DOI:
10.1039/C6RA03521C
(Paper)
RSC Adv., 2016,
6, 74833-74844
Evaluating corrosion inhibition property of some Schiff bases for mild steel in 1 M HCl: competitive effect of the heteroatom and stereochemical conformation of the molecule†
Received
6th February 2016
, Accepted 1st August 2016
First published on 1st August 2016
Abstract
To evaluate the effect produced by the heteroatom present in organic Schiff bases along with overall stereochemical conformation of such bases towards corrosion inhibition performance, three Schiff bases, namely 2-pyridyl-N-(2′-methylamino phenyl) methyleneimine (PMAM), 2-pyridyl-N-(2′-methylthiophenyl) methyleneimine (PMTM) and 2-pyridyl-N-(2′-methoxyphenyl) methyleneimine (PMPM), are assessed for mild steel in 1 M HCl. Chemical similarity exists among these three molecules, varying only in the nature of the heteroatom present away from the imine group. Results from weight loss, potentiodynamic polarization and electrochemical impedance spectroscopic (EIS) techniques demonstrate that the Schiff bases form a resistive layer on the metal surface, and thereby block the cathodic as well as the anodic reaction sites effectively. Experimentally obtained corrosion inhibition efficiency follows the sequence: PMAM > PMTM > PMPM, and this is also confirmed following surface electron microscopy (SEM) images. The Langmuir isotherm is found to provide a sufficiently good description of adsorption behavior of the compounds on the metal surface. Density functional theory (DFT) calculations and molecular dynamics (MD) simulations are done to correlate the efficiency of these compounds with their intrinsic molecular parameters, as well as energy optimized molecular orientation.
Introduction
Mild steel is the most commonly used ferrous metal. Its widespread usage is due to some unique properties that are associated with it, which include fairly high tensile strength, ductility, malleability, and most importantly it is the cheapest form of steel available. One of the main disadvantages encountered with mild steel is its susceptibility towards corrosion. Hydrochloric acid (HCl), on the other hand, is a highly corrosive, strong mineral acid with a wide array of industrial applications. With growing industrial activities worldwide, the study of the corrosion process of mild steel in several acidic media, including HCl, and its mitigation has now gained tremendous impetus. Among many available methods to restrict the acid induced metal degradation, application of organic corrosion inhibitors has now become a vibrant field of research and great numbers of scientific studies are devoted to this subject.1–6 Presence of heteroatoms, such as O, N, S or P, as well as unsaturated bonds allows organic inhibitors to get adsorbed on the metal surface and thereby help in reducing the aggressiveness of acid attack on it.7–12 Several reports suggest the inhibitory effect of these heteroatoms follow the order: P > S > N > O.13,14
Among several classes of organic inhibitors, Schiff bases are very popular which may be attributable to the ease of synthesis from relatively inexpensive starting-materials and their eco-friendly or low toxic properties.15,16 The high inhibitory performance of these compounds results from the presence of different heteroatoms (e.g. N, O, Cl, Br) and π electrons in their structure, and most importantly the imine functional group.17–21 The present work intends to study inhibitive performance of three Schiff bases, namely, 2-pyridyl-N-(2′-methylamino phenyl) methyleneimine (PMAM), 2-pyridyl-N-(2′-methylthiophenyl) methyleneimine (PMTM) and 2-pyridyl-N-(2′-methoxyphenyl) methyleneimine (PMPM) (Table 1) in towards corrosion of mild steel in 1 M HCl solution employing electrochemical as well as weight loss methods. These inhibitors are having structural similarity, only differing in the heteroatom at the 2′-substituent of phenyl ring. How these heteroatoms, together with the stereochemical conformation, are changing the intrinsic molecular parameters of the molecules, which in-turn influence their adsorption and subsequent corrosion inhibition properties, are being explored here. For this purpose, various molecular parameters like energy of the frontier molecular orbitals (HOMO and LUMO) in energy optimized geometry, electronegativity, global hardness and softness, fraction of electron transferred have been determined by density functional theory (DFT) calculations. Local reactive sites of the inhibitor molecules are analyzed through Fukui indices. Moreover, MD simulation is employed to ascertain the adsorption behaviour of inhibitors on Fe (1 1 0) surface. Surface morphology of the corroded metal surface in absence and presence of inhibitors are assessed by surface electron microscopy (SEM) images.
Table 1 Structure, abbreviation and characteristics of Schiff-bases
Molecules |
Structural formula |
Melting point |
Characteristic IR peaks (cm−1)23 |
ESI-MS (m/z): [L–H+] (amu) |
2-Pyridyl-N-(2′-methylaminophenyl) methyleneimine (PMAM) |
 |
157 °C |
1647 (C N) 1280 (C–N str. for aromatic secondary amine) |
212.1 |
2-Pyridyl-N-(2′-methylthiophenyl) methyleneimine (PMTM) |
 |
185 °C |
1622 (C N) 690 (C–S str. for aryl thioethers) |
229.2 |
2-Pyridyl-N-(2′-methoxyphenyl) methyleneimine (PMPM) |
 |
168 °C |
1624 (C N) 1220 (aryl-O str.) |
213.1 |
Experimental details
Synthesis of inhibitors
Inhibitors PMAM, PMPM and PMTM are synthesized as per reported procedure (schematically it is represented in Scheme 1).22 For preparing PMAM, PMTM and PMPM, methanolic solution of pyridine-2-carboxaldehyde is added to either of N-methylphenylenediamine or 2-methyl thioaniline or o-anisidine, respectively (1
:
1 mole ratio) and refluxed under nitrogen for 6 h. After cooling to room temperature, the solvent is removed completely and the thick oily residue is dissolved in CHCl3 and washed successively with distilled water, brine solution and finally again with distilled water. The organic layer is then separated out and dried over anhydrous Na2SO4 for 1 h, filtered and solvent is removed completely. The oily residue is kept at 4 °C for overnight to get solid residue, which is then recrystallized from diethyl ether. These compounds are characterized by melting point measurement, molecular ion peak as obtained in the corresponding mass spectrum (Advion make compact mass spectrometer, serial no. 3013-0140) and FT-IR (Thermo-Nicolet iS10 IR spectrometer in the range 4000–400 cm−1) (Table 1, Fig. 1 and S1, ESI†). Existence of the molecular ion peak in the corresponding mass spectrum confirms the formation of the inhibitor molecules (supported vide huge abundance ratio in ESI-MS).
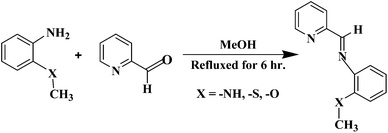 |
| Scheme 1 Mechanistic pathway for synthesis of Schiff-bases. | |
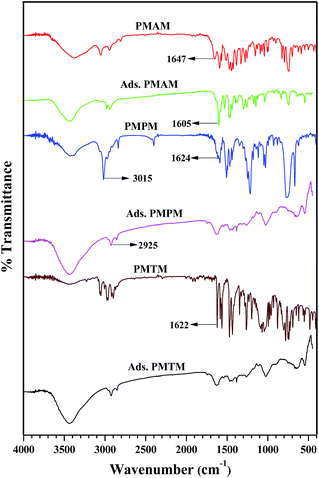 |
| Fig. 1 FTIR spectra of the synthesized Schiff base derivatives and their surface adsorbed forms. | |
Electrochemical measurements
Potentiodynamic polarization and electrochemical impedance measurement are done by conventional three-electrode system (model: Gill AC, ACM Instruments, UK) consisting of mild steel working electrode (WE) with an exposed area of 0.25 cm2, platinum as counter electrode and saturated calomel electrode (SCE) as reference. Volume of HCl solution taken for each experiment is 200 mL. Before electrochemical tests, the WE is kept in the test solution for 45 min and the rest potential was checked for next 5 min (Fig. S2, ESI†). No substantial variation of rest potential confirms that the steady state is reached. Polarization curves are recorded within a potential ranged from −250 to +250 mV with respect to rest potential at constant sweep rate of potential 0.5 mV s−1, which is proved sufficient to meet the demand of Tafel extrapolation tests of mild steel in 1 M HCl. Inhibition efficiency, ηP (%) is defined as: |
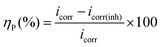 | (1) |
where, icorr and icorr(inh) are the values of corrosion current density of uninhibited and inhibited specimens, respectively.
Electrochemical impedance spectroscopy (EIS) measurements are performed at the rest potential over the frequency range 100 kHz to 10 mHz using 10 mV (rms) voltage excitation. Inhibition efficiency, ηz (%) is defined as:
|
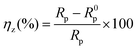 | (2) |
where,
Rp and
R0p are the values of polarization resistance in presence and absence of inhibitor.
Rp represents the cumulative effect arising due to different resistances prevailing at the metal–electrolyte interface, namely, charge transfer resistance, resistance due to adsorbed corrosion products as well as inhibitors. All tests are carried out under unstirred conditions at room temperature of around 30 °C.
Weight loss measurements
The weight loss tests are performed on mild steel coupons (wt% composition: 0.19 C, 0.21 Si, 0.21 Mn, 0.01 P, 0.01 S and the remainder iron) with dimensions of 2.5 × 2.5 × 0.1 cm3. Metallic specimens are polished with metallurgical grade emery papers of 400–1600 grid, washed thoroughly with acetone and distilled water. Clean and dried coupons are weighed and then immersed in 75 mL of 1 M HCl, taken in a graduated 100 mL beaker covered with a watch glass, without and with the presence of inhibitor molecules having 10 mM concentration for 1 to 96 h of duration at room temperature of around 30 °C. Very small change in volume of the electrolytic solution during immersion period is compensated by adding requisite amount of distilled water. Metallic coupons are then removed, scales formed on the surface are cleaned with bristled brush, washed, dried in a vacuum desiccator and final weights are recorded. Percentage inhibition efficiency, ηW (%) is calculated following the relation: |
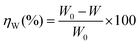 | (3) |
where, W0 and W are the weight loss of the metal coupons in acid medium without and with inhibitor.
All the electrochemical and gravimetric measurements are carried out thrice and the values of inhibition efficiencies obtained for three different sets of experiment are found to be very close (within a range of ±1 to 2%).
Surface morphology
For morphological studies, the mild steel surface is prepared by keeping the samples for 6 h in 1 M HCl in the absence and presence of organic inhibitors at 10 mM concentration. The specimens are then washed gently with water, carefully dried and analyzed by a scanning electron microscopy using a Hitachi S-3000N microscope.
Computational details for quantum chemical calculation
DFT calculations are performed in this present study with the ORCA programme (version 2.7.0).24 This is an open source code developed by Prof. Dr Franc Neese (Director, MPI für Chemische Energiekon version, Muelheim, Germany). Geometry optimizations of all inhibitor molecules are performed with B3LYP functional level25,26 of DFT employing triple-ζ quality basis sets TZV(P) with one set of polarization functions on the atoms like N, O and S.27 For carbon and hydrogens like atoms, slightly smaller polarized split-valence SV(P) basis sets are used which are double-ζ quality in the valence region and have polarizing set of d functions on atoms other than hydrogens. SCF calculations are converged tightly (1 × 10−8 Eh in energy, 1 × 10−7 Eh in the density and 1 × 10−7 in maximum element of the DIIS error vector). Theoretical parameters are calculated in the liquid phase because electrochemical corrosion always happens in liquid phase. Therefore, it is necessary to include the effect of a solvent in the computational outcomes. This method models the solvent as a continuum of uniform dielectric constant (ε) where solute is placed as a uniform series of inlocking atomic spheres.
Following Koopmans' theorem, different intrinsic molecular quantities like electronegativity (χ, a measure of the power of a group of atoms to attract electrons towards itself), global hardness (η, a parameter related to the resistance of an atom to a charge transfer) and global softness (σ, which shows the reactivity of the inhibitor molecules in terms of charge transfer), etc., which are supposed to influence the overall reaction between two interacting systems, have been calculated.28–30 These quantities are related to electron affinity (A) and ionization potential (I) as follows:
From these values, the fraction of electrons transferred from the inhibitor molecule to the metallic atom (ΔN) is calculated. As per its underlying concept, during the reaction of two systems having different electronegativity (here, the metallic surface and an inhibitor molecule) the following mechanism will take place: the electron flow will happen from the molecule with lower electronegativity towards that of a higher value, until the chemical potentials become the same. In order to calculate the fraction of electrons transferred, a theoretical value for the electronegativity of bulk iron was used χFe = 7 eV,31 and a global hardness of ηFe = 0, by assuming that for a metallic bulk I = A.32 The fraction of electrons transfers to the metallic surface is calculated as follows:33
|
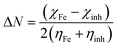 | (9) |
The local reactivity of the molecules is analyzed through an evaluation of the Fukui indices using Dmol3 module, Material studio™ version 6.1 by Accelrys Inc., San Diego, CA. All the calculation are performed applying BLYP exchange correlation functional and the double numeric with polarization (DNP) basis set, because this set is the best set available in Dmol3. Fukui indices are used to measure the chemical reactivity, as well as an indicative of the reactive regions and the nucleophilic and electrophilic behaviour of the molecule. The regions of a molecule where the Fukui function is large are chemically softer than the regions where the Fukui function is small, and by invoking the HSAB principle in a local sense, one may establish the behaviour of the different sites with respect to hard or soft reagents. The Fukui function fk is defined as the first derivative of the electronic density ρ(
) with respect to the number of electrons N in a constant external potential v(
) (eqn (10)).34
|
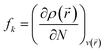 | (10) |
The Fukui functions can be written by taking the finite difference approximations as:35
|
fk+ = qk(N + 1) − qk(N), (for nucleophilic attack)
| (11) |
|
fk− = qk(N) − qk(N − 1), (for electrophilic attack)
| (12) |
where
qk is the gross charge of
kth atom in the molecule. The
qk(
N + 1),
qk(
N) and
qk(
N − 1) are defined as the charge of the anionic, neutral and cationic species, respectively, in their energy optimized electronic state. Here Fukui functions are obtained through the finite difference approximation using Hirschfeld population analysis (HPA).
36
Molecular dynamics simulation
Adsorption behaviour of inhibitor molecule is very much important to understand the corrosion behaviour on metal surface. Molecular dynamics (MD) simulation is the technique, which has become popular towards finding the preferential adsorption sites on metal surfaces. The molecular dynamics (MD) simulations are performed using the software, studio™ 6.1. Fe (1 1 0) surface is chosen for the simulation study. The MD simulation of the interaction between inhibitor molecules and Fe (1 1 0) surface is carried out in a simulation box (36.83 Å × 36.83 Å × 77.36 Å) with periodic boundary conditions to model a representative part of the interface devoid of any arbitrary boundary effects. The box consisted of a Fe slab, a water slab (150H2O molecules) containing the studied compounds and a vacuum layer. During simulations, all the bulk atoms in the Fe (1 1 0) systems were kept “frozen”, and the inhibitor molecules and water molecules are allowed to interact with the metal surface freely. The MD simulation is performed under 298 K, NVT ensemble, with a time step 1.0 and simulation time of 100 ps using the COMPASS force field. The interaction energy, Einteraction between the Fe (1 1 0) surface and the inhibitor molecule is calculated according to the following equation:37,38 |
Einteraction = Etotal − (Esurface+water + Einh(solv))
| (13) |
where, Etotal is the total energy of the simulation system, Esurface+water is the energy of iron surface together with H2O molecules, and Einh(solv) is the energy of the solvent stabilized inhibitor molecule. These individual energy components are determined by selecting the respective constituents from the simulation box under final equilibrated state (presented pictorially in Fig. S3, ESI†). It is worth mentioning here that there are certain limitations associated with the single point energy calculation as done in the present MD simulation method, such as considering relatively less number of water molecules, not considering the acidic component in the water, etc. As a consequence, the MD simulation result is approximated and may be regarded as a supportive result for the experimentally obtained findings.
Results
Polarization measurements
The current–potential relationships for mild steel electrode in 1 M HCl without and with PMAM, PMTM and PMPM at 10 mM concentration at 303 K are shown in Fig. 2. Such polarization curves for each inhibitor molecule at different concentrations are included in Fig. S4(A–C), ESI.† Different parameters obtained from polarizations study, like, corrosion potential (Ecorr), corrosion current density (icorr), anodic and cathodic Tafel slopes (ba and bc) are shown in Table 2. It is observed that, both the cathodic and anodic currents are gradually shifting towards lower value for the whole potential range with increasing concentration of all the three inhibitors. In presence of the inhibitors, no systematic variation of anodic and cathodic Tafel slopes with inhibitor concentration is found. Overall, both are increased from those observed in the blank solution at lower overpotential range (as evident from Fig. S5, ESI†). This indicates relatively smaller change of anodic and cathodic currents with polarization compared to that when no inhibitor is present. Following Bockris et al., increase in Tafel slope may be attributed to either adsorption of organic impurities or the presence of conducting films on the electrode surface.39 In the present case, overall increase in anodic and cathodic Tafel slopes may arise from the adsorption of inhibitor molecules on both the cathodic and anodic reaction sites, and thereby, retarding rate of the corresponding reactions. Such simultaneous adsorptive nature of inhibitors may be responsible for irregular variation of Tafel slopes, which is also been reported by many others for similar systems.1–16 Mixed-type corrosion inhibition property of the three inhibitors is also evident from the observed irregular variation of Ecorr values as a function of inhibitor concentration. Further, with respect to the uninhibited sample, in presence of PMTM and PMPM, Ecorr values show a definite tendency of shifting towards more negative direction. All these observations suggest that though the Schiff bases used in this present study act, in general, as mixed type inhibitors, for PMTM and PMPM, effect of diminishing the rate of cathodic reduction reaction (hydrogen evolution) is somewhat more prominent than that of the anodic metal oxidation reaction. Maximum decrease in icorr is observed in case of PMAM indicating that it is the most effective corrosion inhibitor among the studied Schiff base molecules.
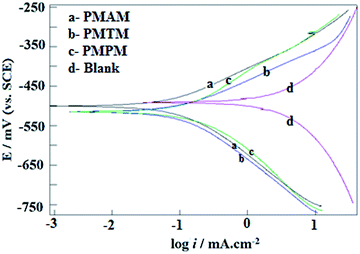 |
| Fig. 2 Potentiodynamic polarization curves for mild steel in 1 M HCl in the absence and presence of Schiff-bases having 10 mM concentration. | |
Table 2 Data from potentiodynamic polarization studies for mild steel in 1 M HCl in various inhibitor systems
System |
Conc./mM |
−Ecorr (mV per SCE) |
icorr (μA cm−2) |
ba (mV dec−1) |
−bc (mV dec−1) |
ηP (%) |
Blank |
|
492 |
1200 |
62 |
78 |
|
PMAM |
0.10 |
505 |
378 |
90 |
105 |
69 |
0.50 |
500 |
255 |
79 |
113 |
79 |
1.00 |
503 |
170 |
82 |
107 |
86 |
5.00 |
502 |
104 |
71 |
106 |
91 |
10.00 |
500 |
65 |
72 |
104 |
95 |
PMTM |
0.10 |
505 |
657 |
84 |
122 |
45 |
0.50 |
504 |
340 |
92 |
120 |
72 |
1.00 |
502 |
232 |
91 |
103 |
80 |
5.00 |
503 |
132 |
82 |
125 |
89 |
10.00 |
515 |
100 |
74 |
127 |
92 |
PMPM |
0.10 |
497 |
709 |
82 |
101 |
41 |
0.50 |
499 |
399 |
89 |
108 |
66 |
1.00 |
494 |
325 |
83 |
130 |
73 |
5.00 |
515 |
183 |
99 |
104 |
85 |
10.00 |
514 |
124 |
96 |
110 |
90 |
Electrochemical impedance spectroscopy (EIS)
For mild steel, Nyquist plots and Bode diagrams obtained from EIS in the absence and presence of three inhibitors (e.g.; PMAM, PMTM and PMPM) in 10 mM concentration are shown in Fig. 3A and B, respectively, while Nyquist plots for all the three inhibitors with different concentrations are given in Fig. S6(A–C), ESI.† For all the three inhibitors with varied concentration, the shape of the impedance diagrams are maintained comparing with that of blank solution, suggesting almost no change in the corrosion mechanism due to inhibitor addition.40 The capacitive loops in absence and presence of inhibitors exhibit only one semicircle in the Nyquist plot, corresponding to one time constant. Bode diagrams also exhibit one negative fluctuation only. These indicate that corrosion inhibitive effect by the studied Schiff bases is mostly polarization resistance controlled. Polarization resistance comprises various resistive effects acting at the metal–solution interface, which include charge transfer resistance, resistance due to the adsorbed organic inhibitors, as well as corrosion products. It is further noticed that in the Nyquist plots, the obtained semicircle is depressed under the real axis, which may be attributable to the roughness and inhomogeneity of the electrode surface.41,42 Considering all these facts, the impedance plots are fitted with the equivalent circuit having one time constant, as depicted in Fig. S7 (ESI†). In Nyquist plots, the polarization resistance (Rp) stands by difference in real impedance in lower and higher frequencies. Thus, larger the diameter of the capacitive loop in Nyquist plot, higher is the Rp. It is seen that with increase in inhibitor concentration, diameter of the capacitive loop, and thus Rp as well as ηz (%) increases. These observations suggest that extent of adsorption of the inhibitors increases with concentration and thereby provides better barrier towards charge transfer reactions at the metal–solution interface. The constant phase element (CPE), used in the equivalent circuit, is related to the double layer capacity (Cdl) and its impedance is given by:where, Q is a proportionality coefficient, ω is the angular frequency, n is a measure of surface irregularity.41,42 For whole numbers of n = 1, 0, −1, CPE is reduced to the classical lumped elements capacitor (C), resistance (R), and inductance (L), respectively. To obtain a direct correlation between polarization resistance (Rp) and double layer capacitance (Cdl), the later has been recalculated using the following equation:43,44
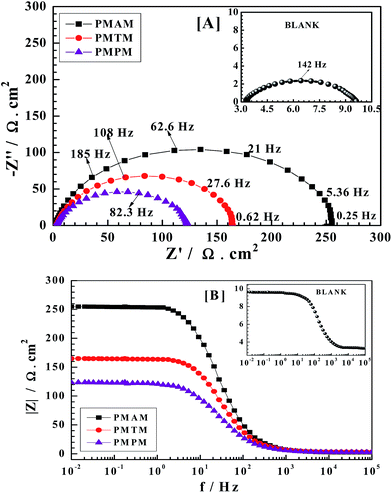 |
| Fig. 3 Electrochemical impedance curves for mild steel in 1 M HCl in the absence and presence of 10 mM concentration of PMAM, PMTM, PMPM exemplified as: (A) Nyquist and (B) Bode plots. | |
All the fitted parameters, together with ηz (%) values are tabulated in Table 3. Capacitors oppose or impede the current flow. Thus decrease in Cdl values with concomitant increase in Rp for gradual increase in inhibitor concentration clearly indicates towards corrosion inhibitive performance of the inhibitors by virtue of enhanced degree of surface coverage, as well as increasing thickness of the adsorbed layer. It is also seen that the addition of inhibitors in aqueous HCl solution brings about an irregular variation in the values of solution resistance (Rs). Same phenomenon is reported by many others.45 Generally, increase in the concentration of Schiff bases, which are non-electrolytic in nature, should result in increasing the Rs values. Thus to explain the observed irregular variation, some competing factor should be taken into consideration. Since in aqueous solution, H+ and Cl− ions remain in heavily solvated ion-pair form, so the movement of one ion gets affected or hindered by the other. Schiff bases readily get protonated in aqueous acidic solution. Due to large size of the protonated forms of these Schiff bases, their charge densities become lower, resulting relatively weaker interaction with the counter negative Cl− ions. Thus the ions can migrate comparatively more freely in aqueous solution, resulting in a decrease in Rs values. Mutual effect of these two opposing factors is most likely being responsible for the observed irregular variation of Rs with varying concentration of the Schiff bases studied.
Table 3 Impedance parameters for the corrosion of mild steel in 1 M HCl in various inhibitor systems
System |
Conc. (mM) |
Rs (Ω cm2) |
Rp (Ω cm2) |
Q (μΩ−1 sn cm−2) |
Cdl (μF cm−2) |
n |
ηz (%) |
θ |
Blank |
|
3.28 |
6.3 |
593 |
195 |
0.834 |
— |
— |
PMAM |
0.10 |
2.71 |
32 |
256 |
86.0 |
0.815 |
80 |
0.80 |
0.50 |
2.21 |
50 |
209 |
76.3 |
0.819 |
87 |
0.87 |
1.00 |
2.35 |
88 |
146 |
65.3 |
0.844 |
93 |
0.93 |
5.00 |
1.75 |
124 |
90.4 |
44.3 |
0.863 |
95 |
0.95 |
10.00 |
2.15 |
252 |
63.8 |
37.7 |
0.887 |
98 |
0.98 |
PMTM |
0.10 |
3.50 |
17 |
348 |
90.5 |
0.792 |
63 |
0.63 |
0.50 |
3.23 |
39 |
252 |
77.6 |
0.797 |
84 |
0.84 |
1.00 |
2.00 |
54 |
206 |
72.2 |
0.811 |
88 |
0.88 |
5.00 |
2.18 |
89 |
143 |
52.8 |
0.812 |
93 |
0.93 |
10.00 |
3.08 |
161 |
85.7 |
41.9 |
0.857 |
96 |
0.96 |
PMPM |
0.10 |
3.08 |
13 |
378 |
97.6 |
0.797 |
52 |
0.52 |
0.50 |
3.60 |
29 |
259 |
87.9 |
0.819 |
78 |
0.78 |
1.00 |
3.56 |
42 |
213 |
76.7 |
0.822 |
85 |
0.85 |
5.00 |
3.02 |
78 |
178 |
67.9 |
0.816 |
92 |
0.92 |
10.00 |
2.61 |
121 |
135 |
51.7 |
0.811 |
95 |
0.95 |
Further, the inhibitive performance is observed to vary in the series: PMAM > PMTM > PMPM; i.e., the same trend as observed in the polarization measurement study. This may also be confirmed from the corresponding Bode plots (Fig. 3B), where impedance moduli for different inhibitors are seen to follow the above mentioned order for the whole frequency range.
Weight loss measurement
The relative performance among the experimental Schiff bases towards inhibition of corrosion of mild steel in 1 M HCl is assessed following weight loss measurement (Fig. 4). Data from weight loss measurement also confirms the performance trend as obtained from electrochemical tests. Additionally, it shows that inhibitory action for all the three inhibitors lasts for considerable time of exposure in acid solution. This suggests towards strong bond formation between the Schiff base and metal surface, that results into a durable inhibitory film formation on the later.
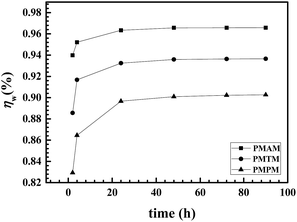 |
| Fig. 4 Variation of inhibition efficiency of PMAM, PMTM, PMPM having 10 mM concentration at different immersion times towards corrosion of mild steel in 1 M HCl by weight loss measurement. | |
Adsorption isotherm
To understand the mechanism of corrosion inhibition of mild steel in 1 M HCl by the studied Schiff bases, the adsorption behaviour of these derivatives on the mild steel surface must be known. If simple adsorptive behaviour is assumed for these derivatives, a direct relationship between inhibition efficiency and surface coverage, θ should exist. EIS data are used to evaluate the surface coverage values, which is based on the relation:46–49 |
 | (16) |
Among several isotherms, Langmuir adsorption isotherm has been proved to be best fitted to interpret the observed data in this present study. Here, concentration of adsorbent (C) is related to the degree of surface coverage (θ) following the relation:
|
 | (17) |
where,
Kads is the constant of adsorption. Excellent linear fittings of the plots of (
C/
θ) against
C are given in Fig. S8(A–C), ESI,
† with good correlation coefficient (
r2 = 0.999) and nearly unit slope, justifies the applicability of the Langmuir model. The adsorption equilibrium constant (
Kads) is related to the standard free energy of adsorption, Δ
G°ads by following equation:
|
ΔG°ads = −RT ln(55.55Kads)
| (18) |
where, 55.55 is the molar concentration of water,
R is the universal gas constant and
T be the temperature (here, 303 K). Corresponding adsorption parameters for PMAM, PMTM and PMPM are given in
Table 4. The large negative values of Δ
G°ads indicate that the studied Schiff bases are adsorbed by an appreciable extent on the mild steel surface at equilibrium. Many references suggest that value of Δ
G°ads around −20 kJ mol
−1 may be ascribed to the electrostatic interaction between charged organic molecules and charged metal surface (
i.e., physisorption).
44,46–49 Whereas, that of the order of −40 kJ mol
−1 or higher involves charge sharing or a transfer between the inhibitor molecule and the metal surface to form a co-ordinate type of bond (
i.e., chemisorption).
44,46–49
Table 4 Adsorption parameters for Schiff bases on mild steel in 1 M HCl
Sample |
r2 |
Kads (M−1) |
−ΔG°ads (kJ mol−1) |
PMAM |
0.999 |
15.17 × 103 |
34.39 |
PMTM |
0.999 |
10.69 × 103 |
33.50 |
PMPM |
0.999 |
7.84 × 103 |
32.72 |
Observed range of free energies in the present study suggests adsorption the Schiff bases on Fe-metal surface is of mixed type, with chemisorption being more dominating over physisorption.
Surface morphology
SEM images of the corroded metal surface in absence and presence of inhibitors are shown in Fig. 5. All the three inhibitors are shown to impart considerable effect in inhibiting corrosion of mild steel in compared to that in uninhibited sample. But, among the three corrosion inhibitors, PMAM stands undoubtedly as the best one.
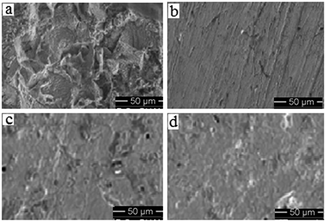 |
| Fig. 5 SEM images of mild steel surface after immersion for 6 h in 1 M HCl having (a) no inhibitor, and having 10 mM of (b) PMAM, (c) PMTM and (d) PMPM. | |
FTIR spectroscopic studies of the adsorbed layer
In order to ascertain if the inhibitor molecules get adsorbed on the mild steel surface, FTIR spectroscopic studies are done with the surface adsorbed layer and these are compared with those of the prepared Schiff bases (Fig. 1). Prior to measurement, cleaned mild steel coupons remain exposed in Schiff base solutions (10 mM concentration) prepared in 1 M HCl for 24 h. Coupons are then washed repeatedly with distilled water and dried under vacuum desiccator. Surface adsorbed layer is collected by scratching it using a knife. FTIR spectra of adsorbed layers contain almost all the characteristic bands in the fingerprint region (1500–500 cm−1) along with vibrational bands corresponding to imine group (1650–1620 cm−1) as well as stretching vibration of aromatic C–H (3100–2950 cm−1) and methyl C–H groups (2950–2850 cm−1) (Fig. 1). Comparing all the FTIR spectra, it is revealed that the imine band of PMAM at 1647 cm−1 undergoes a prominent red shift at 1605 cm−1, whereas for other two Schiff bases these bands get broadened upon adsorption. Adsorption of Schiff bases are also seen to shift the aromatic C–H and methyl C–H vibrational frequencies towards lower wavenumber region. All the information indicates clearly towards adsorption of inhibitor molecules on the mild steel surface, which ultimately leads towards inhibition of corrosion of the latter in acid medium. Similar inferences towards adsorption of inhibitors on metal, following observation of FTIR spectra, have been made by many other researchers.50–52
Molecular orbital energy level calculations
Electron distribution in HOMO and LUMO energy levels for the energy optimized neutral inhibitor molecules in aqueous solution are shown in Fig. 6 and the corresponding energies are tabulated in Table 5. It is well documented that formation constant for protonated Schiff bases is much less than the formation constant of metal–Schiff base complexes.53 Thus it is logical to assume that though Schiff bases exist preferentially in protonated form in acidic medium, but it is the neutral form which is mostly responsible towards interacting with the metal surface. Generally, EHOMO and ELUMO are related to the susceptibility of the molecule to be attacked by electrophiles and nucleophiles, respectively. Higher the value of EHOMO is, stronger is the electron donating ability of the molecule. Whereas, the lower value of ELUMO signifies the molecule to be susceptible to accept electrons.54–56 And the molecule with lower absolute values of the energy band gap (ΔE = ELUMO − EHOMO) exhibits higher inhibition efficiencies because of the possibility of both ways of electron transfer, i.e., inhibitor to metal and vice versa. From Table 5, it is seen that the EHOMO values are decreasing in the order PMAM > PMTM > PMPM, whereas, the same order represents an increase in the values of both the ELUMO and ΔE. This manifests that the extent of bonding (inhibitor to metal and back) between the inhibitor and metal is maximum for PMAM and decreases following the above order. This result derived from quantum chemical calculation conforms to the experimentally obtained one. Electron transfer will occur from inhibitor molecule to metal surface if ΔN > 0 and vice versa for ΔN < 0.57,58 According to Elnga et al., inhibition efficiency increases with increasing electron-donating ability of the molecule at the metal surface if ΔN < 3.6.59 Inspection of Table 5, shows that all the values of ΔN are positive and less than 3.6, indicating that the overall inhibitor–metal interaction is dominated by donation of electron from inhibitor molecule to the Fe surface facilitating the formation of coordinate bond and hence, the corrosion inhibition. Values of ΔN for the three Schiff bases further support the experimentally obtained trend of inhibition efficiency.
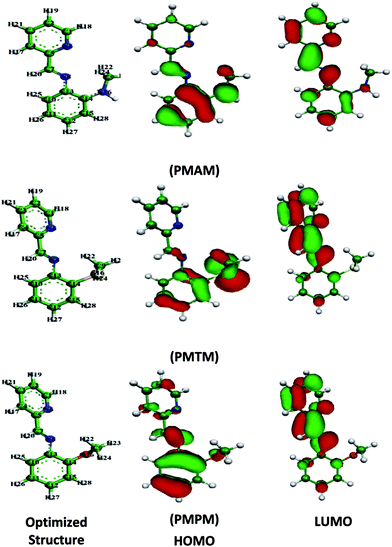 |
| Fig. 6 Optimized molecular structures of Schiff bases with electronic distribution in HOMO and LUMO. | |
Table 5 Calculated quantum chemical parameters for used Schiff-bases obtained from DFT method
Inhibitors |
EHOMO (eV) |
ELUMO (eV) |
ΔE (eV) |
I (eV) |
A (eV) |
χ (eV) |
H (eV) |
σ (eV−1) |
ΔN |
PMAM |
−6.1096 |
−2.1202 |
3.9894 |
6.1096 |
2.1202 |
4.1134 |
1.9947 |
0.5013 |
0.7236 |
PMTM |
−6.1303 |
−2.0164 |
4.1139 |
6.1303 |
2.0164 |
4.0734 |
2.0569 |
0.4862 |
0.7114 |
PMPM |
−6.1519 |
−1.9471 |
4.2108 |
6.1519 |
1.9471 |
4.0495 |
2.1054 |
0.4749 |
0.7006 |
On the other hand, electronegativity (χ), implies electron attracting capability of the molecule. Higher the electronegativity, stronger will be the attracting power to accept electron from the metal surface. Therefore, those inhibitor molecules which possess higher electronegativity would have strong interaction with metal surface and higher inhibition efficiency is expected. From the values of different molecular parameters (Table 5), it is seen that electronegativity of three inhibitor molecules follows the trend PMAM > PMTM > PMPM. Therefore, from this result it is confirmed that PMAM has the highest ability to accept electrons among the three inhibitor molecules.
Global softness (σ) is another important issue concerning the adsorption of inhibitor molecule on metal surface. Adsorption is preferable where comparatively higher softness is acquired. In this study we may consider inhibitor as a soft base and metal surface as a soft acid.54 The reported σ values (vide Table 5) follow the trend PMAM > PMTM > PMPM, which confirms the inhibition efficiency is in the expected order. So, the theoretical outcomes again match well with the experimental findings.
Local reactivity of the corrosion inhibitors
To establish the active site of an inhibitor molecule, three influencing and controlling factors; namely, neutral atomic charge, distribution of frontier molecular orbital and Fukui indices have to be considered. Local reactivity is analyzed by means of the condensed Fukui function.60–62 Condensed Fukui functions allow us to distinguish each part of the molecule on the basis of its distinct chemical behaviour due to the different substituent functional groups. The nucleophilic and electrophilic attack is controlled by the maximum values of fk+ and fk−, respectively. The calculated Fukui indices for nucleophilic and electrophilic attack for the three selected inhibitors are tabulated in Table S1 (ESI†). Regarding to adsorption phenomenon, high value of fk− on any atom present in the inhibitor molecule indicates that the atom prefers to form a chemical bond by donation of electron to the metal, i.e., the atom is more susceptible towards electrophilic attack. Similarly, an atom with a high value of fk+ is said to be responsible for bond formation by accepting an electron from the metal (i.e., back bond or retro donation). For all the three inhibitors, fk− values are seen to be higher at imine C and N atoms, C atoms of the phenyl ring, heteroatom and C atom of the substituent group of the phenyl ring. fk+ values, on the other hand, are observed to be mostly on imine C and N atoms, C and N atoms of pyridine group. These are largely in accordance with the observed electronic distribution of HOMO and LUMO energy levels of the inhibitors. Most striking observation in terms of local reactivity analysis reveals that though the S16 atom present in PMTM possesses the largest fk− value, which should make PMTM the best inhibitor among all the three inhibitors studied, PMAM containing N16 atom with relatively much lower fk− value has come out as the best. Table S1 (ESI†) shows that the lower fk− value of N16 atom in PMAM, compared to that of S16 atom in PMTM, is compensated by the relatively high fk− values of the C atoms of its phenyl ring. fk− values of the C atoms of the phenyl ring for PMPM are seen to be relatively higher than those for both the PMAM and PMTM, but O16 atom in PMPM has very low fk− value than S16 atom of PMTM or N16 atom of PMAM. As far as fk+ values are concerned, it is observed that all the corresponding atoms present in the three different inhibitors have very comparable values.
Molecular dynamics (MD) simulation
MD simulation studies further corroborated the experimental observation regarding the relative anti-corrosive propensity the three inhibitors studied. MD simulation results under equilibrium condition in terms of fluctuation in temperature and energy predicts reasonably the most favourable configuration of the adsorbed inhibitor on the metal surface. Under this condition, Einteraction between the inhibitor and Fe (1 1 0) surface can be provided according to the eqn (13) and the corresponding values are summarized in Table 6. The best adsorption configuration of the inhibitor over Fe (1 1 0) surface as well as the close contacts between those are depicted in Fig. 7. The strong adsorption between the inhibitors and the iron surface are reflected from their high negative interaction energy values. Among the three inhibitors, PMAM shows the highest value of interaction energy, while PMPM showing the least (Table 6). Further, estimation of bond distances among the atoms of the inhibitor molecules and the metal surface reveals that almost all the atoms lie within a range of 2.5 to 3.5 Å from the metal surface. This reflects strong adsorption property for all the inhibitors on the Fe (1 1 0) surface in aqueous medium. Following literature value, it can be argued that the obtained range for bond distance represents the formation of covalent bond which occurs mostly due to chemisorption.62,63 All these results are in good agreement with the results obtained from experimental work as well as from quantum chemical calculations.
Table 6 Output obtained from MD simulation for adsorption of inhibitors on Fe (1 1 0) surface
Systems |
Einteraction (kJ mol−1) |
Fe (1 1 0) + PMAM + H2O |
−529.476 |
Fe (1 1 0) + PMTM + H2O |
−522.577 |
Fe (1 1 0) + PMPM + H2O |
−494.762 |
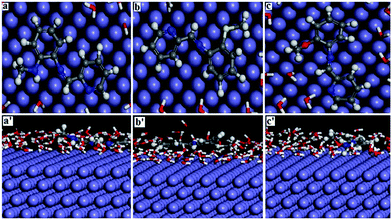 |
| Fig. 7 Equilibrium adsorption configurations of inhibitors PMAM (a and a′), PMTM (b and b′) and PMPM (c and c′) on Fe (1 1 0) surface obtained by molecular dynamics simulations. Top: top view, bottom: side view. | |
Discussion
From the EHOMO and ELUMO trend, it is clear that the binding pattern is same between the three inhibitor molecules and the metallic surface, i.e., electron transfer from inhibitor to metal as well as metal to inhibitor, both are operating during adsorption process. But the extent of such interaction varies from molecule to molecule. In general, lone pair of electrons of nitrogen, oxygen or sulfur atom together with π-electrons of imine group (C
N), pyridine and benzene ring can interact with vacant low lying 3d orbitals of Fe. On the other hand, 4s electrons present in Fe can form coordinate bond with the LUMO of the inhibitors. From the torsion angle value (Table 7), it is clear that PMAM has almost planar structure. This provides PMAM an ideal geometry for parallel adsorption on the metal surface, and hence, results in higher surface coverage, and in-turn, higher inhibition efficiency. On the contrary, PMPM and PMTM are not planar, as evident from torsion angle values (Fig. 8). This will result in lower degree of surface coverage by PMPM and PMTM on the metal surface. For all the molecules, electrons in HOMO are mostly distributed over the substituted-phenyl ring, the attached heteroatom to the phenyl ring, and the imine group. This may be responsible for observed closeness in EHOMO values for all the inhibitors. Electron distribution in LUMO, on the contrary, is seen to distribute mostly on the pyridine and the imine groups for PMPM and PMTM, and over the whole molecular surface for planar PMAM. Thus, it may be concluded that PMAM utilizes its whole planar molecular surface during bonding from metal to the LUMO. But as PMPM and PMTM are not planar, either of the substituted-phenyl moiety or the pyridine moiety can be adsorbed in a true parallel orientation on the metal surface by inhibitor to metal electron transfer (Fig. 7). Though both the aromatic moieties are not disposed perfectly perpendicular to each other, some probability of simultaneous interaction between metal surface and PMPM or PMTM through both the parallel as well as torsionally twisted non-planar orientation cannot be ruled out. In that case, each molecule will be involved in simultaneous two-ways bond formation, but obviously the overall bond strength will be lower than that observed in the case PMAM. This is manifested in the calculated interaction energy between the Fe (1 1 0) surface and the three Schiff bases, as observed in MD simulation studies. It may be argued that inhibitors preferentially occupy the anodic reaction sites on the metal surface through electron donation from HOMO of the inhibitors, whereas the electrons present in cathodic reactions sites interact mostly with LUMO of the inhibitors. Thus, excess negative charge builds up at the anodic sites, and anodic metal dissolution reaction (i.e., oxidation) rate diminishes. On the other hand, deficiency in the number of available electron for reduction of hydrogen ions leads to decrease in the rate of hydrogen evolution reaction (i.e., reduction reaction) at the cathodic reaction sites. Overall, rate of the corrosion process is mitigated. As in all cases ΔN is positive, interaction involving HOMO of the inhibitors is predominant over that involving LUMO. But as ΔN is seen to decrease following the order PMAM > PMTM > PMPM, the effect of retro-donation (electron transfer from metal to LUMO of the inhibitor) is becoming more and more important in the above order for the inhibitors. This may have significance with the experimentally observed fact that though all the inhibitors are mixed type, PMTM and PMPM are relatively more cathodic than PMAM. It may be noted further that the S atom present in PMTM possesses the maximum tendency towards bond formation through electron donation, as evident from its atomic fk− value. Yet, maximum inhibition efficiency is experimentally observed for PMAM, which is due to favourable energetic disposition of the frontier molecular orbitals, which, in-turn, is influenced by its spatial molecular orientation. Further, from the study of local reactivity of the atoms, it may be concluded by saying that the effect of S atom present on the substituent group attached to the phenyl ring in PMTM is less dominating than the overall effect of the π-electron cloud of the phenyl ring in PMAM in regard to adsorption of these molecules on the metal surface.
Table 7 Torsion angle (°) in the optimized form of the inhibitors
Torsion angle |
PMAM |
PMTM |
PMPM |
C7–N8–C13–C14 |
−176.78 |
−136.79 |
−142.48 |
C7–N8–C13–C10 |
3.55 |
47.39 |
41.96 |
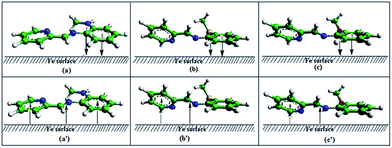 |
| Fig. 8 Schematic representation of probable mode of adsorption of inhibitors, PMAM (a and a′), PMTM (b and b′) and PMPM (c and c′) on metal surface (arrow represents the direction of most probable bonding). Top: adsorption involving HOMO, bottom: adsorption involving LUMO. | |
Conclusion
Present study explicitly manifests that the effect exerted by heteroatom present is not the ultimate criteria in dictating the extent of adsorption, and hence inhibition of corrosion for structurally comparable inhibitor molecules, rather their stereochemical disposition also plays their role in the overall process. Among structurally resembled three Schiff bases studied, the one having N atom is seen to provide greater absorptivity and corrosion inhibition propensity over one having S atom due to conducive spatial orientation which results into favourable energetic criteria of lower energy difference between the frontier molecular orbitals. All the three inhibitors are seen to act broadly as mixed type inhibitor where chemisorption is somewhat predominating over physical adsorption as evidenced from adsorption isotherm and MD simulation studies. The occurrence of adsorption of the studied Schiff bases on metal surface is established unambiguously following FTIR spectra of free and surface adsorbed inhibitor molecules. From electronic distribution of HOMO and LUMO and from atomic reactivity study for all the inhibitors, it is concluded that the phenyl ring and the heteroatom attached to the phenyl ring is mostly involved in bond formation between the inhibitor and metal surface through electron transfer from HOMO to metal. For PMAM showing maximum inhibition effect, simultaneous electron transfer from metal to phenyl ring, the imine as well as pyridine group is probable, which may be due to nearly flat orientation of the molecule. On the contrary, for the other two inhibitors, such simultaneous retro-donation from metal to phenyl ring is not so pronounced. This disparity along with other intrinsic molecular parameters has resulted in the observed sequence of inhibition efficiency, i.e., PMAM > PMTM > PMPM.
Acknowledgements
DS and PB thank Department of Science and Technology (DST), Govt. of India for supporting research projects (ref. no. SR/FT/CS-110/2010, SB/FT/CS-003/2012 and GAP-183112). SKS thanks DST, New Delhi, India for his DST Inspire Fellowship. Authors are thankful to one of the reviewers for valuable suggestions on MD simulation study.
Notes and references
- X. Lei, H. Wang, Y. Feng, J. Zhang, X. Sun, S. Lai, Z. Wang and S. Kang, RSC Adv., 2015, 5, 99084 RSC.
- E. Bayol, T. Gürten, A. A. Gurten and M. Erbil, Mater. Chem. Phys., 2008, 112, 624 CrossRef CAS.
- S. K. Saha, A. Dutta, P. Ghosh, D. Sukul and P. Benerjee, Phys. Chem. Chem. Phys., 2015, 17, 5679 RSC.
- M. Finšgar and J. Jackson, Corros. Sci., 2014, 86, 17 CrossRef.
- M. Jeeva, G. V. Prabhu, M. S. Boobalan and C. M. Rajesh, J. Phys. Chem. C, 2015, 119, 22025 CAS.
- I. B. Obot and N. O. Obi-Egbedi, Corros. Sci., 2010, 52, 198 CrossRef CAS.
- M. Behpour, S. M. Ghoreishi, N. Mohammadi, N. Soltani and M. Salavati-Niasari, Corros. Sci., 2010, 52, 4046 CrossRef CAS.
- D. Daoud, T. Douadi, H. Hamani, S. Chafaa and M. Al-Noaimi, Corros. Sci., 2015, 94, 21 CrossRef CAS.
- L. Herrag, B. Hammouti, S. Elkadiri, A. Aouniti, C. Jama, H. Vezin and F. Bentiss, Corros. Sci., 2010, 52, 3042 CrossRef CAS.
- L. C. Murulana, M. M. Kabanda and E. E. Ebenso, RSC Adv., 2015, 5, 28743 RSC.
- R. Solmaz, Corros. Sci., 2010, 52, 3321 CrossRef CAS.
- M. Hany and A. El-Lateef, Corros. Sci., 2015, 92, 104 CrossRef.
- Y. Abboud, A. Abourriche, T. Saffaj, M. Berrada, M. Charrouf, A. Bennamara, A. Cherqaoui and D. Takky, Appl. Surf. Sci., 2006, 252, 8178 CrossRef CAS.
- B. P. Markhali, R. Naderi, M. Sayebani and M. Mahdavian, Anti-Corros. Methods Mater., 2014, 61, 300 CrossRef CAS.
- P. Piotr, H. Adam, P. Krystian, B. Bogumil and B. Franz, Curr. Org. Chem., 2009, 13, 124 CrossRef.
- D. Sinha, A. K. Tiwari, S. Singh, G. Shukla, P. Mishra, H. Chandra and A. K. Mishra, Eur. J. Med. Chem., 2008, 43, 160 CrossRef CAS PubMed.
- J. Aljourani, K. Raeissi and M. A. Golozar, Corros. Sci., 2009, 51, 1836 CrossRef CAS.
- A. Aytac, U. Ozmen and M. Kabasakaloğlu, Mater. Chem. Phys., 2005, 89, 176 CrossRef CAS.
- Y. Zhou, S. Xu, L. Guo, S. Zhang, H. Lu, Y. Gong and F. Gao, RSC Adv., 2015, 5, 14804 RSC.
- S. Shahabi, P. Norouzi and Md. R. Ganjali, Int. J. Electrochem. Sci., 2015, 10, 2646 CAS.
- M. Palomar-Pardavé, M. Romero-Romo, H. Herrera-Hernández, M. A. Abreu-Quijano, N. V. Likhanova, J. Uruchurtu and J. M. Juárez-García, Corros. Sci., 2012, 54, 231 CrossRef.
- S. Roy, P. Mitra and A. K. Patra, Inorg. Chim. Acta, 2011, 370, 247 CrossRef CAS.
- J. Coates, in Encyclopedia of Analytical Chemistry, ed. R. A. Meyers, John Wiley & Sons Ltd, Chichester, 2000, pp. 10815–10837 Search PubMed.
- F. Neese, An Ab initio, DFT and Semiempirical SCF-MO Package, Version 2.9, Max Planck Institute for Bioinorganic Chemistry, Mulheim an der Ruhr, Germany, 2012 Search PubMed.
- D. A. Becke, J. Chem. Phys., 1986, 84, 4524 CrossRef.
- C. Lee, W. Yang and G. R. Parr, Phys. Rev. B: Condens. Matter Mater. Phys., 1988, 37, 785 CrossRef CAS.
- A. Schafer, C. Huber and R. Ahlrichs, J. Chem. Phys., 1994, 100, 5829 CrossRef.
- L. Pauling, The Nature of the Chemical Bond, Coruell University Press, Ithaca, New York, 1960 Search PubMed.
- R. G. Parr and R. G. Pearson, J. Am. Chem. Soc., 1983, 105, 7512 CrossRef CAS.
- P. Geerlings, F. De Proft and W. Langenaeker, Chem. Rev., 2003, 103, 1793 CrossRef CAS PubMed.
- S. Martinez, Mater. Chem. Phys., 2002, 77, 97 CrossRef.
- M. J. S. Dewar and W. Thiel, J. Am. Chem. Soc., 1963, 85, 3533 CrossRef.
- I. Lukovits, E. Kálmán and F. Zucchi, Corrosion, 2001, 57, 3 CrossRef CAS.
- F. De Proft, J. M. L. Martin and P. Geerlings, Chem. Phys. Lett., 1996, 256, 400 CrossRef CAS.
- R. R. Contreras, P. Fuentealba, M. Galvan and P. Perez, Chem. Phys. Lett., 1999, 304, 405 CrossRef CAS.
- F. L. Hirshfeld, Theor. Chem. Acc., 1977, 44, 129 CrossRef CAS.
- S. K. Saha, A. Dutta, P. Ghosh, D. Sukul and P. Benerjee, Phys. Chem. Chem. Phys., 2016, 18, 17898 RSC.
- K. F. Khaled, Electrochim. Acta, 2010, 55, 6523 CrossRef CAS.
- J. O. 'M. Bockris and S. Srinivasan, Electrochim. Acta, 1964, 9, 31 CrossRef CAS.
- N. Labjar, M. Lebrini, F. Bentiss, N. E. Chihib, S. El Hajjaji and C. Jama, Mater. Chem. Phys., 2010, 119, 330 CrossRef CAS.
- T. Pajkossy, Solid State Ionics, 2005, 176, 1997 CrossRef CAS.
- U. Rammelt and G. Reinhard, Corros. Sci., 1987, 27, 373 CrossRef CAS.
- X. Wu, H. Ma, S. Chen, Z. Xu and A. Sui, J. Electrochem. Soc., 1999, 146, 1847 CrossRef CAS.
- M. Lebrini, F. Robert and C. Roos, Int. J. Electrochem. Sci., 2010, 5, 1698 CAS.
- Z. Zhang, N. C. Tian, X. D. Huang, W. Shang and L. Wu, RSC Adv., 2016, 6, 22250 RSC.
- P. Roy, A. Pal and D. Sukul, RSC Adv., 2014, 4, 10607 RSC.
- P. Roy, P. Karfa, U. Adhikari and D. Sukul, Corros. Sci., 2014, 88, 246 CrossRef CAS.
- A. Pal, S. Dey and D. Sukul, Res. Chem. Intermed., 2016, 42, 4531 CrossRef CAS.
- F. Bentiss, M. Lebrini and M. Lagrenee, Corros. Sci., 2005, 47, 2915 CrossRef CAS.
- M. Finšgar, B. Petovar, K. Xhanari and U. Maver, Corros. Sci., 2016 DOI:10.1016/j.corsci.2016.05.028.
- N. Soltani, H. Salavati, N. Rasouli, M. Paziresh and A. Moghadasi, Chem. Eng. Commun., 2016, 203, 840 CAS.
- I. B. Obot and A. Madhankumar, Mater. Chem. Phys., 2016, 177, 266 CrossRef CAS.
- K. Rengaraj, B. Sivasankar, M. Anbu and M. Palanichamy, J. Chem. Sci., 1991, 103, 707 CAS.
- I. B. Obot and Z. M. Gasem, Corros. Sci., 2014, 83, 359 CrossRef CAS.
- A. Dutta, S. Saha, P. Banerjee and D. Sukul, Corros. Sci., 2015, 98, 541 CrossRef CAS.
- K. Zhang, B. Xu, W. Yang, X. Yin, Y. Liu and Y. Chen, Corros. Sci., 2015, 90, 284 CrossRef CAS.
- A. Kokalj, Electrochim. Acta, 2010, 56, 745 CrossRef CAS.
- N. Kovacevic and A. Kokalj, Corros. Sci., 2011, 53, 909 CrossRef CAS.
- M. K. Awad, M. R. Mustafa and M. M. A. Elnga, J. Mol. Struct.: THEOCHEM, 2010, 959, 66 CrossRef CAS.
- S. John, M. Kuruvilla and A. Joseph, RSC Adv., 2013, 3, 8929 RSC.
- N. O. Obi-Egbedi and I. B. Obot, Corros. Sci., 2011, 53, 263 CrossRef CAS.
- S. Saha and P. Banerjee, RSC Adv., 2015, 5, 71120 RSC.
- J. P. Zeng, J. Y. Zhang and X. D. Gong, Comput. Theor. Chem., 2011, 963, 110 CrossRef CAS.
Footnote |
† Electronic supplementary information (ESI) available: Mass spectra, rest potential variation, polarization diagrams, Nyquist plots, equivalent circuit, adsorption isotherms, Fukui functions. See DOI: 10.1039/c6ra03521c |
|
This journal is © The Royal Society of Chemistry 2016 |
Click here to see how this site uses Cookies. View our privacy policy here.