DOI:
10.1039/C6RA13075E
(Paper)
RSC Adv., 2016,
6, 85254-85260
π-Stacking assisted redox active peptide–gallol conjugate: synthesis of a new generation of low-toxicity antimicrobial silver nanoparticles†
Received
19th May 2016
, Accepted 27th August 2016
First published on 1st September 2016
Abstract
In this study, we describe the rational design and synthesis of a redox-active petide–gallol conjugate and explore its application in the preparation of antimicrobial silver nanoparticles. Increase in acidity and redox activity of peptide–gallol compounds upon pi-stacking was predicted in silico. A representative phenyl alanine–glycine dipeptide–gallol conjugate was synthesized via click chemistry between N-propargyl dipeptide and azidomethyl pyrogallol. The enhanced redox properties of the conjugate were exemplified by rapid formation of silver nanoparticles (Ag NPs) at room temperature without the need of an external capping agent. The nanoparticles formed were found to exhibit prominent antifungal activity against Candida albicans NCIM 3471 along with robust biofilm eradication ability. Thus, our study established the hypothesis that rationally designed peptide–gallol conjugates can be used as biocompatible, redox-active moieties for in situ generation of non-toxic antimicrobial silver nanoparticles in a fast one pot chemical reduction method.
Introduction
The unique antimicrobial properties of silver nanoparticles (Ag NPs) make them an integral component for water and air purifying agents, food preservatives, cosmetics, clothing and numerous household items.1 Reactive oxygen species (ROS) have been identified as the key mediators for this silver-induced cytotoxicity.2 The cytotoxic potential has an intimate relation with nanoparticle size; typically, the smaller the size of the nanoparticles, the higher is the cytotoxic efficiency.3 Ag NPs had been demonstrated to exert a size-dependant apoptotic effect in osteoblast precursor cell lines in vitro, with 10 nm particles having a higher apoptotic effect over 50 nm and 100 nm particles.4 A recent study reaffirmed superior toxicity of 10 nm Ag NPs against a range of microbial organisms and mammalian cells in vitro and further demonstrated higher intracellular bioavailability of 10 nm Ag NPs.5 The antifungal activity of the Ag NPs is well established and reported to be comparable or often better than conventional antifungal agents like amphotericin B.6 One specific study determined a similar size dependency in fungicidal property of Ag NPs where particles in the range of 20–25 nm were most efficacious against fungal strains like C. albicans and C. neoformans.7 Consequently, control over size is an important consideration in the design and synthesis of Ag NPs-based antimicrobial agent. Reduction mediated methods afford efficient size control through optimization of nucleation and subsequent Ostwald ripening processes.8 Agnihotri et al.9 designed a co-reduction based approach to synthesize monodisperse Ag NPs with mean size ranging from 5 to 100 nm. The synthesized particles were studied for size specific toxicity and Ag NPs in the range of 5–10 nm were demonstrated to have the highest bactericidal potential.
Over the years, various synthetic routes, reducing agents and stabilizers have been used to tune size, distribution and shape of Ag NPs.10,11 Two methods are primarily used for synthesis: a physical approach that uses techniques like evaporation, condensation or laser ablation and a chemical route which involves reduction of metal ions to metallic atom followed by agglomeration to nano-clusters.12,13 It is possible to control the shape and size of nanoparticles by varying molar ratios of substrate and capping agent.14 Size selectivity may also be attained by sorting nanoparticles in centrifugal field.15 So far, Ag NPs have been used as a topical antibacterial agent for wound healing but the application is toxicity-limited. Recently, application of green chemistry perspectives in synthesis of Ag NPs has received substantial attention because of concerns being raised on their biological and environmental impact.16–19 Natural products like crude green tea extract or catechin has been exploited previously for the synthesis of biocompatible Ag NPs.20 They exhibited low toxicity towards mammalian cells even at high concentrations, due to the presence of antioxidant polyphenols on their surface which inhibits metal-induced ROS inside the cell. Gallic acid [3,4,5-trihydroxybenzoic acid] is a well-known phenolic compound found in tea leaves and has been reported to reduce metallic silver salts to Ag NP at high temperatures.21 However, due to application of harsher reaction conditions, this method is neither clean nor energy-efficient.
The present study reports the rational development of an engineered polyphenol–peptide conjugate that can afford a biocompatible route to in situ generation of stable Ag NPs exploiting a fast, one pot addition method. The basis of our design involved combining four building blocks namely the gallol unit (2), propargyl amine (3), glycine (4) and L-phenyl alanine (5) through a triazole linker into a final conjugate molecule 1 (Scheme 1).
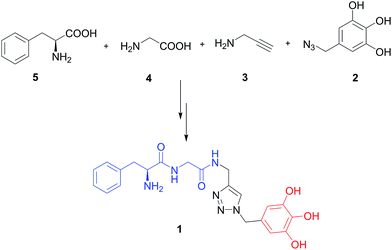 |
| Scheme 1 Building blocks of peptide–gallol conjugate. | |
The gallol moiety was chosen to serve as the redox active partner; phenyl alanine was chosen as for its stacking ability due to the presence of aromatic moiety, glycine and triazole were included to provide enough flexibility to the molecule. We hypothesized that the final conjugate molecule (1) comprising these four units would present sufficient redox activity to reduce silver salt precursors to stable and biocompatible metallic silver nanoparticles under ambient conditions.22 As ionization of phenolic hydroxyl groups are critical to enhance the redox activity of polyphenolic compounds, we reasoned that greater ionization of phenolic groups will enhance the reducing property while affording milder conditions for generation of Ag NPs. The reason for such expectation was driven by a possible decrease in pKa with stacking interactions involving phenyl alanine side chain and the phenolic moiety. We did computations to understand the effect of π–π interactions on the acidity of peptide–gallol conjugate and determined that the aforesaid interaction might increase acidity. Our calculations were in suitable agreement with previous reports where stacking interactions were hypothesized to play a pivotal role in controlling the acidity of phenolic compounds and a decrease in pKa of the phenol up to 2 units was predicted theoretically, and was validated with experimental findings.23,24 The enhanced redox property of 1 was corroborated by rapid formation of silver nanoparticles (AgNPs) at room temperature without recruiting any external capping agent. Encouragingly, these nanoparticle formulations also showed good antimicrobial activity in vitro with decent minimum inhibitory concentration (MIC) values, particularly against Candida albicans. In addition, these nanoparticles also inhibited the biofilm formation. It may be interesting to note that polar aspartate and tyrosine based short peptide based hydrogels has been previously exploited to synthesize gold and silver nanoparticles. The carboxylate moiety of the tyrosine or the aspartate residues facilitated reduction under visible light.25,26
Experimental
Materials and methods
All reactions were conducted with oven-dried glassware under inert atmosphere unless mentioned otherwise. All common reagents were of commercial grade unless otherwise specified. All reactions were conducted with anhydrous solvents dried using standard methods and purified by distillation prior to use. Silica gel (60–120 and 230–400 mesh) was used for column chromatography. Thin layer chromatography (TLC) was performed on aluminium-backed plates coated with Silica gel 60. Locally available Ultra violet light chamber and iodine blower were used as the TLC spot indicator. All intermediates and final products were characterized using proton (1H) nuclear magnetic resonance (NMR), 13C NMR and mass spectroscopy. The NMR spectra were recorded using Bruker 400 MHz and 600 MHz spectrometer. The following abbreviations have been used for NMR peak assignments: s = singlet, d = doublet, t = triplet, q = quartet, m = multiplet, dd = double of doublet. Electrospray-Ionization Mass Spectrometer manufactured by Waters Corporation (Milford, Massachusetts, USA) was used to record mass spectra.
Computational methods for geometry optimization
Geometry optimizations were carried out with Orca 3.0.3 software package.27 A phenyl alanine dipeptide–gallol conjugate was compared against a control peptide–gallol conjugate where the π–π interactions were eliminated by having an alanine instead of phenyl alanine in one of the terminus. As the strength of an acid depends upon the stability of the corresponding conjugate base, we decided to compute difference in the thermodynamic energy of the peptides and the respective conjugate bases. Thus, the geometry optimizations were done for the peptide–gallol conjugates and their conjugate bases (detailed procedure are described in ESI section†).
Synthesis of peptide–gallol conjugate
The key step in the synthesis of peptide gallol conjugate involved mediating the ‘click chemistry’28 between azidomethylgallol and the protected phenyl-N-propargyl glycine. The gallol unit was prepared by hydrolyzing tetrabenzylgallate and then reducing to the corresponding alcohol. The latter was subsequently converted to the azide analogue via the bromide (Scheme 2). The protected benzyl ester was made by 1-ethyl-3-(3-dimethylaminopropyl)carbodiimide (EDC) mediated coupling of Boc-phenyl alanine with benzyl glycinate. Hydrolysis followed by another coupling with propargyl amine provided the dipeptide component. Click reaction between the azidomethylgallol and the dipeptide followed by deprotection (first with trifluoroacetic acid and then with H2 in presence of Pearlman catalyst) afforded the final peptide–gallol conjugate 1 (Scheme 3). This was characterized by 1H NMR (600 MHz), 13C NMR (150 MHz) and mass spectrometry (spectral data provided in ESI†).
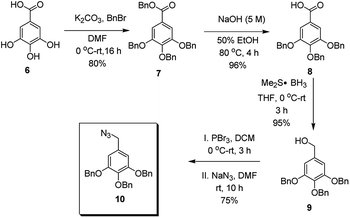 |
| Scheme 2 Schematic representation of the synthesis of polyhydroxyl phenolic azide 10. | |
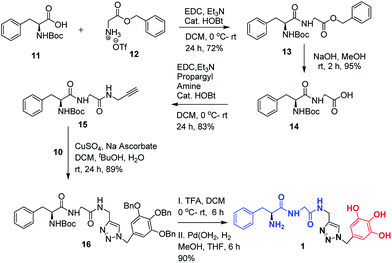 |
| Scheme 3 Schematic representation of the synthesis of Phe–Gly–gallol 1. | |
Synthesis of Ag NPs
The Ag NPs were prepared by mixing an aqueous solution of AgNO3 with a methanolic solution of the conjugate 1 at room temperature. Ag NPs formed almost instantaneously, with a characteristic straw-yellow coloration. Briefly, to a colorless stirring solution of AgNO3 (0.5 mL, 10 mM) in deionized water, different volumes of Phe–Gly–gallol 1 in dry MeOH (0.8 mg mL−1) was added at room temperature (r.t.), maintaining molar ratio of 1
:
2 (AgNP1), 1
:
4 (AgNP2) and 1
:
8 (AgNP3) respectively between reducing agent 1 and AgNO3 precursor. Appropriate volume of MeOH was added prior to the addition of 1 to maintain the net volume of the reaction mixture at 5 mL. Stirring was continued for 15 min. Suspensions of AgNP1, AgNP2 and AgNP3 was stored at 4 °C without further purification.
Physicochemical characterization
Absorption spectra of Ag NPs at r.t. were recorded using a Shimadzu (model no. UV2450) Ultra violet-visible (UV-Vis) spectrophotometer over a period of 24 h at different time intervals. Jeol JEM – 2100 High Resolution Transmission Electron Microscope (HR-TEM) was used to determine particle size. The final particles were drop-casted onto a carbon-coated copper grid and viewed at 200 kV. The diameters of particles were calculated using ImageJ (1.48v).
Antimicrobial studies
Minimum inhibitory concentration (MIC) values of nanoparticles against Staphylococcus epidermidis (NCIM 2493) and Escherichia coli (ATCC® 35218™) were determined according to guidelines of Clinical and Laboratory Standards Institute (CLSI) Performance standards for antimicrobial susceptibility testing; seventeenth informational supplement Document M100-S17. CLSI, Wayne, PA: CLSI (2007). The culture conditions and bacterial growth were monitored as described earlier by Roymahapatra et al.29 For Candida albicans 3471, growth and culture conditions were monitored at 30 °C in RPMI or potato dextrose agar medium. Microbial growth was visually inspected after overnight incubation. The lowest concentration of nanoparticles inhibiting the growth reflected the MICs.
Biofilm-eradication and biocompatibility analysis
The formation of E. coli and C. albicans biofilm on polystyrene plate was carried out according to Samanta et al.30 To determine the effect of AgNP1 on biofilm eradication from the polystyrene plate, nanoparticles were added to the biofilm in different concentrations. After incubation, the remaining biofilm metabolic activity was quantified by the XTT-reduction assay. For imaging, the experimental plates were flipped and stained biofilms were visualized by fluorescence microscopy to compare their gross morphologies. MTT [(3-(4,5-dimethylthiazol-2-yl)-5-(3-carboxymethoxyphenyl)-2-(4-sulfophenyl)-2H-tetrazolium)], assay was performed to determine the cell cytotoxicity of the phe–gly–gallol peptide capped AgNP1 against mouse embryonic fibroblast cell line, 3T3 following the method described earlier by Mandal et al.31
Results and discussion
Geometry optimizations predict increased acidity with π–π interactions
We performed computational calculations to determine the effect of stacking interactions on acidity. It was observed that the conjugate base of the peptide–gallol having phenyl terminal is substantially more stable (4.47 kcal mol−1) than alanine terminated peptide–gallol with no functional ‘handle’ to undergo π–π interaction. The computed energy values and the energy minimized conformation of the peptide–gallol hybrids and the conjugate bases are shown in Table 1. The stability of the conjugate base of phenyl terminal peptide–gallol is due to strong π–π interactions as indicated by its energy minimized conformation, thereby increasing the acidity.
Table 1 The free energy of the peptide–gallol and its conjugate base in most stable geometry. The free energies are given in hartree and the energy differences are in kcal mol−1
Entry |
System |
Free energy of acid (hartree) |
Free energy of conjugate base (hartree) |
Free energy difference (kcal mol−1) |
1 |
Peptide–gallol with phenyl alanine termini |
 |
 |
323.73 |
2 |
Peptide–gallol with alanine termini |
 |
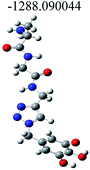 |
319.26 |
Based on computational predictions, we determined that incorporation of a phenyl alanine moiety on the gallol backbone can enhance the redox potential of the target construct. In line with that rationale, we synthesized a peptide–gallol conjugate and confirmed its structure with extensive spectrochemical analysis. The redox-activity of this conjugate was used to synthesize antimicrobial Ag Nps from metallic Ag at room temperature using a simple, one-pot reaction.
Physicochemical characterization of Ag NPs
Absorption spectra of spherical Ag NPs are reported to present a maximum between 420 and 450 nm with a blue or red shift with corresponding decrease or increase in particle size.32 The UV-Vis spectra of Ag NPs, presented in Fig. 1a–c demonstrated surface plasmon resonance (SPR) characteristic to Ag NPs.33 AgNP1 presented a moderately narrow band with a peak maxima at 422 nm [Fig. 1a], AgNP2 also displayed the characteristic Ag-SPR band at around 422 nm [Fig. 1b]; however, the intensity of the primary peak was relatively weak compared to AgNP1. AgNP3s presented a wide band with further decrease in primary peak height [Fig. 1c]. Absorption spectra were recorded over different time intervals to determine the stability of the particles. At r.t., the intensity of the characteristic bands for AgNPs were observed to decrease over time due to depletion of stable nanoparticles from suspension. However, the AgNPs were stable for weeks when maintained at 4 °C.
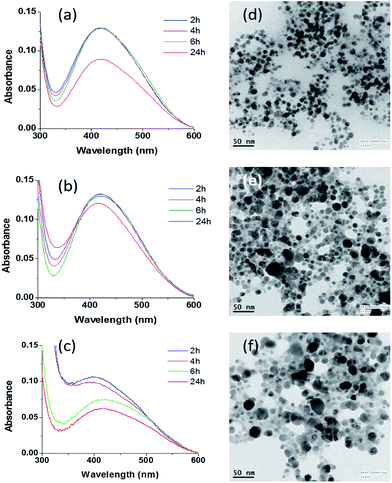 |
| Fig. 1 (a–c) UV-Vis absorption spectra of AgNP1, AgNP2 and AgNP3 respectively; (d–f) TEM images of AgNP1, AgNP2 and AgNP3 respectively. | |
The TEM images of Ag NPs are presented in Fig. 1d–f against the corresponding UV spectrum. Nanoparticles showed spherical to pseudo-spherical morphology. AgNP1 [Fig. 1d], AgNP2 [Fig. 1e] and AgNP3 [Fig. 1f] presented particles with mean diameter of 9.584 ± 5.823 nm, 12.034 ± 6.216 nm and 14.512 ± 7.223 nm respectively. These results indicate that increase of reductant concentration didn't have a statistically significant impact on mean diameter of particles. However, with decreasing reductant concentration, the shape of the particles declined from spherical to pseudo-spherical morphology with broader size distribution.
Antimicrobial results
Table 2 presents the MIC values obtained for the Ag NPs tested against Staphylococcus epidermidis (NCIM 2493), Escherichia coli (ATCC® 35218™) and Candida albicans 3471. As observed, all the three nanoformulations exhibited prominent antibacterial and antifungal activity. We couldn't however find a difference in the MIC values. This is in agreement with our TEM analysis. As antimicrobial activity of Ag NPs depends critically on their size,33 lack of statistically significant difference in mean particle diameter explains nano-formulations reported herein presenting similar MIC values. The MIC value was lower when tested against E. coli (Gram-negative) than against S. epidermidis (Gram-positive). Differences on the cellular wall of each strain can be used to explain the results; Gram-positive strains have a wider cellular wall than Gram-negative strains.34 All Ag NPs presented high antifungal activity and our results are in agreement with previous reports.6 It was also interesting to note that peptide–gallol conjugate alone didn't exert any antibacterial and antifungal activity, which suggests that the pristine conjugate is nontoxic and can effectively serve as a biocompatible coat for Ag NPs.
Table 2 Minimum inhibition concentration of AgNPs (μg mL−1)
Formulation |
E. coli |
S. epidermidis |
C. albicans |
No antimicrobial activity was found with the concentrations used in this work. The MIC values are averages of 3 experiments. |
AgNP1 |
9.37 |
4.68 |
0.29 |
AgNP2 |
9.37 |
4.68 |
0.29 |
AgNP3 |
9.37 |
4.68 |
0.29 |
Peptide–gallol conjugate |
a |
a |
a |
Biofilm-eradication and biocompatibility analysis
As no significant difference was detected between the antimicrobial activities of the Ag NPs synthesized under different reaction conditions, we proceeded for further analysis with AgNP1. It was observed that the biofilm adhered cells were metabolically inactive and mostly dead after treatment with AgNP1 (Fig. 2) at previously determined MIC values (Table 2).
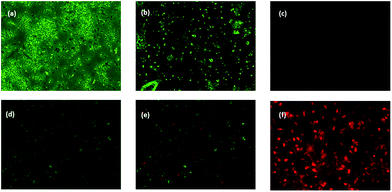 |
| Fig. 2 Images of biofilm inhibition using the synthesized nanoparticles. Images were captured after 24 h treatment with AgNP1. The biofilm eradication of AgNP1 was tested against bacterial pathogen, E. coli (a–c) and fungal pathogen, C. albicans (d–f). Biofilm was developed after 24 h growth in polystyrene plate and subsequently treated with AgNP1 with different concentrations as 0 μg mL−1 (a & d); 4.68 μg mL−1 (b); 9.37 μg mL−1 (c); 0.15 μg mL−1 (e) and 0.29 μg mL−1 (f). AgNP1 significantly reduced live cells (green fluorescence) in developed biofilm and cells were dead (red fluorescence) against all tested pathogens. Images were captured at a magnification of 400×. | |
We next analyzed the toxicity of our Ag NPs on mammalian cells in vitro and compared against particles synthesized using NaBH4 as substrate. The aim of the experiment was to determine if the Ag NPs as synthesized can be exploited as antimicrobial agents and how they compare against Ag NPs synthesized using conventional routes.
The result obtained from the cytotoxicity assay performed on non-carcinoma 3T3 cell line revealed that the level of toxicity was almost two fold reduced for the presently formulated nanoparticle in comparison to NaBH4 synthesized nanoparticles (Fig. 3). Cell viability was decreased in dose dependent manner with increasing the concentration of nanoparticles. The obtained IC50 values were 250 μg mL−1 and 100 μg mL−1 for phe–gly–gallol peptide capped AgNP1 and NaBH4 mediated synthesized nanoparticles, respectively. The observed IC50 value of 250 μg mL−1 for phe–gly–gallol peptide capped AgNP1, was substantially higher than IC50 of 0.5 μg mL−1 to control Candida infection, thus providing a reasonable therapeutic window.
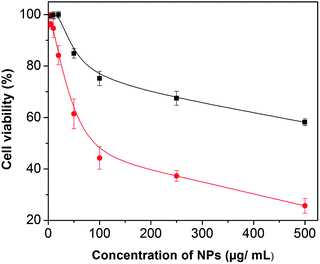 |
| Fig. 3 Dose dependent cytotoxicity assay. Cell viability was monitored in a range of nanoparticles dose (1–500 μg mL−1) against non-carcinoma 3T3 cell line. The chemically (NaBH4) synthesized Ag-nanoparticle shows more toxicity (red line) than phe–gly–gallol peptide mediated Ag NPs (black line). | |
Silver nanoparticles are well known to produce huge amount of reactive oxygen species (ROS) responsible for cell toxicity. In this case, the reducing or capping agent, phe–gly–gallol moiety may have some free phenolic OH. It is possible to neutralize the species like singlet oxygen, hydrogen peroxide, and peroxynitrite by the donation of hydrogen radical from free phenolic OH in gallol moiety which might be the cause of less toxicity.
Conclusion
For the first time, we used a rationally designed peptide–gallol conjugate to synthesize Ag NPs in the size range 10–20 nm using a fast, one pot chemical reduction method. Synthesized Ag NPs were characterized using TEM and UV-Vis spectroscopy. The antimicrobial activity of the Ag NPs were analyzed and was found to be comparable with reported literature.6 Our present study established the proof-of-concept that peptide–gallol conjugates can be used as biocompatible, redox-active moieties for in situ generation of antimicrobial Ag NPs. Our future studies will be directed towards tailoring the redox-activity of such polyhydroxyphenol–peptide conjugate. We plan to extend this study by synthesizing new, molecularly tailored peptide–gallol conjugates that can provide better control over size, shape and PDI of Ag NPs while augmenting their antimicrobial efficiency.
Spectral characterization35
(2-tert-Butoxycarbonylamino-3-phenyl propionylamino)-acetic acid benzyl ester (13)
δH (400 MHz, CDCl3) 7.40–7.16 (m, 10H), 6.48 (t, J = 5.1 Hz, 1H), 5.16 (s, 2H), 5.01 (s, 1H), 4.41 (s, 1H), 4.08 (dd, J = 18.3, 5.5 Hz, 1H), 3.97 (dd, J = 18.3, 5.1 Hz, 1H), 3.12 (dd, J = 13.9, 6.5 Hz, 1H), 3.03 (dd, J = 13.8, 7.3 Hz, 1H), 1.39 (s, 9H); δC (100 MHz, CDCl3) 171.7, 169.5, 155.6, 136.7, 135.2, 129.4, 128.8, 128.7, 128.5, 127.1, 80.4, 67.3, 55.8, 41.5, 38.5, 28.4; ESI-MS m/z calcd for C23H28N2NaO5 [M + Na]+ 435.1896, found 435.1904.
(2-tert-Butoxycarbonylamino-3-phenyl-propionylamino)-acetic acid (14)
δH (400 MHz, DMSO-d6) 12.60 (s, 1H), 8.32–8.16 (m, 1H), 7.47–7.10 (m, 5H), 6.92 (d, J = 8.7 Hz, 1H), 4.20 (td, J = 10.7, 9.9, 3.7 Hz, 1H), 3.79 (dd, J = 16.0, 5.8 Hz, 2H), 3.00 (dd, J = 14.0, 3.9 Hz, 1H), 2.71 (dd, J = 13.9, 10.7 Hz, 1H), 1.28 (s, 8H); δC (150 MHz, DMSO) 172.1, 171.2, 155.2, 138.3, 129.2, 127.9, 126.1, 77.9, 55.5, 40.7, 37.5, 28.1; HRMS: m/z calcd for C16H22N2NaO5 [M + Na]+ 345.1426, found 345.1434.
[2-Phenyl-1-(prop-2-ynylcarbamoylmethyl-carbamoyl)-ethyl]-carbamic acid tert-butyl ester (15)
δH (400 MHz, CDCl3) 7.36–7.15 (m, 5H), 6.89 (s, 1H), 6.67 (s, 1H), 5.09 (d, J = 6.7 Hz, 1H), 4.29 (q, J = 6.9 Hz, 1H), 3.92–4.06 (m, 3H), 3.85 (dd, J = 16.8, 5.7 Hz, 1H), 3.12 (dd, J = 13.8, 6.6 Hz, 1H), 3.01 (dd, J = 13.8, 7.6 Hz, 1H), 2.19 (s, 1H), 1.41 (s, 9H); δC (100 MHz, CDCl3) 172.2, 168.6, 156.0, 136.4, 129.5, 129.3, 128.9, 128.8, 127.4, 81.0, 79.4, 71.6, 56.7, 43.2, 38.0, 29.2, 28.4; HRMS: m/z calcd for C19H25N3NaO4 [M + Na]+ 382.1743, found 382.1736.
{2-Phenyl-1-[({[1-(3,4,5-tris-benzyloxy-benzyl)-1H-[1,2,3]triazol-4-ylmethyl]-carbamoyl}-methyl)carbamoyl]-ethyl}-carbamic acid tert-butyl ester (16)
δH (400 MHz, CDCl3) 7.40–6.99 (m, 22H), 6.70 (s, 1H), 6.49 (s, 2H), 5.24 (s, 2H), 5.03 (s, 1H), 4.99 (s, 4H), 4.95 (s, 2H), 4.42–4.29 (m, 2H), 4.19 (q, J = 7.0 Hz, 1H), 3.86 (dd, J = 16.8, 6.1 Hz, 1H), 3.73 (dd, J = 16.9, 5.6 Hz, 1H), 2.98 (dd, J = 14.3, 6.9 Hz, 1H), 2.87 (dd, J = 13.8, 7.6 Hz, 1H), 1.26 (s, 9H); δC (100 MHz, CDCl3) 172.2, 169.1, 155.9, 153.3, 145.2, 144.5, 138.9, 137.7, 136.8, 136.4, 129.9, 129.3, 128.9, 128.7, 128.3, 128.1, 128.0, 127.6, 127.3, 122.3, 108.1, 80.9, 75.3, 71.4, 56.5, 54.4, 43.2, 38.0, 35.1, 28.3; HRMS: m/z calcd for C47H61N6O7 [M + H]+ 811.3819, found 811.3811.
2-Amino-3-phenyl-N-({[1-(3,4,5-trihydroxy-benzyl)-1H-[1,2,3]triazol-4-ylmethyl]-carbamoyl}-methyl)-propionamide (1)
δH (600 MHz, DMSO-d6) 8.27 (t, J = 5.8 Hz, 1H), 8.19 (s, 1H), 7.82 (s, 1H), 7.29–7.17 (m, 5H), 6.24 (s, 2H), 5.26 (s, 2H), 4.29 (d, J = 5.6 Hz, 2H), 3.70 (s, 2H), 3.46 (dd, J = 8.7, 4.6 Hz, 1H), 2.98 (dd, J = 13.5, 4.6 Hz, 1H), 2.60 (dd, J = 13.5, 8.8 Hz, 1H); δC (150 MHz, DMSO) 170.3, 168.6, 146.1, 144.8, 138.5, 132.9, 129.3, 128.2, 126.2, 125.9, 122.6, 107.0, 55.9, 52.9, 41.9, 40.4, 34.2; HRMS: m/z calcd for C21H25N6O5 [M + H]+ 441.1886, found 441.1904.
Acknowledgements
DST is acknowledged for an SERB grant (No. SB/S1/OC-94/2013) and for the JC Bose Fellowship to AB which supported this research. KS and PB is also grateful to DST, Government of India, for a young scientist fellowship. PB thanks CSIR, Government of India, for a research fellowship.
Notes and references
- C. Marambio-Jones and E. M. V. Hoek, J. Nanopart. Res., 2010, 12, 1531–1551 CrossRef CAS.
- C. Carlson, S. M. Hussain, A. M. Schrand, L. K. Braydich-Stolle, K. L. Hess, R. L. Jones and J. J. Schlager, J. Phys. Chem. B, 2008, 112, 13608–13619 CrossRef CAS PubMed.
- Z. Lu, K. Rong, J. Li, H. Yang and R. Chen, J. Mater. Sci.: Mater. Med., 2013, 24, 1465–1471 CrossRef CAS PubMed.
- T. H. Kim, M. Kim, H. S. Park, U. S. Shin, M. S. Gong and H. W. Kim, J. Biomed. Mater. Res., Part A, 2012, 100, 1033–1043 CrossRef PubMed.
- A. Ivask, I. Kurvet, K. Kasemets, I. Blinova, V. Aruoja, S. Suppi, H. Vija, A. Kakinen, T. Titma, M. Heinlaan, M. Visnapuu, D. Koller, V. Kisand and A. Kahru, PLoS One, 2014, 9, e102108 Search PubMed.
- A. Panáček, M. Kolář, R. Večeřová, R. Prucek, J. Soukupová, V. Kryštof, P. Hamal, R. Zbořil and L. Kvítek, Biomaterials, 2009, 30, 6333–6340 CrossRef PubMed.
- F. Martinez-Gutierrez, P. L. Olive, A. Banuelos, E. Orrantia, N. Nino, E. M. Sanchez, F. Ruiz, H. Bach and Y. Av-Gay, Nanomedicine: Nanotechnology, Biology and Medicine, 2010, 6, 681–688 CrossRef CAS PubMed.
- A. Panáček, R. Prucek, J. Hrbáč, T. j. Nevečná, J. Šteffková, R. Zbořil and L. Kvítek, Chem. Mater., 2014, 26, 1332–1339 CrossRef.
- S. Agnihotri, S. Mukherji and S. Mukherji, RSC Adv., 2014, 4, 3974–3983 RSC.
- K. M. M. Abou El-Nour, A. a. Eftaiha, A. Al-Warthan and R. A. A. Ammar, Arabian J. Chem., 2010, 3, 135–140 CrossRef CAS.
- S. Y. Yeo, H. J. Lee and S. H. Jeong, J. Mater. Sci., 2003, 38, 2143–2147 CrossRef CAS.
- A. S. Gurav, T. T. Kodas, L.-M. Wang, E. I. Kauppinen and J. Joutsensaari, Chem. Phys. Lett., 1994, 218, 304–308 CrossRef CAS.
- S. Kapoor, D. Lawless, P. Kennepohl, D. Meisel and N. Serpone, Langmuir, 1994, 10, 3018–3022 CrossRef CAS.
- Y. Sun and Y. Xia, Science, 2002, 298, 2176–2179 CrossRef CAS PubMed.
- F. Bonaccorso, M. Zerbetto, A. C. Ferrari and V. Amendola, J. Phys. Chem. C, 2013, 117, 13217–13229 CAS.
- P. Anastas and N. Eghbali, Chem. Soc. Rev., 2010, 39, 301–312 RSC.
- J. M. DeSimone, Science, 2002, 297, 799–803 CrossRef CAS PubMed.
- M. Poliakoff and P. Anastas, Nature, 2001, 413, 257 CrossRef CAS PubMed.
- V. K. Sharma, R. A. Yngard and Y. Lin, Adv. Colloid Interface Sci., 2009, 145, 83–96 CrossRef CAS PubMed.
- Q. Sun, X. Cai, J. Li, M. Zheng, Z. Chen and C.-P. Yu, Colloids Surf., A, 2014, 444, 226–231 CrossRef CAS.
- D. Li, Z. Liu, Y. Yuan, Y. Liu and F. Niu, Process Biochem., 2015, 50, 357–366 CrossRef CAS.
- G. A. Martínez-Castañón, N. Niño-Martínez, F. Martínez-Gutierrez, J. R. Martínez-Mendoza and F. Ruiz, J. Nanopart. Res., 2008, 10, 1343–1348 CrossRef.
- C. Ibarra, B. S. Nieslanik and W. M. Atkins, Biochemistry, 2001, 40, 10614–10624 CrossRef CAS PubMed.
- A. Olasz, P. Mignon, F. De Proft, T. Veszprémi and P. Geerlings, Chem. Phys. Lett., 2005, 407, 504–509 CrossRef CAS.
- S. Ray, A. K. Das and A. Banerjee, Chem. Commun., 2006, 2816–2818 RSC.
- B. Adhikari and A. Banerjee, Chem.–Eur J., 2010, 16, 13698–13705 CrossRef CAS PubMed.
- F. Neese, Orca 3.0.3, Max Planck Institute for Bioinorganic Chemistry: Mülheim/Ruhr, Germany, https://orcaforum.cec.mpg.de Search PubMed.
- V. V. Rostovtsev, L. G. Green, V. V. Fokin and K. B. Sharpless, Angew. Chem., Int. Ed., 2002, 41, 2596–2599 CrossRef CAS.
- G. Roymahapatra, S. M. Mandal, W. F. Porto, T. Samanta, S. Giri, J. Dinda, O. L. Franco and P. K. Chattaraj, Curr. Med. Chem., 2012, 19, 4184–4193 CrossRef CAS PubMed.
- T. Samanta, G. Roymahapatra, W. F. Porto, S. Seth, S. Ghorai, S. Saha, J. Sengupta, O. L. Franco, J. Dinda and S. M. Mandal, PLoS One, 2013, 8, e58346 CAS.
- S. M. Mandal, L. Migliolo, S. Das, M. Mandal, O. L. Franco and T. K. Hazra, J. Cell. Biochem., 2012, 113, 184–193 CrossRef CAS PubMed.
- S. Pal, Y. K. Tak and J. M. Song, Appl. Environ. Microbiol., 2007, 73, 1712–1720 CrossRef CAS PubMed.
- R. K. Bera, S. M. Mandal and C. R. Raj, Lett. Appl. Microbiol., 2014, 58, 520–526 CrossRef CAS PubMed.
- J. Thiel, L. Pakstis, S. Buzby, M. Raffi, C. Ni, D. J. Pochan and S. I. Shah, Small, 2007, 3, 799–803 CrossRef CAS PubMed.
- Limited spectral data.
Footnotes |
† Electronic supplementary information (ESI) available: The procedure for preparation of unknown compounds, their 1H and 13C NMR and HRMS spectra, Cartesian coordinates. See DOI: 10.1039/c6ra13075e |
‡ Equal contributions. |
|
This journal is © The Royal Society of Chemistry 2016 |
Click here to see how this site uses Cookies. View our privacy policy here.