DOI:
10.1039/C6RA09505D
(Paper)
RSC Adv., 2016,
6, 69431-69441
Effect of surface species and structure on the performance of CeO2 and SO42− doped MCM-41 catalyst toward NH3-SCR†
Received
13th April 2016
, Accepted 11th July 2016
First published on 11th July 2016
Abstract
The present work elucidated the effects of structures and surface species on the activity of CeO2 and SO42− doped MCM-41 catalysts toward NO reduction by NH3. The results indicated that the sulfated species were generated over Cat-A (Ce(NO3)3·6H2O and H2SO4 simultaneously doped on MCM-41) and Cat-B (Ce(NO3)3·6H2O first immersed to MCM-41 and followed by impregnation of H2SO4) except for Cat-C (H2SO4 first doped onto MCM-41 and followed by Ce(NO3)3·6H2O). The sulfate ion contributed to the formation of Ce3+ concentration, oxygen vacancies, and surface acidity, resulting in improvement of SCR activity. However, the positive influence on SCR activity was overwhelmed by the decomposition of sulfated species. The apparent activation energy of Cat-A (60 kJ mol−1) was much lower compared with that of Cat-B and Cat-C (71 and 78 kJ mol−1, respectively). Pore structures and surface species were responsible for the SCR activity over the CeO2 and SO42− doped MCM-41 catalysts. The best catalytic activity was obtained over Cat-A, followed by Cat-B, and Cat-C exhibited the least catalytic activity.
1. Introduction
Nitrogen oxide species (NOx) emission from flue gases of stationary sources are important pollutants in the air, which not only cause various environmental problems such as greenhouse effects, acid rain, and photochemical smog, but also affect human health.1 With stringent NOx emission legislation, many strategies have been implemented for controlling NOx emission. Among NOx removal technologies, selective catalytic reduction (SCR) of NOx by NH3 is one of the most widely used technologies for controlling NOx from stationary resources, and the commercial catalyst is V2O5–WO3 (MoO3)/TiO2.2,3 However, the potential volatility and intense toxicity of vanadium oxide are harmful to human health and the environment.4,5 Consequently, great efforts have been made to develop environmentally-friendly SCR catalysts.
In recent years, mesoporous materials have been extensively used for catalyst support due to their large specific surface area, favored pore diameter, and ordered framework.6 MCM-41, a typical representative in mesoporous materials, possesses potential catalytic applications. It is well known that pure MCM-41 mesoporous molecular sieves are restrained in industry application due to some disadvantages, such as inefficient catalytic activity and weak surface acidity. However, pure MCM-41 can be modified by some transition metals, remarkably improving the catalytic activity and surface acidity.7–11 Liu et al.11 reported that the Cu/MCM-41 catalysts exhibited excellent catalytic activity in the NO-SCR reaction. Wan et al.12 also revealed that high catalytic activity of Cu–Al-MCM-41 modified by Cu and Al species was obtained in the C3H6-SCR reaction. Besides, Qiu et al.13 investigated the effect of Cu contents on NH3-SCR activity over MCM-41 catalysts. Ziolek et al.14 reported that surface acidity could be improved by the introduction of Nb into MCM-41 in the NH3-SCR reaction. Meanwhile, few studies have been done to elucidate the effect of structures and surface species on SCR activity over MCM-41 modified by some transition metals in the NH3-SCR reaction.
Recently, CeO2-based catalysts are extensively investigated because of their excellent oxygen storage capacity and redox ability in the NH3-SCR reaction. Furthermore, supported SCR catalysts are dramatically influenced by the kinds of surface species and the structures of supporting materials. Excellent dispersion of active CeO2 particles, which contributes to the redox ability and surface acidity, can be facilitated by the favored structure of MCM-41, resulting in improvement of catalytic activity in the NH3-SCR reaction. Therefore, the structures and surface species of MCM-41 modified by CeO2 should be intensively studied.
Sulfate modification is an effective method to increase catalytic activity for the improvement of surface acidity over CeO2-based catalysts.15–18 But the effect of sulfated CeO2 on the structures and SCR performances over MCM-41 has been scarcely investigated. It is widely accepted that surface species and structures of the support catalysts are remarkably affected by the metal-loading procedure, resulting in different catalytic activities.19,20 Thereby, the effect of CeO2 and SO42− doped MCM-41 on SCR activity can be investigated in the NH3-SCR reaction.
In this work, the CeO2 and SO42− doped MCM-41 supports were prepared by an impregnation method. The specific surface area, pore structures, surface species, and catalytic activity were investigated. Furthermore, characteristics of the samples were explored by XRD, NH3-TPD, TGA, N2 adsorption-desorption, H2-TPR, Raman, and XPS.
2. Experimental
2.1 Catalyst preparation
MCM-41 supports were purchased from Nanjing Xian-feng nanomaterials technology Co., Ltd. Ce(NO3)3·6H2O and H2SO4 were purchased from Sinopharm Chemical Reagent Co., Ltd, and both were analytical grade. The CeO2 and SO42− doped MCM-41 were prepared by a wet impregnation method. First, the Ce(NO3)3·6H2O (1.5 g) and 0.1 g mL−1 H2SO4 (3 mL) were simultaneously dissolved in deionized water (3 mL) and then 2.1 g of the MCM-41 supports were immersed. The mixture was kept at 60 °C for 5 h and dried for 12 h at 105 °C. The powders were calcined at 550 °C for 5 h in air and the catalysts were named as Cat-A. Second, 0.1 g mL−1 H2SO4 (3 mL) was dissolved in deionized water (3 mL) and then 2.1 g of the MCM-41 supports were added. This powder was maintained at 60 °C for 5 h, dried for 12 h at 105 °C, and calcined at 550 °C for 5 h in air. The obtained powders were placed into the 1.5 g Ce(NO3)3·6H2O again. The above powders were immersed at 60 °C for 5 h, dried for 12 h at 105 °C, and calcined at 550 °C for 5 h. The catalysts were assigned as Cat-B. Third, the process was the same as Cat-B, but the impregnation sequence of Ce(NO3)3·6H2O and H2SO4 was opposite. The 1.5 g Ce(NO3)3·6H2O was first immersed, and followed by a 0.1 g mL−1 H2SO4 (3 mL) solution. This catalyst was marked as Cat-C.
2.2 Catalyst characterization
Powder X-ray diffraction (XRD) measurements were made with a Rigaku D/Max 2500 system between 10° and 70° at a step of 6° min−1 by using Cu Kα (40 kV, 100 mA) radiation to determine the crystal structure.
Raman spectra (RS) were performed on a Renishaw-2000 Raman spectrometer at a resolution of 2 cm−1 by using the 514.5 nm line of an Ar ion laser as the excitation source.
TGA experiments were carried out on a Simultaneous Thermal Analyzer (DTG-60H) with 0.009 mg samples under a nitrogen flow of 10 mL min−1. The temperature was increased from 50 to 900 °C at a rate of 10 °C min−1.
The specific surface areas of the catalysts were obtained at −196 °C using a TristarII3020 automated gas sorption system. Prior to N2 measurement, the catalysts were degassed at 300 °C for 4 h. The specific surface areas were measured with a Brunauer–Emmett–Teller (BET) model instrument. Average pore diameters and total pore volumes were obtained from desorption branches of the isotherms by the Barrett–Joyner–Halenda (BJH) method.
X-ray photoelectron spectroscopy (XPS) was performed in an ULVAC PHI 5000 Versa Probe-II equipment operating at 10−9 Pa with an Al Kα radiation (1486.6 eV) to analyze the chemical states and the surface atomic concentration of the catalysts. The observed spectra were corrected with the C 1s binding energy value of 284.8 eV.
The H2 temperature-programmed reduction (H2-TPR) experiment was conducted on a GC-9750 equipped with a thermal conductivity detector. Before the H2-TPR experiment, the catalysts were pretreated in pure N2 at 400 °C for 60 min and cooled to 100 °C. The H2-TPR runs were performed in a flow of 5 vol% H2/Ar (30 mL min−1) from 100 to 900 °C with a heating rate of 10 °C min−1.
The temperature programmed desorption of ammonia (NH3-TPD) of samples was carried out in order to investigate the surface acidity using gas chromatography with a thermal conductivity detector (TCD) setup (Fuli, GC-9750, China). Before the test, the samples were pretreated in N2 at 400 °C for 45 min, followed by introduction of 5% NH3/N2 at a flow rate of 30 mL min−1 for about 60 min. Desorption was performed by heating from 100 to 450 °C with a heating rate of 10 °C min−1.
2.3 Activity test
The SCR activity measurements were obtained using a fixed-bed quartz reactor (8 mm i.d.) with 0.4 mL catalysts. The experiments were performed at 150–450 °C and the gas flow was measured by mass flow meters. The reactant gas composition was as follows: 600 ppm NO, 600 ppm NH3, 5 vol% H2O (when used), 50 ppm SO2 (when used), 5 vol% O2 and N2 balance. The total flow rate was 400 mL min−1, corresponding to a gas hourly space velocity of 60
000 h−1. The concentrations of NOx and NH3 were continuously measured by an ECOM J2KN and GXH-1050E flue gas analyzer, respectively. The N2O species were analyzed with a gas chromatograph (Fuli, 9790). To minimize the effect of gas adsorption on the catalysts, the reaction system was kept for 30 min at each reaction temperature to reach a steady state before analysis.
3. Results and discussions
3.1 XRD analysis
The XRD patterns of Cat-A, Cat-B, and Cat-C catalysts are shown in Fig. 1a. The XRD pattern of Cat-A exhibited the presence of cubic CeO2 phase (PDF: 43-1003), together with orthorhombic Ce2(SO4)3 (PDF: 52-1494) peaks. The Cat-B and Cat-C catalysts merely showed XRD peaks corresponding to cubic CeO2 phase (PDF: 43-1003). No sulfated species were observed with Cat-B and Cat-C catalysts, which indicated sulfated species were in the forms of a highly dispersed state or clustered state, which were too small to be detected by XRD over these two samples. Besides, strengths of the peaks of Cat-B and Cat-C were higher than that of Cat-A, and the value of FWHM (full width at half maximum) over Cat-A in Table 1 was larger than those of Cat-B and Cat-C, suggesting that Cat-B and Cat-C possessed higher crystallinity than Cat-A. According to Zhang et al.'s reports,21 the lattice parameter could serve as a function of the particle sizes of catalysts, and the lattice parameter would increase with a decrease in particle sizes of catalysts. Therefore, the lattice parameter a was calculated in the 2θ range of 27–30° in Fig. 1b for the catalysts, and the results are presented in Table 1. It was obvious that the lattice parameter a of Cat-A was larger than that of Cat-B and Cat-C. The phenomenon reflected that the Cat-A catalyst possessed smaller particle sizes in comparison with Cat-B and Cat-C.
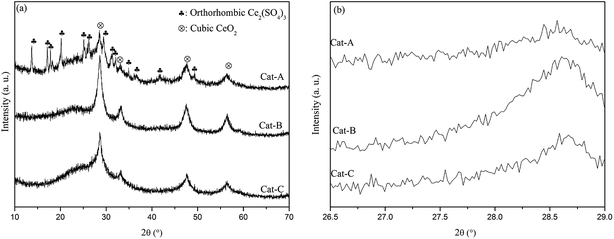 |
| Fig. 1 XRD patterns of different catalysts. | |
Table 1 Lattice parameter and FWHM of Cat-A, Cat-B and Cat-C catalysts
Sample |
FWHM |
2θ/° |
Lattice parameter a/Å |
Cat-A |
1.35 |
28.55 |
5.408 |
Cat-B |
1.20 |
28.67 |
5.386 |
Cat-C |
1.28 |
28.64 |
5.393 |
3.2 N2 adsorption–desorption isotherms
Fig. 2 shows the nitrogen adsorption–desorption isotherms and the BJH pore size distribution curves of the catalysts. It was clear from Fig. 2 that all the catalysts showed type IV isotherms (according to IUPAC classification), which was typically characteristic of mesopores (2–50 nm).22 For the Cat-B and Cat-C samples, there were two regions of hysteresis: one at P/P0 > 0.35 and the other at 0.2, which suggested that a greater abundance of micropores and mesoporous existed over these two samples. Furthermore, the appearance of a H2 hysteresis loop was observed over pure MCM-41 with ink-bottle-type pores where large cavities were connected by narrow channels.23,24 However, the hysteresis loop was absent over Cat-A, Cat-B, and Cat-C catalysts. In other words, the adsorption isotherm nearly coincided with the desorption isotherm over the three samples. This phenomenon indicated that the pores of Cat-A, Cat-B, and Cat-C were open pores.25,26 After the simultaneous introduction of Ce(NO3)3·6H2O and H2SO4, the profile of the curve over Cat-A changed. The volume of adsorbed N2 also decreased, indicating a remarkable reduction of total pore volume and specific surface area, as shown in Table 2. In brief, the pore structures of Cat-A, Cat-B, and Cat-C changed due to the introduction of Ce(NO3)3·6H2O and H2SO4 inside the framework of MCM-41. Fig. 2 also showed the BJH pore size distributions of the samples. The Cat-A catalyst mainly showed and exhibited distribution peaks near 2.8 and 4.2 nm, and desorption pore size distributions of Cat-B and Cat-C were 3.0 nm. Besides, a dramatic decrease in the N2 desorption differential pore volumes per weight (cm3 g−1 nm−1) of Cat-A was observed when compared with Cat-B and Cat-C. This meant that the abundant mesopores disappeared over the Cat-A catalyst, resulting in a decrease in BET surface area.
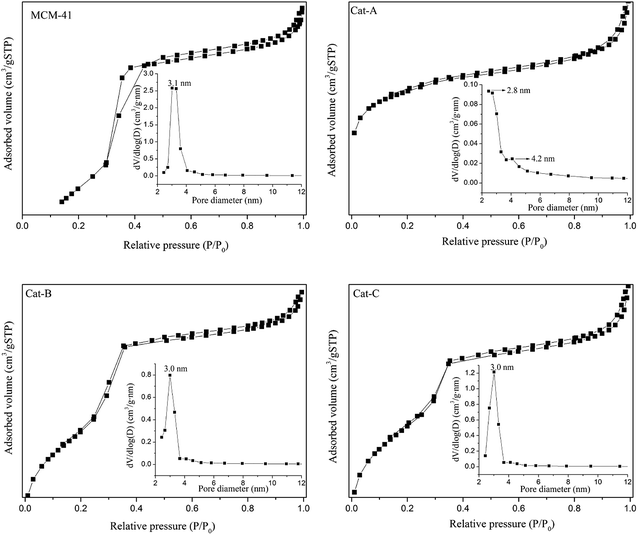 |
| Fig. 2 N2 adsorption–desorption results of the catalysts. | |
Table 2 BET measurements for the catalysts
Samples |
BET (m2 g−1) |
Total pore volume (cm3 g−1) |
Average pore diameter (nm) |
MCM-41 |
952 |
1.0452 |
3.47 |
Cat-A |
336 |
0.1302 |
6.75 |
Cat-B |
555 |
0.4598 |
3.34 |
Cat-C |
519 |
0.3665 |
3.84 |
Table 2 shows structure properties such as the BET surface area, the average pore diameter, and the BJH desorption pore volume of the samples from N2 physisorption results. The BET surface areas of the MCM-41, Cat-A, Cat-B, and Cat-C were 952, 336, 555, and 519 m2 g−1, respectively. After addition of CeO2 and SO42−, the BET surface area of pure MCM-41 decreased remarkably. The total pore volume decreased according to the sequence: Cat-A (0.1302 cm3 g−1) < Cat-C (0.3665 cm3 g−1) < Cat-B (0.4598 cm3 g−1) < MCM-41 (1.0452 cm3 g−1). The average pore diameter was as follows: 6.67 nm for Cat-A > 3.84 nm for Cat-C > 3.34 nm for Cat-B. It was obvious that the BET surface areas and total pore volume of Cat-A, Cat-B, and Cat-C both remarkably decreased compared with those of pure MCM-41, which indicated that the mesopores of the MCM-41 support were blocked by the aggregated Ce2(SO4)3 and the separated CeO2 and SO42− species, leading to the disappearance of small pores and then a dramatically decrease in BET surface area. Based on the above discussion, the catalysts with the same composition but different impregnation sequences showed significantly different pore structures. It was reasonably speculated that the structural properties of Cat-A, Cat-B, and Cat-C catalysts were affected by the surface species over the samples.
3.3 Raman
The Raman spectra are shown in Fig. 3. It was obvious that all of samples were dominated by the main band at 460 cm−1, which was attributed to the F 2g mode of fluorite-type CeO2.27 The bands at 1005 cm−1 correspond to the reflection of sulfate groups over Cat-A and Cat-B catalysts.16 However, the sulfate species were not observed over Cat-C. In comparison with the Cat-B and Cat-C catalysts, the Cat-A catalyst not only showed a decrease in the intensity of the band at 460 cm−1, but also an increase in the FWHM value. Ilieva et al.28 reported that an increase in the FWHM value was ascribed to defect formation or the small crystal size in the CeO2 structure. In addition, these observables in width of the F 2g mode implied the effect of oxygen vacancies and inhomogeneous strain (related to the existence of low-valence cerium states). Wang et al.29,30 also proved that the shift and width in the main F 2g mode band was closely related to the presence of reduced states of cerium. These indicated that the surface species and structures depended on the impregnation sequence of CeO2 and SO42− species over MCM-41.
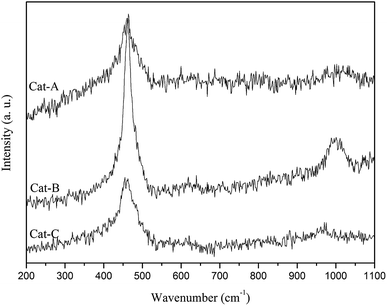 |
| Fig. 3 Raman profiles of the catalysts. | |
3.4 TGA analysis
In order to investigate the effect of structures and surface species on the SCR activity over the Cat-A, Cat-B, and Cat-C catalysts, thermo-gravimetric analysis (TGA) was applied and the results are shown in Fig. 4. It was obvious that the whole temperature range could be divided into two stages:
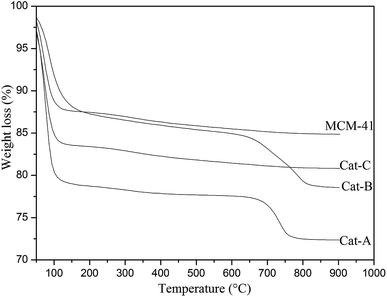 |
| Fig. 4 Thermo-gravimetric analysis of the catalysts. | |
In the first stage at 30–250 °C, a remarkable weight loss was observed over the three samples, corresponding to desorption of physical adsorbed water and dehydroxylation,16 and the weight loss was shown as follows: MCM-41 (12.5%) < Cat-B (13%) < Cat-C (17%) < Cat-A (21.6%).
The second stage was at about 600–900 °C and for the Cat-A and Cat-B catalysts a weight loss was observed, respectively. This weight loss was attributed to the decomposition of Ce2(SO4)3.31 However, no weight loss was found over the Cat-C catalyst, implying that there was no formation of Ce2(SO4)3.
3.5 XPS analysis
Atomic concentrations of the catalysts are presented in Table 3. According to the XPS results, it was obvious that surface atomic concentration of Ce over Cat-C was the highest, followed by Cat-A and Cat-B which showed the lowest surface atomic concentrations. Interestingly, the surface S atomic concentration (provided in ESI†) was not detected over the three catalysts, which indicated that the sulfated species were likely to insert into the MCM-41 pores.
Table 3 Surface atomic concentration of the catalysts from XPS results (atomic%)
Samples |
Surface atomic concentration (atomic%) |
Ce |
S |
Si |
O |
Ce3+/(Ce4+ + Ce3+) |
Oα/(Oα + Oβ) |
Cat-A |
2.8 |
0 |
20.85 |
76.35 |
24 |
67.7 |
Cat-B |
1.13 |
0 |
25.07 |
73.80 |
17.8 |
73.7 |
Cat-C |
4.87 |
0 |
19.67 |
75.46 |
16.3 |
59.4 |
The Ce 3d XPS spectra of the Cat-A, Cat-B, and Cat-C samples are shown in Fig. 5a. The u, u′′, u′′′, v, v′′, and v′′′ peaks were attributed to the Ce4+ state while the u′ and v′ peaks corresponded to the Ce3+ state.32,33 It was well known that the CeO2-based catalysts showed outstanding reducibility via the redox shift between Ce4+ and Ce3+ which improved the oxygen storage/release capacity. Thereby, the surface Ce3+ and Ce4+ concentrations were calculated and the results are shown in Table 3. The Ce3+/(Ce3+ + Ce4+) ratio ranked in the sequence of Cat-A (24%) > Cat-B (17.8%) > Cat-C (16.3%). Besides, the Ce3+ enrichment over Cat-A was ascribed to the appearance of lattice defects or oxygen vacancies. Combined with XRD results, the Ce2(SO4)3 species were observed but the S species were not detected over the Cat-A catalyst as seen from Table 3. These further confirmed that the sulfonation could not exist at the outer surface of MCM-41 modified by Ce(NO3)3·6H2O and SO42− species. The sulfonation not only served as a stabilizer of oxygen vacancies during the impregnation and calcination process, but also contributed to reducing the oxidation state of the surface cations.34,35 Thus, the surface Ce3+ concentration of Cat-A was improved when compared with those of Cat-B and Cat-C.
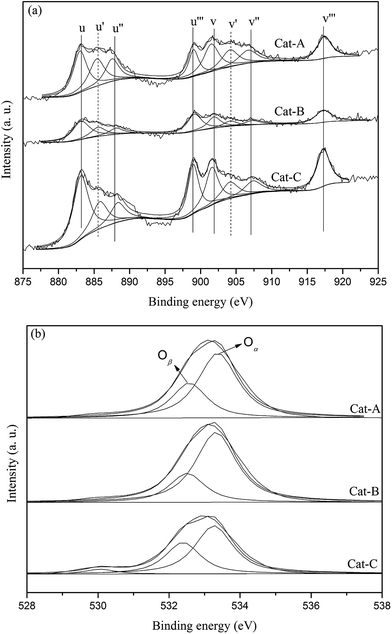 |
| Fig. 5 (a) Ce 3d XPS spectra of the catalysts. (b) O 1s XPS spectra of the catalysts. | |
Fig. 5b shows the O 1s XPS spectra of the Cat-A, Cat-B, and Cat-C catalysts. The O 1s peaks at 531.5–532.3 eV were assigned to the lattice oxygen (marked as Oβ)36,37 and the other peak at 532–533 eV could be attributed to the chemisorbed oxygen (marked as Oα).38 In order to reflect the variation of oxygen species, the percentage of the chemisorbed oxygen and lattice oxygen were measured by Gaussian curve fitting and the data are shown in Table 3. The Oα/(Oα + Oβ) ratio of Cat-A, Cat-B, and Cat-C was 67.7%, 73.7%, and 59.4%, respectively.
3.6 H2-TPR analysis
The reducibility of Cat-A, Cat-C, and Cat-B was characterized by H2-TPR experiments and the results are exhibited in Fig. 6. The reduction peaks of the surface CeO2 and the bulk CeO2 over pure CeO2 are located at 509 and 800 °C, respectively.39 For the Cat-C catalyst, TPR curve consisted of two peaks: a low-temperature peak and a high-temperature peak, which was ascribed to the reduction of surface CeO2 and bulk CeO2, respectively. But the intensity reduction peaks of Cat-C were much weaker than those of Cat-A and Cat-B. The Cat-A catalyst with a shoulder peak at 660 °C exhibited a sharp reduction peak at 694 °C, corresponding to the reduction of surface CeO2 and sulfated species. It was obvious that the Cat-B catalyst showed merely one reduction peak at 665 °C, which was attributed to the reduction of sulfated species. Compared with Cat-B and Cat-C, the reduction temperature of surface CeO2 and sulfated species over Cat-A both shifted to higher temperatures, the reason of which might be that the strongest interaction between Ce, SO42− and support existed. Besides, no reduction of sulfated species was observed over Cat-C. Furthermore, the intensity reduction peak of sulfated species over Cat-A was stronger than that of Cat-B. This phenomena indicated that the redox properties of Cat-A, Cat-B, and Cat-C were dramatically affected by the surface species and structures.
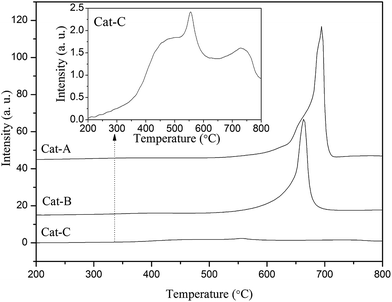 |
| Fig. 6 H2-TPR results of the catalysts. | |
3.7 NH3-TPD analysis
NH3-TPD analysis was performed in order to investigate the effect of surface acidity over Cat-A, Cat-B, and Cat-C. As shown in Fig. 7, Cat-A showed two NH3-desorption peaks: the first one centered at 175 °C, attributed to weak acidity; and the other located at 320 °C, corresponding to medium acidity.40 Weaker peaks were observed at 100–250 °C on the Cat-B and Cat-C when compared with that of Cat-A, implying that Cat-A possessed a larger amount of weakly bound NH3 sites. However, the intensity peaks of NH3 desorption over Cat-A, Cat-B, and Cat-C were remarkably higher than that of pure MCM-41, which meant that the surface acidity over the catalysts was greatly improved by the introduction of CeO2 and SO42− species into MCM-41. In addition, the NH3-TPD results also revealed that the amount and distribution of surface acidity were affected significantly by the different structures and surface species over Cat-A, Cat-B, and Cat-C.
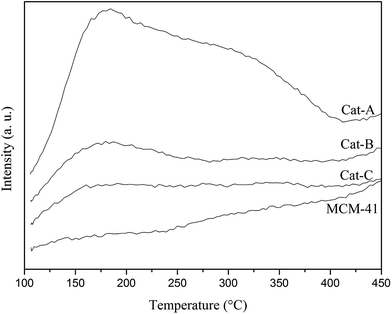 |
| Fig. 7 NH3-TPD results of the catalysts. | |
3.8 The SCR activity of the catalysts
Catalytic activities of the Cat-A, Cat-B, and Cat-C catalysts are shown in Fig. 8. Pure MCM-41 exhibited inferior catalytic activity and its maximum NOx conversion was about 35% at 400 °C. With the addition of Ce(NO3)3·6H2O and SO42− species into MCM-41, the SCR performance was dramatically enhanced. The Cat-C catalyst exhibited slight NH3-SCR activity and 18.7% NOx conversion was attained at 250 °C. With an increase of temperature, the Cat-C catalyst showed above 80% NOx conversion at 337–450 °C. For the Cat-B catalyst, the SCR activity was dramatically promoted, its light-off temperature T50 (NOx conversion was 50%) was about 268 °C and over 80% NOx conversion was in the temperature range of 294–450 °C. The T50 of Cat-A decreased to 230 °C and more than 80% NOx conversion was observed from 252–450 °C. It was obvious that the catalytic activity of Cat-A was evidently higher than Cat-B and Cat-C with the same components but different surface species and structures, which resulted in different SCR performance. Consequently, the catalytic activities of the catalysts were affected by the surface species and structures of Cat-A, Cat-B, and Cat-C, and Cat-A showed the best catalytic activity.
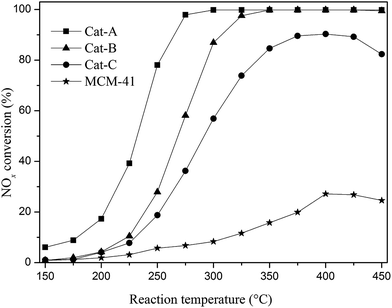 |
| Fig. 8 NOx conversion for the catalysts. | |
The results of N2O production and N2 selectivity of Cat-A, Cat-B, and Cat-C catalysts are exhibited in Fig. 9. The low N2O production (below 12 ppm) was generated over the samples. Above 97% N2 selectivity of Cat-A, Cat-B, and Cat-C was attained at 150–450 °C. The Cat-A, Cat-B, and Cat-C catalysts showed excellent N2 selectivity in the temperature range of 150–450 °C. Hence, the CeO2 and SO42− species doped into MCM-41 is a promising environmentally-friendly SCR catalyst.
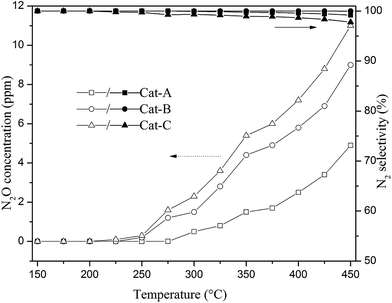 |
| Fig. 9 N2O production and N2 selectivity of the catalysts. | |
3.9 NO oxidation
In order to investigate NO oxidation ability of the Cat-A, Cat-B, and Cat-C catalysts, the properties of NO oxidation to NO2 are exhibited in Fig. 10. It was obvious that NO2 productions of Cat-A and Cat-C were remarkably higher than that of Cat-B at 150–450 °C. These indicated that Cat-A and Cat-C showed excellent oxidation ability. Roy et al.41 reported that the evident difference of NH3-SCR catalysts in NO oxidation ability and SCR performance could be assigned to the role of NH3 during the SCR process. Therefore, surface acidity played an important role in NO oxidation activity. Combined with SCR activity and NH3-TPD results, Cat-C with the weakest surface acidity showed the least SCR performance. This indicated that the NO oxidation ability over Cat-A, Cat-B, and Cat-C was not the only factor for determining the catalytic activity.
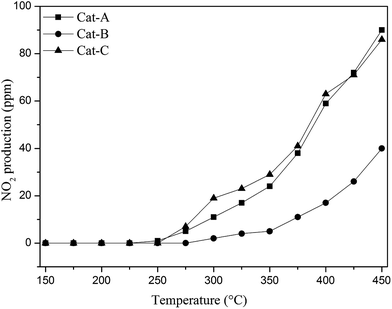 |
| Fig. 10 NO oxidation activity over the Cat-A, Cat-B and Cat-C catalysts. | |
3.10 NH3 oxidation
The NH3 oxidation activity and by-products (N2O, NO, and NO2) of the Cat-A, Cat-B, and Cat-C catalysts are shown in Fig. 11. The NH3 conversion of Cat-A was higher than those of Cat-B and Cat-C, especially for low temperature (below 300 °C) and the NH3 conversion decreased as follows: Cat-A > Cat-B ≈ Cat-C. The concentration of NO and N2O over Cat-C was dramatically higher than that of Cat-A and Cat-B. The NO2 production was not detected at 150–450 °C and N2O production was detected at higher temperatures (above 350 °C) over Cat-A, Cat-B, and Cat-C. It was well known that the excellent redox property could result in unwanted NH3 unselective oxidation, causing the negative NOx conversion at high temperatures. From the H2-TPR results, Cat-C showed the lowest reduction temperature, which contributed to NH3 unselective oxidation. Thereby, Cat-C possessed inferior catalytic activity at high temperatures. It was reported that surface acidity played an important role in activating NH3, which was the necessary step in the NH3-SCR reaction, resulting in enhancement of the catalytic activity and N2 selectivity.42 The formation of sulfated species over Cat-A and Cat-B could contribute to surface acidity, remaining a proper redox ability, and then weaken the NH3 unselective catalytic oxidation, leading to an enhanced N2 selectivity and SCR activity. Consequently, the surface acidity could contribute to the NH3 conversion and catalytic activity. Combined with the NH3-TPD and SCR performance results, Cat-A possessed excellent NH3 conversion and SCR activity in comparison with Cat-B and Cat-C, which might be ascribed to the abundance of weak and medium surface acidity.
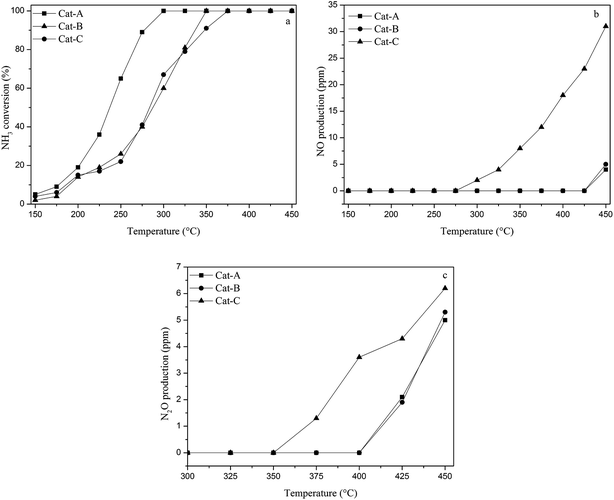 |
| Fig. 11 NH3 oxidation activity (a) and by-products over the catalysts: NO concentration (b); and N2O concentration (c). | |
In order to elucidate the effect of surface species and structures on SCR performance over the catalysts, pure MCM-41, Cat-A, Cat-B, and Cat-C were calcined at 650 °C for 4 h and named as A-MCM-41, A-Cat-A, A-Cat-B, and A-Cat-C, respectively. The BET surface areas, pore volumes, and average pore diameters of the A-Cat-A, A-Cat-B, and A-Cat-C catalysts results are shown in Table 4. The BET surface areas ranked in the sequence of A-Cat-C (478) > A-Cat-A (412) > A-Cat-B (100). From Fig. 12, A-Cat-A exhibited the best catalytic activity, followed by A-Cat-B, and A-Cat-C showed an inferior performance. When the calcination temperature was 650 °C, a portion of sulfated species would be decomposed from the TGA results; the structure would be destroyed under high temperature treatment, and this led to the decrease of catalytic activity in either event (the decomposition of sulfated species or the collapse of structure). Furthermore, pure A-MCM-41 possessed a lager BET surface area (921 m2 g−1) compared with that of the three aged samples, but it showed quite poor SCR activity. No obvious linear dependence between the catalytic activity and the BET surface areas was established. In addition, the catalytic activities of A-Cat-A, A-Cat-B, and A-Cat-C were decreased when compared with those of the fresh samples. Thereby, it was reasonable that decomposition of sulfated species could result in deactivation over the samples. Consequently, the formation of sulfated species over MCM-41 modified by Ce(NO3)3·6H2O and SO42− contributed to the improvement of SCR activity.
Table 4 BET measurements for the A-Cat-A, A-Cat-B and A-Cat-C catalysts
Samples |
BET (m2 g−1) |
Total pore volume (cm3 g−1) |
Average pore diameter (nm) |
A-MCM-41 |
921 |
0.9785 |
3.38 |
A-Cat-A |
412 |
0.1468 |
5.83 |
A-Cat-B |
100 |
0.1164 |
7.55 |
A-Cat-C |
478 |
0.3703 |
3.57 |
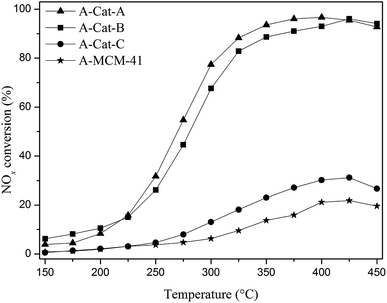 |
| Fig. 12 NOx conversion for the aged catalysts. | |
To investigate the effect of surface species and structures on catalytic activity over the Cat-A, Cat-B, and Cat-C catalysts, the SCR performance was studied by a macro-kinetic approach and the results are shown in Fig. 13. Generally speaking, with respect to NO, the NH3-SCR reaction was a first-order reaction.43,44 Therefore, the effective first-order rate constant was related to NO conversion by:
where
k: reaction constant (cm
3 g
−1 s
−1);
V: the total gas flow rate (mL s
−1);
W: the catalyst weight (g);
x: the NO conversion (%);
A: the pre-exponential factor;
R: the gas constant (8.3145 J mol
−1 K
−1);
T: the temperature (K); and
Ea: the apparent activation energy (kJ mol
−1). The measured activation energies of NO conversion over Cat-A, Cat-B, and Cat-C were 60, 78, and 71 kJ mol
−1, respectively (
Fig. 13). These differences originated from different samples with the same components but different surface species and structure. The different surface species and structures over Cat-A, Cat-B, and Cat-C should be further discussed because the performance of the samples was significantly affected by the different structures, redox properties, and surface species. Combined with the XRD and BET results, the particle sizes of CeO
2 over Cat-A was smaller than the other two samples. It was reported
45 that excellent dispersion of metal species on the support contributed to the catalytic activity of supported metal catalysts, which might be another reason that the Cat-A catalyst showed superior SCR performance. However, the Cat-A catalyst possessed the least BET surface area for the fresh samples. The reason was that the small pores were blocked or filled by the formation of sulfate species, resulting in the disappearance of small pores and then a decrease in the specific surface area. Meanwhile, the Cat-A catalyst exhibited the best catalytic activity due to the formation of sulfated species. From TGA results, the sulfated species existed on the Cat-A and Cat-B catalysts, and the weight loss of Cat-A was more evident than that of Cat-B and Cat-C at 30–150 °C, indicating occurrence of the largest number of hydroxyls over Cat-A, which contributed to the surface acidity, and then resulted in the excellent catalytic activity. Taking the SCR performance results into account, the catalytic activity ranked as follows: Cat-A > Cat-B > Cat-C. This phenomenon further confirmed that the SCR activity was dramatically improved by the formation of sulfated species. Considering the Raman, XPS, and H
2-TPR results, the occurrence of the interaction between Ce, SO
42− and MCM-41 led to a difficulty in reducing the surface CeO
2 and sulfated species over the Cat-A catalyst. However, the sulfonation could contribute to the formation of Ce
3+ concentration and surface acidity. The higher Ce
3+/(Ce
3+ + Ce
4+) ratio led to more oxygen vacancies, unsaturated chemical bonds, and a charge imbalance, which was beneficial to the activation and transportation of active oxygen species, resulting in the improvement of SCR activity. The surface acidity could contribute to adsorption of ammonia and then improve the catalytic activity. Hence, the sequence of catalytic activities over Cat-A, Cat-B, and Cat-C was the same as that of Ce
3+/(Ce
3+ + Ce
4+) ratio and amount of surface acidity. These also confirmed that the formation of sulfated species on the surface of MCM-41 could contribute to the SCR activity. Meanwhile, the O
α/(O
α + O
β) of Cat-A (67.7%) was lower than that of Cat-B (73.7%), which was due to the formation of sulfated species and then covered on the surface of Cat-A, leading to a decrease in surface oxygen species. Therefore, the catalytic activity of Cat-A, Cat-B, and Cat-C was dependent on the different surface species and structures.
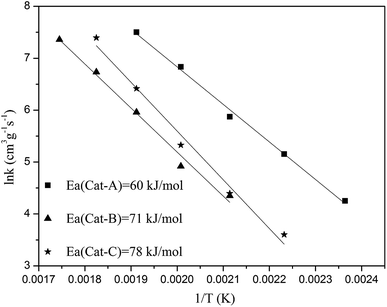 |
| Fig. 13 Arrhenius plots for NOx conversion over different catalysts. | |
3.11 The effect of H2O and SO2 on SCR activity
The emission of SO2 and H2O is unavoidable in exhaust gases from power plants. Therefore, the effect of SO2 and H2O on SCR performance should not be ignored. Fig. 14 presents the effect of SO2 and H2O on catalytic activity at 350 °C over the Cat-A, Cat-B, and Cat-C catalysts. As shown in Fig. 14, nearly 100% NOx conversion of Cat-A was obtained in the presence of 5 vol% H2O, and no decrease in SCR activity was observed for 9.5 h. When 5 vol% H2O and 50 ppm SO2 were added, the NOx conversion of Cat-A was decreased. However, almost 80% NOx conversion still was attained during the measured period although the NOx conversion could not recover to its original value after 5 vol% H2O and 50 ppm SO2 were cut off. However, the Cat-A catalyst still exhibited a superior SCR performance and 97% NOx conversion was obtained. Thereby, Cat-A possessed the best SO2+H2O durability.
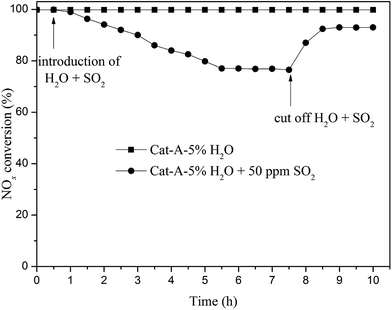 |
| Fig. 14 The effect of H2O and SO2 on SCR activity over Cat-A. | |
4. Conclusions
The present work investigated the effect of surface species and structures on SCR activity of a CeO2 and SO42− doped MCM-41 support. The results revealed that Cat-A showed the best SCR performance and above 80% NOx conversion was obtained in the temperature range of 294–450 °C. The XRD results suggested that Cat-A possessed the smallest CeO2 particle sizes. The BET results suggested that the specific surface area was not the only factor affecting catalytic activity. The Raman and H2-TPR results implied that an interaction between Ce, SO42− and MCM-41 existed and the sulfated species formed over Cat-A and Cat-B, which could affect the redox ability. The TGA results indicated that the sulfated species were generated over Cat-A and Cat-B. The XPS and NH3-TPD results proved that Cat-A possessed higher surface Ce3+/(Ce3+ + Ce4+) ratio and a larger amount of surface acid sites. The apparent activation energy of Cat-A, Cat-B, and Cat-C was 60, 71, and 78 kJ mol−1, respectively. These above factors depend on the structure and surface species over CeO2 and SO42− doped MCM-41, which could in turn affect the catalytic activity.
Acknowledgements
This work is supported by the National Natural Science Foundation of China (No. 21307047) and Academic Newcomer Award of Yunnan Province.
References
- C. H. He, Y. H. Wang, Y. S. Cheng, K. L. Christine and R. T. Yang, Activity, stability and hydrocarbon deactivation of Fe/Beta catalyst for SCR of NO with ammonia, Appl. Catal., A, 2009, 368, 121–126 CrossRef CAS.
- B. Guan, R. Zhan, H. Lin and Z. Huang, Review of state of the art technologies of selective catalytic reduction of NOx from diesel engine exhaust, Appl. Therm. Eng., 2014, 66, 395–414 CrossRef CAS.
- T. V. W. Janssens, H. Falsig, L. F. Lundegaard, P. N. R. Vennestrøm, S. B. Rasmussen, P. G. Moses, F. Giordanino, E. Borfecchia, K. A. Lomachenko, C. Lamberti, S. Bordiga, A. Godiksen, S. Mossin and P. Beato, A Consistent Reaction Scheme for the Selective Catalytic Reduction of Nitrogen Oxides with Ammonia, ACS Catal., 2015, 5, 2832–2845 CrossRef CAS.
- I. Lezcano-Gonzalez, U. Deka, H. E. van der Bij, P. Paalanen, B. Arstad, B. M. Weckhuysen and A. M. Beale, Chemical deactivation of Cu-SSZ-13 ammonia selective catalytic reduction (NH3-SCR) systems, Appl. Catal., B, 2014, 154–155, 339–349 CrossRef CAS.
- Q. L. Zhang, C. T. Qiu, H. D. Xu, T. Lin, M. C. Gong and Y. Q. Chen, Novel promoting effects of tungsten on the selective catalytic reduction of NO by NH3 over MnOx-CeO2 monolith catalyst, Catal. Commun., 2011, 16, 20–24 CrossRef CAS.
- J. Qiu, K. Zhuang, M. Lu, B. L. Xu and Y. N. Fan, The selective catalytic reduction activity of Cu/MCM-41 catalysts prepared by using the Cu2+-MCM-41 mesoporous materials with copper ions in the framework as precursors, Catal. Commun., 2013, 31, 21–24 CrossRef CAS.
- V. Parvulescu and B. L. Su, Iron, cobalt or nickel substituted MCM-41 molecular sieves for oxidation of hydrocarbons, Catal. Today, 2001, 69, 315–322 CrossRef CAS.
- B. Lindlar, A. Kogelbauer and R. Prins, Chemical, structural, and catalytic characteristics of Al-MCM-41 prepared by pH-controlled synthesis, Microporous Mesoporous Mater., 2000, 38, 167–176 CrossRef CAS.
- A. Hagen, K. Schueler and F. Roessner, The performance of Ti-MCM-41 in aqueous media and after mechanical treatment studied by in situ XANES, UV/Vis and test reactions, Microporous Mesoporous Mater., 2002, 51, 23–33 CrossRef CAS.
- A. B. Sorokin and A. Tuel, Metallophthalocyanine functionalized silicas: catalysts for the selective oxidation of aromatic compounds, Catal. Today, 2000, 57, 45–59 CrossRef CAS.
- C. C. Liu and H. S. Teng, Cu/MCM-41 for selective catalytic NO reduction with NH3—comparison of different Cu-loading methods, Appl. Catal., B, 2005, 58, 69–77 CrossRef CAS.
- Y. Wan, J. X. Ma, Z. Wang, W. Zhou and S. Kaliaguine, On the mechanism of selective catalytic reduction of NO by propylene over Cu-Al-MCM-41, Appl. Catal., B, 2005, 59, 235–242 CrossRef CAS.
- J. Qiu, K. Zhuang, M. Lu, B. L. Xu and Y. N. Fan, The selective catalytic reduction activity of Cu/MCM-41 catalysts prepared by using the Cu2+-MCM-41 mesoporous aterials with copper ions in the framework as precursors, Catal. Commun., 2013, 31, 21–24 CrossRef CAS.
- M. Ziolek, I. Sobczak, I. Nowak, P. Decyk, A. Lewandowska and J. Kujawa, Nb-containing mesoporous molecular sieves—a possible application in the catalytic processes, Microporous Mesoporous Mater., 2000, 35–36, 195–207 CrossRef CAS.
- J. R. Sohn, S. H. Lee and J. S. Lim, New solid superacid catalyst prepared by doping ZrO2 with Ce and modifying with sulfate and its catalytic activity for acid catalysis, Catal. Today, 2006, 116, 143–150 CrossRef CAS.
- S. Gao, X. B. Chen, H. Q. Wang, J. S. Mo, Z. B. Wu, Y. Liu and X. L. Weng, Ceria supported on sulfated zirconia as a superacid catalyst for selective catalytic reduction of NO with NH3, J. Colloid Interface Sci., 2013, 394, 515–521 CrossRef CAS PubMed.
- D. Pietrogiacomi, A. Magliano, P. Ciambelli, D. Sannino, M. C. Campa and V. Indovina, The effect of sulphation on the catalytic activity of CoOx/ZrO2 for NO reduction with NH3 in the presence of O2, Appl. Catal., B, 2009, 89, 33–40 CrossRef CAS.
- X. S. Du, X. M. Wang, Y. R. Chen, X. Gao and L. Zhang, Supported metal sulfates on Ce-TiOx as catalysts for NH3-SCR of NO: High resistances to SO2 and potassium, J. Ind. Eng. Chem., 2016, 36, 271–278 CrossRef CAS.
- M. Yonemitsu, Y. Tanaka and M. Iwamoto, Metal ion-planted MCM-41 2. Catalytic epoxidation of stilbene and its derivatives with tert-butyl hydroperoxide on Mn-MCM-41, J. Catal., 1998, 178, 207–213 CrossRef CAS.
- Y. Wang, Y. Ohishi, T. Shishido, Q. Zhang, W. Yang, Q. Guo, H. Wan and K. Takehira, Characterizations and catalytic properties of Cr-MCM-41 prepared by direct hydrothermal synthesis and template-ion exchange, J. Catal., 2003, 220, 347–357 CrossRef CAS.
- F. Zhang, S. W. Chan, J. E. Spanier, E. Apak and Q. Jin, Cerium oxide nanoparticles: size-selective formation and structure analysis, Appl. Phys. Lett., 2002, 80, 127–129 CrossRef CAS.
- B. Guan, H. Lin, L. Zhu, B. Tian and Z. Huang, Effect of ignition temperature for combustion synthesis on the selective catalytic reduction of NOx with NH3 over Ti0.9Ce0.05V0.05O2-δ nanocomposites catalysts prepared by solution combustion route, Chem. Eng. J., 2012, 181–182, 307–322 CrossRef CAS.
- X. N. Wei and X. Shi, Mesoporous zirconium phenylphosphonates for selective enrichment of phosphopeptides, J. Phys. Chem. C, 2014, 118, 4213–4221 CAS.
- H. Wan, J. N. Li, W. G. Yu, Z. Y. Liu, Q. Q. Zhang, W. B. Zhang and H. F. Zou, Fabrication of a novel magnetic yolk-shell Fe3O4@mTiO2@mSiO2 nanocomposite for selective enrichment of endogenous phosphopeptides from a complex sample, RSC Adv., 2014, 4, 45804–45808 RSC.
- M. Jaroniec, M. Kruk, H. Shin, R. Ryoo, Y. Sakamoto and O. Terasaki, Comprehensive characterization of highly ordered MCM-41 silicas using nitrogen adsorption, thermogravimetry, X-ray diffraction and transmission electron microscopy, Microporous Mesoporous Mater., 2001, 48, 127–134 CrossRef CAS.
- M. Thommes, B. Smarsly, M. Groenewolt, P. Ravikovitch and A. Neimark, Adsorption hysteresis of nitrogen and argon in pore networks and characterization of novel micro- and mesoporous silicas, Langmuir, 2006, 22, 756–764 CrossRef CAS PubMed.
- J. P. Wang, Z. Yan, L. L. Liu, Y. Y. Zhang, Z. T. Zhang and X. D. Wang, Low-temperature SCR of NO with NH3 over activated semi-coke composite-supported rare earth oxides, Appl. Surf. Sci., 2014, 309, 1–10 CrossRef CAS.
- L. Ilieva, G. Pantaleo, I. Ivanov, A. M. Venezia and D. Andreeva, Gold catalysts supported on CeO2 and CeO2-Al2O3 for NOx reduction by CO, Appl. Catal., B, 2006, 65, 101–109 CrossRef CAS.
- X. Q. Wang, J. A. Rodriguez, J. C. Hanson, D. Gamarra, A. Martínez-Arias and M. Fernández-García, Unusual physical and chemical properties of Cu in Ce1-xCuxO2 Oxides, J. Phys. Chem. B, 2005, 109, 19595–19603 CrossRef CAS PubMed.
- X. Q. Wang, J. A. Rodriguez, J. C. Hanson, D. Gamarra, A. Martínez-Arias and M. Fernández-García, In situ studies of the active sites for the water gas shift reaction over Cu-CeO2 catalysts: Complex interaction between metallic copper and oxygen vacancies of ceria, J. Phys. Chem. B, 2006, 110, 428–434 CrossRef CAS PubMed.
- D. W. Kwon, K. B. Nam and S. C. Hong, The role of ceria on the activity and SO2 resistance of catalysts for the selective catalytic reduction of NOx by NH3, Appl. Catal., B, 2015, 166–167, 37–44 CrossRef CAS.
- X. Gao, Y. Jiang, Y. Zhong, Z. Y. Luo and K. F. Cen, The activity and characterization of CeO2-TiO2 catalysts prepared by the sol-gel method for selective catalytic reduction of NO with NH3, J. Hazard. Mater., 2010, 174, 734–739 CrossRef CAS PubMed.
- S. Ricote, G. Jacobs, M. Milling, Y. Ji, P. M. Patterson and B. H. Davis, Low temperature water-gas shift: Characterization and testing of binary mixed oxides of ceria and zirconia promoted with Pt, Appl. Catal., A, 2006, 303, 35–47 CrossRef CAS.
- C. R. Vera, J. C. Yori and J. M. Parera, Redox properties and catalytic activity of SO42--ZrO2 catalysts for n-butane isomerization: Role of transition metal cation promoters, Appl. Catal., A, 1998, 167, 75–84 CrossRef CAS.
- F. Dury and E. Gaigneaux, The deoxygenation of benzoic acid as a probe reaction to determine the impact of superficial oxygen vacancies (isolated or twin) on the oxidation performances of Mo-based oxide catalysts, Catal. Today, 2006, 117, 46–52 CrossRef CAS.
- B. J. Dou, G. Lv, C. Wang, Q. L. Hao and K. S. Hui, Cerium doped copper/ZSM-5 catalysts used for the selective catalytic reduction of nitrogen oxide with ammonia, Chem. Eng. J., 2015, 270, 549–556 CrossRef CAS.
- Y. J. Kim, H. J. Kwon, I. Heo, I. Nam, B. K. Cho, J. W. Choung, M. Cha and G. K. Yeo, Mn-Fe/ZSM5 as a low-temperature SCR catalyst to remove NOx from diesel engine exhaust, Appl. Catal., B, 2012, 126, 9–21 CrossRef CAS.
- H. Praliaud, S. Mikhailenko, Z. Chajar and M. Primet, Surface and bulk properties of Cu-ZSM-5 and Cu/A2O3 solids during redox treatments. Correlation with the selective reduction of nitric oxide by hydrocarbons, Appl. Catal., B, 1998, 16, 359–374 CrossRef CAS.
- S. Damyanova, C. A. Perez, M. Schmal and J. M. C. Bueno, Characterization of ceria-coated alumina carrier, Appl. Catal., A, 2002, 234, 271–282 CrossRef CAS.
- Y. S. Shen, Y. F. Ma and S. M. Zhu, Promotional effect of zirconium additives on Ti0.8Ce0.2O2 for selective catalytic reduction of NO, Catal. Sci. Technol., 2012, 2, 589–599 CAS.
- S. Roy, B. Viswanath, M. S. Hegde and G. Madras, Low-temperature selective catalytic reduction of NO with NH3 over Ti0.9M0.1O2-δ (M = Cr, Mn, Fe, Co, Cu), J. Phys. Chem. C, 2008, 112, 6002–6012 CAS.
- W. Zhao, Q. Zhong, Y. X. Pan and R. Zhang, Systematic effects of S-doping on the activity of V2O5/TiO2 catalyst for low-temperature NH3-SCR, Chem. Eng. J., 2013, 228, 815–823 CrossRef CAS.
- G. S. Qi and R. T. Yang, Performance and kinetics study for low temperature SCR of NO with NH3 over MnOx-CeO2 catalyst, J. Catal., 2003, 217, 434–441 CrossRef CAS.
- G. S. Qi, R. T. Yang and R. Chang, MnOx-CeO2 mixed oxides prepared by co-Precipitation for selective catalytic reduction of NO with NH3 at low temperatures, Appl. Catal., B, 2004, 51, 93–106 CrossRef CAS.
- T. Zhang, J. Liu, D. X. Wang, Z. Zhao, Y. C. Wei, K. Cheng, G. Y. Jiang and A. J. Duan, Selective catalytic reduction of NO with NH3 over HZSM-5-supported Fe-Cu nanocomposite catalysts: the Fe-Cu bimetallic effect, Appl. Catal., B, 2014, 148–149, 520–531 CrossRef CAS.
Footnote |
† Electronic supplementary information (ESI) available. See DOI: 10.1039/c6ra09505d |
|
This journal is © The Royal Society of Chemistry 2016 |
Click here to see how this site uses Cookies. View our privacy policy here.