DOI:
10.1039/C6RA10738A
(Paper)
RSC Adv., 2016,
6, 54394-54403
Native honeybee silk membrane: a potential matrix for tissue engineering and regenerative medicine
Received
26th April 2016
, Accepted 1st June 2016
First published on 2nd June 2016
Abstract
Biomimetic natural origin biomaterials are noteworthy targets for further innovation in biomedical and tissue engineering. In this study, honeybee silk membranes (HBSM) are investigated in their native form to explore their applicability for tissue engineering. HBSMs extracted from honeybee combs were physico-chemically characterized for their surface topography, stability followed by evaluation of the biodegradation, mechanical and biological properties. Field emission scanning electron microscopy (FESEM) and atomic force microscopy (AFM) studies revealed a uniform sheet-like morphology with sub-micron pores and the presence of evenly knitted fibers in a specific pattern/alignment. Fourier transform infrared (FTIR) spectroscopy suggested the presence of a native coiled coil structural conformation. HBSMs were found to be cytocompatible and supported the proliferation of murine L929 fibroblasts, human osteosarcoma MG-63 cells and primary porcine knee chondrocytes. Cells cultured on HBSMs maintained osteogenic and chondrogenic potential as indicated by mineralization and accumulation of sulphated glycosaminoglycans (GAGs), respectively. In vitro analysis of the immune response (in terms of TNF-α release) and blood compatibility (in terms of LDH activity) further attests its possible applicability. Moreover, an in vivo subcutaneous implantation study in mice showed minimal inflammation. Taken together, this study demonstrates the potential of natural, biocompatible HBSMs as a suitable biomaterial for tissue engineering and regenerative medicine.
Introduction
In the recent years tissue engineering has escalated the development of functional alternatives for damaged tissues by employing the principles of engineering and biology.1 It has risen as a promising approach to create functional engineered tissues and organs using cells and biomaterials.1–3 A wide range of biomaterials such as poly-caprolactone, poly(ethylene glycol), ceramics, and metals have been extensively used as matrices in regenerative medicine.4,5 These biomaterials demonstrate suitable physical properties like mechanical strength, density and viscosity for various organ or tissue specific applications. However, they lack the indispensable biological cues, which facilitates cell proliferation and differentiation to regain tissue functions.4 Recently, silkworm silk has been widely used as a potent biomaterial of choice for tissue engineering purposes. Apart from being highly cytocompatible, silk is known to possess excellent mechanical and bio-physical properties.5,6 Various silk formats like scaffolds, films, hydrogels and membranes have been prepared with ease from raw silk, which provide suitable niche for growing a variety of cells.6,7
Silk protein is mainly reported from selected group of organisms like silkworm, spider and hornet. Apart from these, honeybee are known to produce silk and has drawn great interest amongst researchers worldwide. The major constraint of honeybee silk is its lower yield and cumbersome extraction methodology. Owing to this, most research has been limited to its recombinant forms.8–18 Honeybee comb is presently the prime source for isolation of natural HBSMs. These combs are made up of compartments, which are joined together in a hexagonal arrangement and are primarily composed of wax and silk.17,19,20 Worker bees first construct compartments from wax followed by filling these compartments with fertilized eggs. After larva formation, salivary glands of the larva produce silk protein, which is secreted into the gland lumen to form a highly concentrated protein.17,19,20 Before advancing to pupae stage, these larvae wrap themselves with this concentrated protein. After growing adult, bees leave these compartments and worker bees cover this silk membrane with wax.
Honeybee silk plays a major role in the enhancement of mechanical properties of honeybee comb. It is mainly composed of four fibrous and non-repetitive proteins (approximately 32 kDa), which forms a heterotetrameric structure. These structures play a vital role in silk synthesis and function. The fibrous proteins with similar structure have equivalent mechanical properties with tensile elastic modulus of 6.5 GPa, tensile strength of 164.4 MPa, breaking strain of 3.8% and anisotropic ratio of longitudinal moduli to transverse as 18.9, representing its strong and highly flexible nature.10,17,18,21 The presence of high alanine content in honeybee silk results in a very close spacing of helices which forms very tight coiled coils.8 The coiled coil structures of honeybee silk are formed by interaction of helices and contain an insignificant amount of β-sheet structure.8–10,12–18,22
Silkworm silk biomaterial has been extensively used as scaffolding matrix to support various types of cell lineages including stem cells. These matrices efficiently support cell adhesion, proliferation and differentiation to various lineages by providing a suitable niche.5,6 Similarly, recombinant honeybee silk membranes have also been reported to support such cell adherence and proliferation.4 Honeybee silk (recombinant) has been studied for its mechanical and structural properties and is well-known for its attractive physical and chemical properties.4,8,10 However, its utilization as a biomaterial in its native form is still unexplored. Silk biomaterials are mostly derived from regenerated or recombinant silk, however use of native silk biomaterials is limited. The membranes obtained from honeybee comb, as reported herein, provide an opportunity to study native honeybee silk for its potential utilization as a biomaterial. In our current endeavour, we attempted to explore the potential of HBSMs without altering its native protein structure. We isolated pristine HBSMs from honeybee comb, which is treated as waste after extraction of valuable honey and wax. Moreover the processing approach to obtain HBSMs requires minimal use of solvents.
Experimental
Isolation of HBSMs from beehive
Honeybee comb from beehive of Indian honeybee Apis cerana indica was used for current study. Comb was washed extensively in warm water (55–60 °C) for 15 min to remove water soluble contaminants/pigments and dried overnight. Dried honeybee comb was dipped in chloroform (100% v/v) and incubated in a shaker incubator (180 rpm) overnight at 37 °C. This washing step was repeated thrice to allow efficient wax removal from the comb. Remaining mass contained mainly raw honeybee silk.8 After washing, individual comb membranes were manually separated into distinct outer, middle and inner membranes. These individual HBSMs were further rinsed with chloroform overnight to remove residual wax. Finally, membranes were treated with acetone (100% v/v) for 48 h to remove remaining traces of wax (Fig. 1). Membranes were stored at 25 °C for further use.
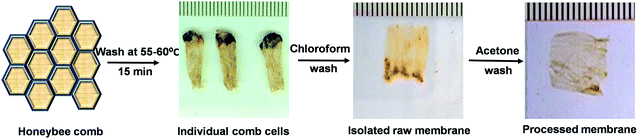 |
| Fig. 1 Scheme representing the processing of HBSM to obtain clear membrane. | |
Fourier transformed infrared (FTIR) spectroscopy
Infrared spectroscopy of HBSM was performed with a FTIR spectrometer (Fisher Scientific) and each measurement incorporated 32 scans at a resolution of 4 cm−1. Secondary structures were assigned as described previously.4 Absorption bands between 1610 and 1625 cm−1 were assigned as β-sheet structure; frequency of 1630, 1640 and 1650 cm−1 were assigned as coiled-coil structure while the frequency of 1660 cm−1 were assigned as β turns.4 Amide I, II and III regions were assigned between 1700 and 1600 cm−1, 1600 to 1500 cm−1 and 1350 to 1200 cm−1 respectively.23
Field emission scanning electron microscopy (FESEM) of HBSMs
Surface topography of HBSMs was examined by field emission scanning electron microscope (FESEM, Zeiss, Sigma) at an operating voltage of 5 kV. Samples were sputter coated with gold particles using sputter coater and images were captured.
Atomic force microscopy (AFM)
Surface analysis of HBSM was performed by AFM (Agilent, Model 5500) in non-contact mode with tips mounted on cantilevers and spring constant 40 N m−1. Membranes were placed onto glass cover slip and air-dried. Measurements were done at room temperature (RT, ∼25 °C) with pre-specified scan area. Three different areas of HBSMs were scanned and results are presented as average of 3 values.
Mechanical strength analysis
Tensile strength of HBSM was measured using Universal Testing Machine (Instron 3343) equipped with 100 N load cell at ambient conditions (25 °C and 80–85% relative humidity). The crosshead speed used was 1 mm min−1. Four individual dry honeybee sacs were cut open to form uniform membranes. The thickness of each membrane was measured using digital caliper (World Precision Instruments, Inc., USA) and taken as an average of four measurements. Membranes with 5.75 ± 0.5 mm length, 5.25 ± 0.5 mm breadth and 30 μm thickness were used for mechanical study.
Degradation profile of HBSMs
HBSM biodegradability was determined by in vitro digestion using protease type XIV (Sigma-Aldrich, USA). HBSMs were incubated with protease enzyme (1 U ml−1) prepared in phosphate buffered saline (PBS, pH 7.4, with 0.05% sodium azide). Control HBSMs were dipped in PBS without enzyme.
After every 72 h, enzyme solution was replenished with fresh enzyme. At pre-selected time points (viz. 1, 7, 14, 21 and 28 days) enzyme solution was removed completely and membranes were allowed to dry for 24 h at RT. For degradation studies, samples were placed in incubator maintained at 37 °C. The dry sample weight was recorded and % mass remaining was calculated by the formula:
|
% mass remaining = [Wt/Wi] × 100
| (1) |
where
Wi is the initial dry weight of HBSMs and
Wt is the final dry weight after predefined time point.
Solubility and integral stability of HBSMs
Solubility and integral stability of membranes were evaluated by estimating the cumulative protein released in PBS. Outer and inner membranes were incubated at 4 °C in 5 ml PBS and left for 135 days. Cumulative protein release was estimated on day 90, 120 and 135 by Bradford protein estimation (Sigma-Aldrich, USA) using manufacturer's protocol. Briefly, HBSM released protein was collected and mixed with Bradford reagent. The absorbance was measured at 595 nm using a multiplate reader (Tecan infinite M 200) and released protein amount was calculated from standard curve plotted against bovine serum albumin (BSA, Sigma Aldrich, USA).
Cell culture and in vitro cell proliferation assay on HBSMs
Cell culture and maintenance. For cell culture experiments, L929 murine fibroblast, MG-63 human osteosarcoma and porcine primary chondrocyte cells were used. L929 fibroblast and MG-63 cells were procured from National Centre for Cell Sciences (NCCS, Pune, India). Porcine chondrocytes were isolated from knee joint cartilage tissue obtained from local slaughter house under sterile condition. For further experiments, L929 fibroblasts and chondrocytes were cultured in high glucose Dulbecco's Modified Eagle Medium (DMEM, Gibco, USA) supplemented with 10% fetal bovine serum (FBS, Gibco, USA) and antibiotic–antimycotic solution (Himedia). Cells were maintained at 37 °C in a humidified incubator with 5% CO2. MG-63 cells were maintained in Minimum Essential Medium (MEM, Gibco, USA), 10% FBS and antibiotics cocktail. After attaining 80–90% confluence culture, cells were trypsinized with 0.25% trypsin–EDTA (Gibco, USA) and used for further in vitro experiments.
Alamar blue cell proliferation assay. Cell proliferation study was performed to evaluate the cytocompatibility of the isolated HBSMs. The assay was carried out with L929 murine fibroblasts, MG-63 osteosarcoma cells and primary porcine chondrocytes. Middle layer was taken as a representative membrane to demonstrate cell proliferation and differentiation ability. Membranes (1.5 × 1.5 cm2) were autoclaved and pre-conditioned in culture media overnight. Cells were seeded at a density 104 cells per membrane in a 12 well tissue culture plate. Cell proliferation was monitored using alamar blue dye (Invitrogen, USA), a highly sensitive aqueous fluorescent dye. It does not affect cellular proliferation and viability. On pre-defined days, cells cultured on HBSMs were incubated in medium supplemented with 10% (v/v) alamar dye for 3 h. After incubation, 100 μl media was taken in a 96 well plate and absorbance was read at 570 and 600 nm using a multiplate reader (Tecan infinite M 200). Membranes without cells were used as negative control.
Live/dead cell viability assay. Cell adherence and spreading was assessed using live/dead assay. All three types of cells were seeded on HBSMs and incubated in a humidified atmosphere with 5% CO2 at 37 °C. After 7 days of culture, cell seeded HBSMs were rinsed with PBS (pH 7.4) and incubated in live/dead staining solution (consisting of 4 μM calcein AM and 2 μM ethidium homodimer-1 in PBS, Sigma Aldrich, USA) for 15–20 min at 37 °C. Further, images were captured under fluorescence inverted microscope (EVOS FL, Life Technologies, USA). Viable cells convert calcein AM to calcein in the presence of intracellular esterase activity and fluoresce green. Ethidium homodimer-1 enters into dead cells through damaged membrane, binds with deoxyribonucleic acid (DNA) and fluoresces red. In another set of staining, cells were stained with Hoechst 33342 dye (Sigma-Aldrich, USA) following manufacturer's instruction. Hoechst 33342 binds with DNA and fluoresces blue showing nucleus.
Osteogenic differentiation and alizarin red staining for calcium deposition. HBSMs were evaluated for osteogenic differentiation potential of human osteosarcoma MG-63 cells in presence of osteogenic media. Cells were seeded on pre-conditioned HBSMs at a density 104 cells per membrane and cultured for 7 days. Osteogenic media comprising of MEM with 10% FBS, 10 mM sodium β-glycerol phosphate (Sigma-Aldrich, USA), 50 μg ml−1 L-ascorbic acid (Sigma-Aldrich, USA), 10−8 M dexamethasone (Sigma-Aldrich, USA), and antibiotics was used.24,25 Following incubation for 7 days, the membranes were assessed for calcium deposition.To determine osteogenic differentiation potential, calcium deposition was monitored using alizarin red stain (Sigma-Aldrich, USA). 2% (w/v) alizarin stain solution was prepared by dissolving alizarin powder in distilled water. Stain solution was maintained at pH between 4.1 and 4.3 using ammonium hydroxide (NH4OH) solution. HBSMs with cells were rinsed in distilled water followed by staining in alizarin stain solution for 2 min. Excess stain was washed off. HBSMs were dipped in acetone followed by acetone–xylene solution.24 Positive reactivity of staining for osteogenesis was documented by photomicroscopy using bright field illumination.
Chondrogenic maintenance and sulphated glycosaminoglycans (sGAGs) assay. HBSMs were evaluated for maintaining chondrogenic behaviour of porcine primary chondrocytes when cultured in chondrogenic media. Chondrocytes were seeded on HBSMs at a density of 104 cells per membrane and cultured in chondrogenic media consisting of high glucose DMEM with 10% FBS, antibiotics, 0.25 μg ml−1 amphotericin B (Invitrogen, USA), 100 nM dexamethasone, 10 ng ml−1 TGF-β1, 50 μg ml−1 L-ascorbic acid, 1 mM sodium pyruvate, 1 X ITS+3 (containing 1.0 mg ml−1 insulin from bovine pancreas, 0.55 mg ml−1 human transferrin, 0.5 μg ml−1 sodium selenite, 470 μg ml−1 linoleic acid, 470 μg ml−1 oleic acid and 50 mg ml−1 bovine serum albumin), 2 mM L-glutamine and 40 μg ml−1 L-proline (Sigma-Aldrich, USA) for 7 days.26 Media was replenished thrice a week. In order to visualize sulphated GAGs deposition on HBSMs, alcian blue (Sigma-Aldrich, USA) staining was performed. Cell seeded membranes were fixed in 10% neutral buffered formalin (Sigma Aldrich, USA) overnight. HBSMs were then washed in PBS and further stained with 0.1% alcian blue in 0.4 M MgCl2, 0.025 M sodium acetate (pH 5.6).27 Positive reactivity with GAGs staining was visualized using bright field microscope.
Immunological assessment of HBSM
Macrophage stimulation assay. Immunogenicity of HBSMs isolated from beehive was assessed by the release of tumor necrosis factor alpha (TNF-α). TNF-α is a key player in inflammation and regulation of immunity. It is secreted by leukocytes and other immune cells in response to infections. Short (01 day) and long-term (07 day) studies with RAW 264.7 cells (mouse macrophage cell line) were performed.24,28 Macrophage stimulation by HBSMs was carried out with the following protocol. RAW 264.7 macrophage cells were cultured on HBSMs at a density of 5 × 104 cells per membrane. In a parallel set, Bombyx mori silk films, prepared from 1% w/v fibroin protein, were tested to compare with HBSMs. Uncoated standard tissue culture plate with similar cell density and plates containing 1000 ng ml−1 lipopolysaccharide (LPS) from Escherichia coli (Sigma-Aldrich, USA) were taken as negative and positive controls, respectively.
TNF-α in vitro inflammatory response of HBSMs. The immunogenicity of HBSMs was determined by quantifying TNF-α release using mouse TNF-α ELISA kit (Invitrogen, USA) as per manufacturer's protocol. Accordingly, cell supernatants were collected after specified period of incubation (day 1 and 7). 50 μl of sample and the recombinant TNF-α standard (0–1000 pg ml−1) were added in triplicate in a 96 well plate coated with antibody (anti-mouse TNF-α). Further, 50 μl of secondary antibody was added to the wells and incubated for 90 min at RT. After incubation, wells were washed four times with wash buffer followed by addition of 100 μl of streptavidin–HRP working solution. The plate was incubated at RT for 30 min. Further, wells were washed four times followed by addition of 100 μl of stabilized chromogen (TMB) for 30 min at RT. After incubation, 100 μl stop solution was added and absorbance was read at 450 nm using a multiplate reader (Tecan infinite M 200). TNF-α release by cells was determined from standard graphs.
In vitro blood compatibility of HBSMs. Antithrombogenic property and blood compatibility of HBSM was determined by number of platelets adhered to membranes as described previously.29,30 In brief, whole blood from healthy porcine was collected and 5 U ml−1 of heparin (Sigma-Aldrich, USA) was added instantly. Fresh whole blood was centrifuged at 150g for 15 min at 22 °C to isolate platelet rich plasma (PRP). Supernatant was used for further studies. HBSMs of similar sizes were taken in a 24 well plate whereas collagen type I (from rat tail, 1.5 mg ml−1, Sigma-Aldrich, USA) coated wells were considered as positive controls. Analysis of platelet adhesion was done by incubating 300 μl PRP with samples for 1 h at room temperature (RT) in an orbital shaker at 60 rpm. Subsequently, HBSM were washed with PBS (pH 7.4) thrice. For visualization of adhered platelets, they were fixed in Neutral Buffered Formalin (NBF, Sigma-Aldrich, USA) overnight followed by 10 min treatment with 0.1% Triton X-100 (Sigma-Aldrich, USA) in PBS. Post permeabilization, adhered platelets were stained with rhodamine–phalloidin (1
:
40 in PBS, Life Technologies, USA). An inverted fluorescent microscope (EVOS FL, Life Technologies, USA) was used to visualize stained platelets.Quantitative measurement of platelet activity adhered onto HBSM and collagen coated wells was performed by analyzing lactate dehydrogenase (LDH) activity. Adhered platelets were lysed with 1% Triton X-100 for 1 h at 37 °C. Following Triton X-100 treatment, platelet lysate was centrifuged at 10
000 rpm (4 °C). Supernatant was collected for LDH activity measurement. LDH assay kit (Sigma-Aldrich, USA) was used for measurement following the manufacturer's protocol and reported as milliunits per ml.
In vivo immunogenic response to HBSMs. All in vivo experiments were performed as per the animal care protocols approved by West Bengal University of Animal and Fishery Sciences (WBUAFS), Kolkata, India in accordance with “Principles of laboratory animal care”. The animals used in this study were Swiss (I.B.) mice of 30–35 g body weight of either sex. The mice were examined for 4 weeks post in vivo transplantation.31 Experimental mice were subcutaneously implanted with acellular HBSMs (1.5 × 1.5 cm2). All samples were inserted through a 0.5 cm incision in a subcutaneous pocket of lateral side of thoraco-lumbar region. The surgery was conducted under general anaesthesia using isoflurane (1–3% in oxygen). The wound healing process at the incision site was monitored during the 4 weeks study and no death was reported during evaluation period. In order to evaluate the inflammatory responses, mice were sacrificed by cervical dislocation. Further, samples were collected along with the overlying native tissue and histological examination was performed. Briefly, harvested membranes were fixed in 10% neutral buffered formalin for 24 h. Further, membranes were dehydrated using graded ethanol and embedded in paraffin cassettes. Paraffin blocks were sectioned with 5 μm thicknesses having membrane in vertical orientation. Sections were further deparaffinized and rehydrated using graded ethanol (100, 90, 80, 70, and 50% v/v in Milli-Q water). Slices were stained with hematoxylin and eosin (H&E) and histological sections were assessed for the infiltration of inflammatory cells to reveal cellular response of host to the implant.
Statistical analysis. All quantitative experiments were run atleast in triplicate and results are expressed as mean ± standard deviation for n = 3, unless noted otherwise. Statistical analysis was performed by one-way analysis of variance (ANOVA). Values with *p ≤ 0.05 are considered as significant whereas **p ≤ 0.01 as highly significant. All statistical analyses were executed using Microcal OriginPro 8.
Results
Secondary structure of HBSMs
Honeybee silk conformation in HBSMs was investigated by FTIR spectroscopy. As represented in Fig. 2, one sharp peak at 1651 cm−1 in amide I region and another peak at 1548 cm−1 in amide II region was observed. Peak ranging from 1630 cm−1 to 1650 cm−1 in amide I region represents coiled coil structure, which signifies predominance of coiled coil structure within honeybee silk. Presence of similar structures are known to be resulted from interaction between helical strands.13–15,22
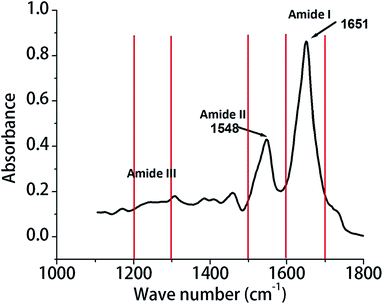 |
| Fig. 2 FTIR spectra of natural HBSM extracted from honeybee comb. | |
Analysis of surface morphology
Outer and inner membranes were analyzed using field emission scanning electron microscope. As reported earlier, honeybee silk is known to be present in both fibrous and sheet form.8 As observed in FESEM images, it is evident that HBSMs are sheet like structures where fibers were finely knitted together (Fig. 3A). The membranes were inherently patterned whereas fibers were aligned in a particular direction with grooves. Magnified image showed HBSMs to be highly porous with sub-micron pore size (Fig. 3A).
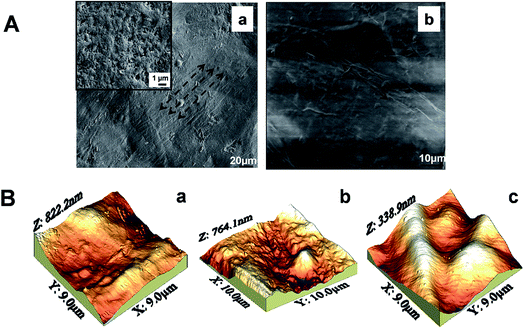 |
| Fig. 3 (A) Scanning electron micrograph of HBSMs showing morphology and porosity. (a) Inner membrane (scale: 20 μm), inset image showing the magnified view of inner membrane (scale: 1 μm) exhibiting the porous membrane structure and (b) middle membrane (scale: 10 μm) (black arrows indicate intricate network of fibers aligned uniformly). (B) Surface morphology analysis with 3D reconstructed AFM images of natural HBSMs. (a) Inner membrane, (b) middle membrane and (c) outer membrane. | |
AFM analysis of HBSMs further indicated tightly joined silk fibers aligned in a specific direction (Fig. 3B). The average roughness of outer, middle and inner membrane was 62.34 ± 3.52 nm, 119.22 ± 5.67 nm and 98.84 ± 4.98 nm, respectively. Similarly, RMS roughness of outer, middle and inner membrane was 74.95 ± 2.76 nm, 144.52 ± 8.25 nm and 122 ± 9.42 nm, respectively. Further, average height of peaks was found to be 178.5 ± 15.29 nm, 368.82 ± 18.65 nm and 360.2 ± 22.47 nm, respectively (Fig. 3B).
Mechanical properties of HBSMs
Mechanical integrity of biomaterial is one of the crucial factors and determines its performance under in vitro/in vivo conditions. The tensile strength of native HBSM after isolation and processing was found to be 777.5 ± 68 kPa. High tensile strength may be attributed to its highly oriented and closely knitted feature as confirmed by morphological studies.
In vitro proteolytic degradation and membrane stability
The inherent ability of a material to degrade with time is an important parameter for its prospective use in various tissue engineering applications. Biodegradability of HBSMs was analyzed using protease type XIV enzyme for 28 days residual weight was measured with an interval of 7 days. Fig. 4A represents loss of weight (∼30%) in control samples overtime. In enzyme treated membranes, there was a significant reduction in weight ∼85% after 28 days of incubation (p ≤ 0.01). Majorly the membranes fragmented within first couple of weeks with a mass loss of ∼75%.
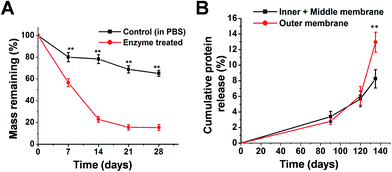 |
| Fig. 4 Membrane degradation and stability. (A) Graph showing degradation pattern of natural HBSMs in enzyme and PBS. Data expressed as % mass remaining. (B) Membrane stability test showing percent cumulative protein release profile for 135 days. Data expressed as percent cumulative protein release. Data represents mean ± standard deviation (n = 3), where **p ≤ 0.01. | |
Similarly, solubility and integral stability study was conducted to evaluate the long-term storage of the membranes incubated at 4 °C in PBS in absence of enzymes (pH 7.4). Protein released was determined by Bradford assay. As shown in Fig. 4B, minimal amount of cumulative protein release (∼3%) was observed from membranes after 90 days of incubation. Cumulative protein release slightly increased with time amounting to ∼6% after 120 days of incubation. On day 135, total protein released from the outer membrane (∼13%) was slightly higher than inner membrane (∼8%) (p ≤ 0.01).
Cytocompatibility studies
Cell proliferation study was conducted to assess the cytocompatibility of membranes. Cell viability on HBSM was observed till 7 days of culture (Fig. 5). All cell types showed enhanced proliferation with time. There was a significant difference in cell proliferation rate on day 7 as compared to day 1 (p ≤ 0.01). Nearly 2 fold increase in fibroblast proliferation rate was observed within a short period of 7 days (p ≤ 0.01).
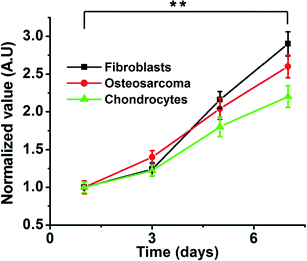 |
| Fig. 5 Cell proliferation on HBSMs at different time intervals with L929 fibroblast, MG-63 osteosarcoma and primary porcine chondrocyte cells. Data represents mean ± standard deviation (n = 3), where **p ≤ 0.01. | |
Similarly, for MG-63 osteosarcoma cells, increase in cell proliferation was ∼1.6 fold (p ≤ 0.01). Primary porcine chondrocytes also showed ∼1.2 fold increase (p ≤ 0.01). These differences in cell proliferation rates amongst all cell types can be attributed to their natural pace of proliferation as per their source of origin. Increased proliferation rate of different cell types suggest the membranes to be cytocompatible.
In addition to cell proliferation, live/dead assay demonstrated the cellular adherence, spreading and viability after 7 days of culture (Fig. 6). Apart from enhanced proliferation, cells were observed to be well spread out onto HBSMs. Cells maintained their native spindle (fibroblasts/MG-63) and polygonal (chondrocytes) morphology similar to standard tissue culture well plates (TCP) (Fig. 6).
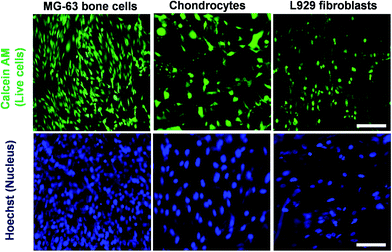 |
| Fig. 6 Microscopic images of MG-63 bone cells, porcine chondrocytes and L929 fibroblast cells growing on HBSMs showing material cytocompatibility. Images were taken after 7 days culture. Scale bar represents 100 μm. | |
Osteogenic differentiation, alizarin red staining for calcium deposition
Alizarin red staining was performed to confirm the signature characteristic of the osteogenic differentiation i.e. calcium deposition on HBSMs that can be visualized as extracellular mineralized nodule deposition. MG63 cell seeded HBSMs were stained with alizarin stain after 7 days in vitro culture in osteogenic media. The microscopic images clearly illustrated osteogenic differentiation of cells onto membranes as indicated by extensive mineralization (formation of red nodules). Results were comparable with cells cultured on standard TCP (Fig. 7).
 |
| Fig. 7 Assessment of cellular functionality by alizarin red staining (with MG-63 cell) and alcian blue (with primary porcine chondrocyte). Cells were grown in osteogenic (for MG-63) and chondrogenic (for chondrocytes) media and staining was done on culture day 7. Scale bar represents 100 μm. | |
Deposition of sulphated glycosaminoglycans (sGAGs) by primary chondrocytes
Alcian blue staining was done to evaluate the maintenance of chondrogenic functionality of primary chondrocytes cultured on HBSMs. Extracellular deposition of sulphated GAGs is hallmark of chondrocyte maintenance and proliferation. HBSMs, seeded with chondrocytes were stained with alcian blue after 7 days in vitro culture in chondrogenic media. The localization of GAG was clearly visualized as extracellular depositions on the matrix within periphery of cells (Fig. 7). The microscopic images showed chondrogenic maintenance on the membranes as indicated by extensive sGAGs deposition. Deposited GAGs were evident all over the membranes as intense blue colour staining similar to positive control (TCP).
In vitro blood compatibility of HBSMs. Hemocompatibility and anti-thrombogenic activity of HBSMs were assessed by comparing the amount of platelets adhered onto HBSMs relative to collagen coated coverslips (positive control). Fluorescent microscopic images demonstrated considerably higher amount of platelets adherence to collagen coated coverslips whereas HBSMs were found to be minimally thrombogenic (as indicated by reduced platelet adhesion, Fig. 8A). Another noticeable point is formation of platelet aggregates with spreaded morphology on collagen substrate indicating thrombogenicity of these surfaces. On the contrary, no such platelet aggregates were observed on HBSMs attesting their low thrombogenic behaviour.32 For quantification of the same, LDH activity of adhered platelets was measured. Results were in accordance with the platelet adhesion images where 6.33 ± 0.45 milliunits per ml LDH activity was obtained for collagen coated surfaces. On evaluating the LDH activity for HBSM, significantly lesser (4.27 ± 0.93 milliunits per ml, p ≤ 0.05) LDH activity was obtained (Fig. 8B).
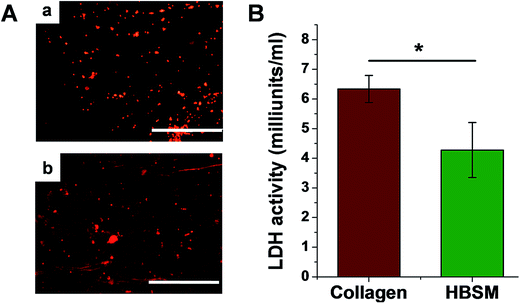 |
| Fig. 8 Analysis of hemocompatibility and antithrombogenic activity of honeybee mat. (A) Images depicting adhesion of platelets on (a) collagen coated coverslip and (b) honey bee mat after incubation with PRP. Adhered platelets were stained with rhodamine–phalloidin (red color) and visualized under fluorescent microscope. (B) Activity of adhered platelets in terms of LDH activity for both the substrates (*p ≤ 0.05). Scale bar 200 μm. | |
TNF-α in vitro inflammatory response to HBSMs
TNF-α (a pro-inflammatory cytokine) plays a pivotal role in regulation of inflammation. It is produced by leukocytes, activated macrophages, and other cells in response to infectious agents. TNF-α release study was conducted over a period of 7 days using RAW 264.7 macrophage cell line (Fig. 9A). On day 1, macrophage cells growing on HBSMs evoked minimal levels of TNF-α as compared to positive control (LPS) (p ≤ 0.05). These values were comparable to negative control i.e. tissue culture plate (TCP) (Fig. 8). After 7 day culture, TNF-α release was very high for positive control (p ≤ 0.01). On HBSMs, the release was observed to be significantly lower and comparable to negative control TCP (p ≤ 0.01), suggesting lower levels of immunogenic responses from HBSMs.
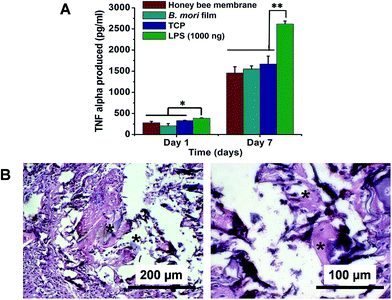 |
| Fig. 9 Analysis of in vitro and in vivo response to HBSMs (A) TNF-α production by RAW 264.7 macrophages. TNF-α released was estimated from a standard graph using recombinant TNF-α. (B) Representative images of hematoxylin and eosin (H and E) staining of the retrieved HBSMs after 4 weeks of subcutaneous implantation in mice. Degraded fragments of HBSMs were observed after 4 weeks (indicated as *). Scale bar represents 200 μm. Data represents mean ± standard deviation (n = 3), where *p ≤ 0.05 and **p ≤ 0.01. | |
In vivo response to HBSMs
In order to study implant integration and material immune response in in vivo conditions, HBSMs were implanted subcutaneously in mice and were retrieved after 4 weeks (Fig. 9B). Following implant retrieval, histology sections were stained with hematoxylin and eosin.31 Microscopic images suggested mild immune response as indicated by infiltration of fewer immune cells surrounding the HBSMs. On closer evaluation, membranes were found to be fragmented (indicated with *, Fig. 9B) within 4 weeks under in vivo conditions. Results were in accordance with in vitro proteolytic degradation study.
Discussion
A successful strategy to develop a novel biomaterial involves the overall evaluation of a material. In this study, with an aim to project the native honeybee silk membranes as a potential biomaterial, we evaluated its various physical, chemical and biological attributes. These silk membranes were obtained directly in its native form from honeybee combs without going through regeneration or recombinant route. Further, this isolation process involves minimal usage of solvents without using any high end specialized equipment. Similarly, structural and morphological properties of a biomaterial are amongst the prime aspects to decide its applications.6 Material properties are known to affect cell adhesion to matrix, which ultimately influence cell spreading, proliferation and differentiation.33 FTIR studies (Fig. 2) revealed only one peak in amide I region, suggesting predominance of coiled coil secondary structures within HBSMs. This result is similar to already published reports for native, regenerated and recombinant honeybee silk protein suggesting protein secondary structure to remain unaltered even after heating at 50–60 °C and subsequent treatments with organic solvents like chloroform and acetone.8–10 Structural properties like porosity and surface roughness are known to play a major role in nutrient and waste transfer,6 cell adhesion, cell migration, cell–surface interactions and cell–cell communication. FESEM studies showed HBSMs' fibers to be closely knitted with micron range pores all over the surface (Fig. 3A). These pores may help in trans-lamellar transport and diffusion of nutrients. It may also aid in enhancing cell–cell interactions. In natural ECM, nanostructure plays a very important role for cell adhesion and proliferation.33 Thus, the presence of nanoscale surface roughness within biomaterials is expected to influence the cell adhesion and proliferation.6,33 AFM studies of HBSMs showed average surface roughness to be 60–119 nm that might be well suited for cell adhesion and proliferation (Fig. 3B). These structural properties project HBSMs as biomaterial, which closely mimics natural ECM thus providing the suitable environment cues for enhanced cell adhesion, spreading and proliferation.
Mechanical strength of these membranes (777.5 ± 68 kPa) suggests that silk being the main component, provides bulk mechanical strength to the mats making it suitable for various tissue engineering applications.
The tensile strength of the native membrane is comparable to various composite biomaterials used for cell culture and tissue engineering applications. As evident from AFM and FESEM images (Fig. 3), the well knitted condensed fiber networks in HBSMs might be the plausible reason for mechanical integrity of these membranes. Moreover, the submicron sized pores on the surface might help in uniform distribution of force throughout the membrane surface and pose a barrier to cracking.34
In addition to structural properties, inherent ability of a material to degrade with time plays an important role in biomaterial applications. Biodegradable material must degrade with time without producing any toxic end-product. Further, if the non-toxic end-products are easy to metabolize by cells themselves is an added advantage as it may serve as a source of energy.35 In vitro proteolytic degradation studies showed that the membranes are degradable over time (Fig. 4A). As shown in degradation graph (Fig. 4A), protease type XIV cleaved the protein chains present within membranes gradually and degrading up to ∼85% of its own weight within 4 weeks. Similarly, in control samples, ∼30% degradation was observed probably due to susceptibility of protein at 37 °C incubation even in presence of buffer (without enzyme) (Fig. 4A). When incubated at 4 °C, the rate of protein degradation was drastically reduced and ∼8–13% weight loss of total protein content, even after 135 days of incubation (without enzyme), suggested protein stability (Fig. 4B). Thus, the membranes may be isolated and ideally be stored for long term usage under ambient conditions; another advantage as a biomaterial. Our degradation results are further supported by similar previous studies on recombinant honeybee silk, showing degradation in presence of enzymes releasing small peptides as end-products without accumulation of partial degradation products.4
Enhanced cell proliferation on these membranes, as supported by cell viability studies, shows its cytocompatibility (Fig. 5). This is well supported by previous similar studies with different cell types cultured on regenerated/recombinant honeybee silk4 and silkworm silks.2,3,6,24–27,36 It further supports our hypothesis that HBSMs will be a potential substrate for culture of a wide range of cell types ranging from cell lines and primary cells to stem cells (Fig. 6). Typically for MG-63 cells, highly confluent cell monolayer was observed throughout the membranes and cells maintained native spindle morphology. Similarly for L929 fibroblasts and primary porcine chondrocytes, native morphology i.e. spindle and polygonal shape was maintained and cells were evenly distributed on membranes. In addition, HBSMs also supported the osteogenic differentiation as observed by calcium deposition. It also provided the suitable environment for the chondrogenic maintenance as shown in Fig. 7. Production of GAGs and mineralized bone are promising aspects of a biomaterial and relate to earlier studies with silkworm silk.24–27 One of the crucial factors for enhanced cell adherence and proliferation on HBSMs might be its structural properties such as surface roughness, patterns and grooves. The specialized nanoscale structure of HBSM, as evident from AFM and SEM images (Fig. 3), might have provided higher effective binding surface for cells to adhere thus influencing its proliferation ability. Further, the presence of submicron sized pores over membrane as confirmed with SEM pictographs might help in effective transfer of nutrients and wastes across the membranes during culture. Promising cell viability results clearly suggests that the membranes are ideally suited for cell culture studies.
Compatibility of HBSMs to blood is an important aspect that would further widen the scope of its applications. Minimal platelet adhesion and LDH activity clearly validates the lower thrombogenic property of HBSMs29 (Fig. 8). Membrane utilization for vascular applications would be interesting where contact to blood is inevitable. The lower response to inflammatory cells is pre requisite for any material intended for implantation. As depicted from the results of in vitro TNF-α release (Fig. 9A) and in vivo implantation studies (Fig. 9B), HBSMs evoked minimal inflammatory response, which suggests the suitability of HBSMs for various in vivo applications.36,37 In vitro immune response of honeybee membranes (in terms of TNF-α release) was comparable with well reported and FDA approved B. mori silk fibroin membranes and to conventional tissue culture plates (TCP). Moreover, these membranes were found to degrade under in vivo conditions within 4 weeks owing to presence of coiled coil structure. Results were in accordance with degradation data, where presence of protease enzyme degraded the membranes within 28 days. Degradation of implanted biomaterial is an important aspect where graft is expected to be replaced with native tissue over time. Crucial point in this regard is that the degraded product should be minimally immunogenic. Fragmented membranes implanted in mice subcutaneous pocket elicited minimal immune response as indicated by presence of fewer attached immune cells (Fig. 9B). Similar to silk and other potential membranes, HBSMs may be utilized as substrate for enzyme immobilization in biosensor applications and development of portable and implantable biomedical aid as these membranes are biocompatible, flexible and stable for long-term.38 Currently, honeybee silk is generated mainly through recombinant method followed by protein purification using nickel agarose affinity chromatography and FPLC. These processes often include convoluted steps and require expensive reagents and instruments, while native HBSM isolation is relatively easy and utilizes minimum reagents. In earlier study, poly(ethylene oxide) (PEO) was blended with honeybee silk to fabricate electrospun honeybee silk fibers as it was difficult to obtain pure honeybee silk fibers followed by methanol treatment which lead to changes in structural properties.4 However, native honeybee silk membrane utilized in this work retains the natural honeybee silk structure and the natural pattern appearance onto the surface, which mimics the natural extracellular matrix. These favorable properties attest the suitability of native honey bee membranes as potential candidate for various tissue engineering applications. As a matter of fact, our study might have played a pivotal role in introducing these native HBSMs as a potential biomaterial with many ideal characteristics. Ease in isolation, widespread availability and cost effectiveness of HBSMs would project it as a potent future alternative biomaterial.
Conclusion
Our preliminary study demonstrates for the first time, the potential of natural native honeybee silk membrane as substrate for cell proliferation and differentiation. Using facile methodology, the membranes could be isolated and processed to an ideal cell culture matrix keeping its native coiled coil protein structure intact. Cell studies using L929 fibroblast, MG-63 osteosarcoma and porcine primary chondrocytes exhibited promising outcomes with high rates of proliferation and maintenance of native morphology and function. Further, osteogenic and chondrogenic potential on honeybee membranes was demonstrated using MG-63 and porcine knee chondrocytes, which supported differentiation with enhanced production of calcium nodules and sGAG, respectively. Hemocompatibility and anti-thrombogenicity studies confirmed its suitability as an suitable implantable matrix. Further, in vitro macrophage stimulation assay with low TNF-α production indicated minimal immunogenicity supported by in vivo subcutaneous implantation on mice with lesser inflammatory response. Taken together, native HBSM with nano-range surface roughness; promising tensile strength along with enhanced cell proliferation and differentiation ability shows its immense potential as a future biomaterial for various biomedical applications.
Acknowledgements
BBM greatly acknowledges funding support from Department of Biotechnology (DBT), Department of Science and Technology (DST) and Indian Council of Medical Research (ICMR), Government of India. Central Instrumentation Facility (CIF) at IIT Guwahati is highly acknowledged for use of high end equipments.
References
- R. Langer and J. Vacanti, Science, 1993, 260, 920–926 CAS.
- B. B. Mandal, S.-H. Park, E. S. Gil and D. L. Kaplan, Biomaterials, 2011, 32, 639–651 CrossRef CAS PubMed.
- H. Liu, H. Fan, Y. Wang, S. L. Toh and J. C. Goh, Biomaterials, 2008, 29, 662–674 CrossRef CAS PubMed.
- C. R. Wittmer, X. Hu, P.-C. Gauthier, S. Weisman, D. L. Kaplan and T. D. Sutherland, Acta Biomater., 2011, 7, 3789–3795 CrossRef CAS PubMed.
- Y. Wang, H. J. Kim, G. Vunjak-Novakovic and D. L. Kaplan, Biomaterials, 2006, 27, 6064–6082 CrossRef CAS PubMed.
- C. Vepari and D. L. Kaplan, Prog. Polym. Sci., 2007, 32, 991–1007 CrossRef CAS PubMed.
- G. H. Altman, F. Diaz, C. Jakuba, T. Calabro, R. L. Horan, J. Chen, H. Lu, J. Richmond and D. L. Kaplan, Biomaterials, 2003, 24, 401–416 CrossRef CAS PubMed.
- T. D. Sutherland, P. M. Campbell, S. Weisman, H. E. Trueman, A. Sriskantha, W. J. Wanjura and V. S. Haritos, Genome Res., 2006, 16, 1414–1421 CrossRef CAS PubMed.
- T. D. Sutherland, S. Weisman, H. E. Trueman, A. Sriskantha, J. W. Trueman and V. S. Haritos, Mol. Biol. Evol., 2007, 24, 2424–2432 CrossRef CAS PubMed.
- T. D. Sutherland, S. Weisman, A. A. Walker and S. T. Mudie, Biopolymers, 2012, 97, 446–454 CrossRef CAS PubMed.
- T. D. Sutherland, J. H. Young, S. Weisman, C. Y. Hayashi and D. J. Merritt, Annu. Rev. Entomol., 2010, 55, 171–188 CrossRef CAS PubMed.
- A. A. Walker, A. C. Warden, H. E. Trueman, S. Weisman and T. D. Sutherland, J. Mater. Chem. B, 2013, 1, 3644–3651 RSC.
- P. M. Campbell, H. E. Trueman, Q. Zhang, K. Kojima, T. Kameda and T. D. Sutherland, Insect Biochem. Mol. Biol., 2014, 48, 40–50 CrossRef CAS PubMed.
- F. Sehnal and T. Sutherland, Prion, 2008, 2, 145–153 CrossRef PubMed.
- J. Poole, J. S. Church, A. L. Woodhead, M. G. Huson, A. Sriskantha, I. L. Kyratzis and T. D. Sutherland, Macromol. Biosci., 2013, 13, 1321–1326 CrossRef CAS PubMed.
- S. Weisman, V. S. Haritos, J. S. Church, M. G. Huson, S. T. Mudie, A. J. Rodgers, G. J. Dumsday and T. D. Sutherland, Biomaterials, 2010, 31, 2695–2700 CrossRef CAS PubMed.
- T. D. Sutherland, J. S. Church, X. Hu, M. G. Huson, D. L. Kaplan and S. Weisman, PLoS One, 2011, 6, 16489 Search PubMed.
- J. Shi, S. Lua, N. Du, X. Liu and J. Song, Biomaterials, 2008, 29, 2820–2828 CrossRef CAS PubMed.
- X. Hu, Q. Lu, L. Sun, P. Cebe, X. Wang, X. Zhang and D. L. Kaplan, Biomacromolecules, 2010, 11, 3178–3188 CrossRef CAS PubMed.
- H. Hepburn and S. Kurstjens, Apidologie, 1988, 19, 25–36 CrossRef.
- K. Zhang, F. Si, H. Duan and J. Wang, Acta Biomater., 2010, 6, 2165–2171 CrossRef CAS PubMed.
- T. Kameda and Y. Tamada, Int. J. Biol. Macromol., 2009, 44, 64–69 CrossRef CAS PubMed.
- X. Hu, D. Kaplan and P. Cebe, Macromolecules, 2008, 41, 3939–3948 CrossRef CAS.
- B. B. Mandal and S. C. Kundu, Biomaterials, 2009, 30, 5019–5030 CrossRef CAS PubMed.
- B. B. Mandal and S. Kundu, Acta Biomater., 2009, 5, 2579–2590 CrossRef CAS PubMed.
- N. Bhardwaj and S. C. Kundu, Biomaterials, 2012, 33, 2848–2857 CrossRef CAS PubMed.
- N. Bhardwaj, Q. T. Nguyen, A. C. Chen, D. L. Kaplan, R. L. Sah and S. C. Kundu, Biomaterials, 2011, 32, 5773–5781 CrossRef CAS PubMed.
- B. B. Mandal, S. Kapoor and S. C. Kundu, Biomaterials, 2009, 30, 2826–2836 CrossRef CAS PubMed.
- M. F. Cutiongco, D. E. Anderson, M. T. Hinds and E. K. Yim, Acta Biomater., 2015, 25, 97–108 CrossRef CAS PubMed.
- J. Li, K. Zhang, P. Yang, W. Qin, G. Li, A. Zhao and N. Huang, Colloids Surf., B, 2013, 110, 199–207 CrossRef CAS PubMed.
- B. B. Mandal, E. S. Gil, B. Panilaitis and D. L. Kaplan, Macromol. Biosci., 2013, 13, 48–58 CrossRef CAS PubMed.
- C. K. Hashi, Y. Zhu, G.-Y. Yang, W. L. Young, B. S. Hsiao, K. Wang, B. Chu and S. Li, Proc. Natl. Acad. Sci. U. S. A., 2007, 104, 11915–11920 CrossRef CAS PubMed.
- L. Bacakova, E. Filova, M. Parizek, T. Ruml and V. Svorcik, Biotechnol. Adv., 2011, 29, 739–767 CrossRef CAS PubMed.
- U. J. Kim, J. Park, C. Li, H.-J. Jin, R. Valluzzi and D. L. Kaplan, Biomacromolecules, 2004, 5, 786–792 CrossRef CAS PubMed.
- Y. Cao and B. Wang, Int. J. Mol. Sci., 2009, 10, 1514–1524 CrossRef CAS PubMed.
- B. Panilaitis, G. H. Altman, J. Chen, H.-J. Jin, V. Karageorgiou and D. L. Kaplan, Biomaterials, 2003, 24, 3079–3085 CrossRef CAS PubMed.
- L. Meinel, S. Hofmann, V. Karageorgiou, C. Kirker-Head, J. McCool, G. Gronowicz, L. Zichner, R. Langer, G. Vunjak-Novakovic and D. L. Kaplan, Biomaterials, 2005, 26, 147–155 CrossRef CAS PubMed.
- X. You and J. J. Pak, Sens. Actuators, B, 2014, 202, 1357–1365 CrossRef CAS.
Footnote |
† Authors contributed equally. |
|
This journal is © The Royal Society of Chemistry 2016 |
Click here to see how this site uses Cookies. View our privacy policy here.