yu and M. F. Ge, Chin. Sci. Bull., 2010, 55, 3661–3667 CrossRef CAS.
-
(a) G. Wang, X. H. Zhang, J. L. Geng, K. Li, D. Ding, K. Y. Pu, L. P. Cai, Y. H. Lai and B. Liu, Chem.–Eur. J., 2012, 18, 9705–9713 CrossRef CAS PubMed;
(b) G. Wang, K. Y. Pu, X. H. Zhang, K. Li, L. Wang, L. P. Cai, D. Ding, Y. H. Lai and B. Liu, Chem. Mater., 2011, 23, 4428–4434 CrossRef CAS.
-
(a) T.-C. Lin, G. S. He, Q. D. Zheng and P. N. Prasad, J. Mater. Chem., 2006, 16, 2490–2498 RSC;
(b) D. Q. Wang, X. M. Wang, Q. G. He, M. Y. Zhou, W. W. Rui, X. T. Tao, F. L. Bai and M. H. Jiang, Tetrahedron Lett., 2008, 49, 5871–5876 CrossRef CAS.
-
(a) M. Rumi, S. J. K. Pond, T. Meyer-Friedrichsen, Q. Zhang, M. Bishop, Y. D. Zhang, S. Barlow, S. R. Marder and J. W. Perry, J. Phys. Chem. C, 2008, 112, 8061–8071 CrossRef CAS;
(b) W. Zhang, Y. Z. Cui, S. J. Wang, T. D. Li and R. Sun, Acta Chim. Sin., 2009, 67, 1880–1884 CAS.
- J. Liu, K. Wang, F. Xu, Z. Tang, W. Zheng, J. Y. Zhang, C. H. Li, T. Yu and X. Z. You, Tetrahedron Lett., 2011, 52, 6492–6496 CrossRef CAS.
- Z.-F. An, R.-F. Chen, J. Yin, G.-H. Xie, H.-F. Shi, T. Tsuboi and W. Huang, Chem.–Eur. J., 2011, 17, 10871–10878 CrossRef CAS PubMed.
-
(a) W. G. Yang, P. S. Chan, M. S. Chan, K. F. Li, P. K. Lo, N. K. Mak, K. W. Cheah and M. S. Wong, Chem. Commun., 2013, 49, 3428–3430 RSC;
(b) Y.-C. Zheng, M.-L. Zheng, S. Chen, Z.-S. Zhao and X.-M. Duan, J. Mater. Chem. B, 2014, 2, 2301–2310 RSC.
-
(a) M. Charlot, N. Izard, O. Mongin, D. Riehl and M. Blanchard-Desce, Chem. Phys. Lett., 2006, 417, 297–302 CrossRef CAS;
(b) A. Bhaskar, G. Ramakrishna, Z. Lu, R. Twieg, J. M. Hales, D. J. Hagan, E. V. Stryland and T. Goodson, J. Am. Chem. Soc., 2006, 128, 11840–11849 CrossRef CAS PubMed.
- B. Strehmel, A. M. Sarker and H. Detert, ChemPhysChem, 2003, 4, 249–259 CrossRef CAS PubMed.
- M. Barzoukas and M. Blanchard-Desce, J. Chem. Phys., 2000, 113, 3951–3959 CrossRef CAS.
- M. Albota, D. Beljonne, J.-L. Brédas, J. E. Ehrlich, J.-Y. Fu, A. A. Heikal, S. E. Hess, T. Kogej, M. D. Levin, S. R. Marder, D. McCord-Maughon, J. W. Perry, H. Röckel, M. Rumi, G. Subramaniam, W. W. Webb, X.-L. Wu and C. Xu, Science, 1998, 281, 1653–1656 CrossRef CAS PubMed.
- C. Reichardt, Chem. Rev., 1994, 94, 2319–2358 CrossRef CAS.
- J. N. Demas and G. A. Crosby, J. Phys. Chem., 1971, 75, 991–1015 CrossRef.
-
(a) J. L. Wang, Z. M. Tang, Q. Xiao, Y. G. Ma and J. Pei, Org. Lett., 2009, 11, 863–866 CrossRef CAS PubMed;
(b) C.-W. Ko, Y.-T. Tao, A. Danel, L. Krzemińska and P. Tomasik, Chem. Mater., 2001, 13, 2441–2446 CrossRef CAS;
(c) W. Rettig and W. Majenz, Chem. Phys. Lett., 1989, 154, 335–341 CrossRef CAS.
- M. A. Albota, C. Xu and W. W. Webb, Appl. Opt., 1998, 37, 7352–7356 CrossRef CAS PubMed.
- M. Albota, D. Beljonne, J.-L. Brédas, J. E. Ehrlich, J.-Y. Fu, A. A. Heikal, S. E. Hess, T. Kogej, M. D. Levin, S. R. Marder, D. McCord-Maughon, J. W. Perry, H. Röckel, M. Rumi, G. Subramaniam, W. W. Webb, X.-L. Wu and C. Xu, Science, 1998, 281, 1653–1656 CrossRef CAS PubMed.
- F. Meyers, S. R. Marder, B. M. Pierce and J. L. Brédas, J. Am. Chem. Soc., 1994, 116, 10703–10714 CrossRef CAS.
- T. Kogej, D. Beljonne, F. Meyers, J. W. Perry, S. R. Marder and J. L. Brédas, Chem. Phys. Lett., 1998, 298, 1–6 CrossRef CAS.
- D. Beljonne, W. Wenseleers, E. Zojer, Z. Shuai, H. Vogel, S. J. K. Pond, J. W. Perry, S. R. Marder and J.-L. Brédas, Adv. Funct. Mater., 2002, 12, 631–641 CrossRef CAS.
-
(a) H. B. Xiao, C. Mei, Y. C. Wang, H. Li, S. X. Qian, H. Y. Yin and Z. S. Xu, Mater. Chem. Phys., 2011, 130, 897–902 CrossRef CAS;
(b) Y.-P. Tian, L. Li, J.-Z. Zhang, J.-X. Yang, H.-P. Zhou, J.-Y. Wu, P.-P. Sun, L.-M. Tao, Y.-H. Guo, C.-K. Wang, H. Xing, W.-H. Huang, X.-T. Tao and M.-H. Jiang, J. Mater. Chem., 2007, 17, 3646–3654 RSC;
(c) H. H. Chen, Z. Q. Liu, D. X. Cao, Y. L. Deng, Y. H. Sun and F. Y. Li, Dyes Pigm., 2013, 96, 563–568 CrossRef CAS;
(d) X. Y. Zhang, Z. Xu, Z. Y. Ge, X. H. Ouyang and W. Ji, J. Photochem. Photobiol., A, 2014, 290, 22–30 CrossRef CAS;
(e) L. Liu, Z. Q. Zhou, J. P. Shi, C. G. Lu, Y. P. Cui and G. Y. Lu, Chin. Chem. Lett., 2011, 22, 1147–1150 CAS;
(f) S. J. Ren, D. L. Zeng, H. L. Zhong, Y. C. Wang, S. X. Qian and Q. Fang, J. Phys. Chem. B, 2010, 114, 10374–10383 CrossRef CAS PubMed.
- F. C. Schaefer and G. A. Peters, J. Org. Chem., 1961, 26, 2778–2784 CrossRef CAS.
-
(a) H. Irngartinger and R. Herpich, Eur. J. Org. Chem., 1998, 1998, 595–604 CrossRef;
(b) K. B. Borisenko, K. Zauer and I. Hargittai, J. Phys. Chem., 1996, 100, 19303–19309 CrossRef CAS.
Footnote |
† Electronic supplementary information (ESI) available. See DOI: 10.1039/c6ra02733d |
|
This journal is © The Royal Society of Chemistry 2016 |
Click here to see how this site uses Cookies. View our privacy policy here.