DOI:
10.1039/C6RA13461K
(Paper)
RSC Adv., 2016,
6, 73817-73826
Dendronized PLGA nanoparticles with anionic carbosilane dendrons as antiviral agents against HIV infection†
Received
24th May 2016
, Accepted 22nd July 2016
First published on 28th July 2016
Abstract
The development of novel strategies to prevent HIV-1 infection is of outstanding relevance. PLGA nanoparticles have become some of the most used nanosized materials for biomedical applications. Polyanionic carbosilane dendrimers and dendrons have shown potent and broad-spectrum anti-HIV-1 activity. Therefore, PLGA nanoparticles functionalized with carbosilane anionic dendrons through a carbodiimide-mediated reaction have been prepared with the aim of them becoming novel antiviral agents against HIV infection. The biocompatibility of the new dendronized nanoparticles has been explored in PBMC and HEC-1A cells. Both inhibition experiments showed differences between dendronized nanoparticles, free dendrons and PLGA nanoparticles. The results obtained with the dendronized nanoparticles indicate that these systems are powerful anti-HIV agents, compared to dendrons or dendrimers alone or PLGA NPs, suggesting an enhancement of their interaction with viral or cell receptors. The topology and size of these new systems could be responsible for the higher activity observed.
Introduction
Polymeric nanoparticles, also known as ‘soft’ nanoparticles, have attracted great interest in the past few years due to the advances produced in controlled polymerization that have enabled the engineering of more sophisticated and multifunctionalized nanosized compounds, to be used in nanomedicine.1 Currently, they are being used in the diagnosis and treatment of a great variety of diseases, from cancer to viral infections, mainly as carriers with polymeric scaffolds that can be chemically modified to modulate the package and delivery of their cargoes.2,3
Different polymers have been used in the preparation of polymeric nanoparticles, like PEG, poly(N-vinylpyrrolidone) or poly(N-isopropylacrylamide) to mention some of the most common ones. However, we focused on poly(latic-co-glycolic acid), PLGA, which is able to generate polymeric nanoparticles4 due to its interesting properties: (i) PLGA is a biocompatible and biodegradable polymer producing lactic and glycolic acid, which can be eliminated by its inclusion in the Krebs cycle of the cells; (ii) PLGA has been approved for its use for parenteral administration both by the FDA and the European Medicine Agency; (iii) there are well described methods for its production and formulation and; (iv) PLGA nanoparticles can protect drugs from degradation while providing a sustained release; and (v) its surface can be modified to present better interactions with biological systems or to be targeted to specific tissues.5 These characteristics have made PLGA nanoparticles excellent candidates for their application as controlled release systems for drugs,6–8 widely explored both with modified9,10 and non-modified nanoparticles,11,12 and as promoters for tissue regeneration.13,14 Several examples can be found in the literature where modified PLGA nanoparticles have been used as drug7,9,15 and gene16,17 delivery systems. PLGA nanoparticles properly functionalized have been also used to deliver active ingredients to specific targets, for instance to the central nervous system (CNS) by crossing the blood–brain barrier (BBB).18 However, no studies on the use of these systems as pharmaceutical drugs per se have been reported so far.
Our research group has been working in the past years in the preparation of polyanionic carbosilane dendritic nanocompounds as antiviral agents for HIV. Several examples have been published both for dendrimer synthesis and applications,19–22 and more recently for the preparation of dendritic wedges or dendrons, which present new and interesting opportunities for their coupling to other molecules of interest.23–25 Dendrons can be used to modify the surface of nanoparticles, in a process known as dendronization,26 which has been widely described for the modulation of properties on metallic nanoparticles27,28 or even for their stabilization.29 This information can be easily transferred for the modification of polymeric nanoparticles such as those based on PLGA.30,31 Recently, we have prepared polymeric nanoparticle dispersions obtained from nano-emulsion templating using a low-energy emulsification method followed by solvent evaporation without further washing steps and their multi-functionalization by using cationic carbosilane dendrons to generate dendronized PLGA nanoparticles. It is worth noting the importance of the nano-emulsion template strategy, since it defines the properties of the resulting nanoparticles.18,32
The applications of PLGA nanoparticles obtained from nano-emulsion templating in the biomedical field are wide. These systems conveniently dendronized with cationic groups are able, for example, to form complexes with phosphorothioate antisense oligonucleotides, to be used as non-viral gene delivery systems. Their gene silencing efficiency has been demonstrated.33
Exploring a different synthetic approach, we decided to use PLGA nanoparticles and anionic carbosilane dendrons as building blocks for the preparation of novel dendronized nanoparticles with antiviral activity (see Fig. 1). Starting from PLGA nanoparticles with different size distributions prepared by nano-emulsion templating,34 a procedure for their surface modification with anionic carbosilane dendrons of different generations, has been developed, without destabilizing the nanoparticle structure. The size, flexibility and multivalency that the nanoparticle scaffold imposes, may afford changes in the antiviral behavior of the anionic dendrons that is necessary to explore, thus obtaining new and improved nanosystems for their use as antiviral agents against the HIV-1 infection.
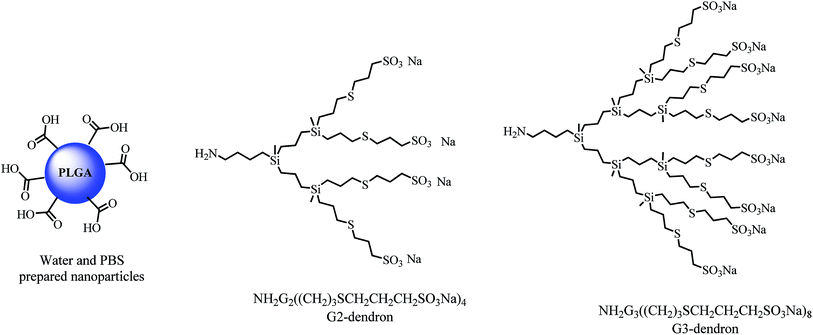 |
| Fig. 1 Structural representation of PLGA nanoparticle and free dendrons. | |
Materials and methods
General information
Unless otherwise stated, reagents were obtained from commercial sources and used as received. Poly(lactic-co-glycolic acid), Resomer 752H in the following abbreviated as PLGA (polystyrene equivalent molecular weight PSE – MW ∼ 10
000 g mol−1, as determined by Gel Permeation Chromathography) was purchased from Boehringer Ingelheim. The lactic acid to glycolic acid ratio of the PLGA used was 75/25 and the end groups were free carboxylic acids. Ethyl acetate used as the organic volatile solvent was purchased from Merck. Polysorbate 80 is a nonionic surfactant kindly provided by Croda. It is a yellow liquid at room temperature and has an HLB value of 15 [Stolnik, 1994]. Salts, used for the electrolyte solution (pH = 7.4) preparation (sodium chloride 8 g L−1, sodium dihydrogen phosphate hydrate 0.219 g L−1 and disodium monohydrogen phosphate dehydrate 2.983 g L−1) were purchased from Merck. Water was MilliQ filtered. Anionic carbosilane dendrons with sulfonate groups at their periphery and an amine group at the focal point where prepared as previously described by our research group.35
Characterization techniques
Thermogravimetric analysis were performed with a Mettler Toledo TGA/DTA 851 device working in a temperature range between 25 and 550 °C and with a heating speed of 10 °C min−1. Dynamic light scattering measurements (DLS) were carried out using a 3D-DLS light scattering (LS Instruments) with a He–Ne laser (λ = 632.8 nm) at 25 °C. The chosen detection angles were 90° and 135°. Measurements of the hard sphere diameters of nanoparticles were performed by transmission electron microscopy (TEM), using a Jeol 1010 TEM and the ImageJ software. The given measures correspond to the mean of at least 500 nanoparticles measured. Fourier transform infrared spectrometry (FTIR) profiles were registered in a Nicolet-6700 device. The samples were dispersed in KBr for their analysis and the results were recorded as absorbance variation. Zeta potential measurements were carried out at 25 °C with a Malvern Instrument Zetasizer Nano Z by laser Doppler electrophoresis.
Preparation and dendronization of nanoparticles
General procedure. Polymeric O/W nano-emulsions were prepared using the phase inversion composition method (PIC) (Solans, 2012). A total of 4 g of each sample was prepared. A mixture of oil and surfactant was mixed and the aqueous phase was stepwise added (at different electrolyte concentrations). The region of O/W nano-emulsion formation was assessed visually, in the pseudoternary water/surfactant/oil diagram, observing the macroscopic appearance of the samples under a spotlight. Samples with transparent, translucent or slightly opaque aspect, having a bluish or reddish shine were considered nano-emulsions. The ethyl acetate was evaporated from the nano-emulsion droplets under reduced pressure (43 mbar, 45 min, 25 °C) to enable the formation of polymeric nanoparticles using the solvent evaporation method (Desgouilles, 2003). The functionalization of the nanoparticles with carbosilane dendrons was performed by using the carbodiimide reaction, linking the free carboxylic groups of the polymer along with the amine focal group of the dendrons through an amide bond.Suspension of the as-prepared nanoparticle dispersion (NPs), dispersed in destilated H2O, were acidified with H3PO4 (pH ≤ 5.0). The carboxylic groups of the PLGA were activated adding first N′-ethylcarbodiimide hydrochloride and then adding N-hydroxysuccinimide to the nanoparticle dispersion during at least two hours at room temperature (25 °C). After this time, activated nanoparticle dispersion was centrifuged at 500 rpm during 30 min. The supernatant was eliminated and the precipitate was re-dissolved in distilled water. Then the suspensions were basified using NEt3 (0.1 μL) and a water solution of carbosilane dendron was added previously basificated with NEt3. Both components were mixed and stirred for at least 18 h, at room temperature (25 °C) to achieve their covalent binding. The functionalized nanoparticles were purified to remove the remaining reactants using a dialysis bag with a MWCO = 10
000–12
000 Da for 1 day in water.
NP(H2O)G2(CBS)(SCH2CH2CH2SO3Na)4 (1). Starting from PLGA (12.5 mg, 1.25 × 10−3 mmol), I (1.69 mg, 1.81 × 10−3 mmol), EDC (0.35 mg, 1.81 × 10−3 mmol), NHS (0.2 mg, 1.81 × 10−3 mmol). White solid. Rh (DLS): 114.91 ± 5.82 nm. ζ potential −48.23 ± 0.67 mV. FTIR (cm−1):
3433 (NH st), 2946 (νs CH2, CH3), 1759 (C
O, st), 1628 (NH δ y N–C
O st sim), 1188 (SO3 st as, CH2, CH3 PLGA), 1095 (νs COC).
NP(H2O)G3(CBS)(SCH2CH2CH2SO3Na)8 (2). Starting from PLGA (11.4 mg, 1.25 × 10−3 mmol), II (3.26 mg, 1.34 × 10−3 mmol), EDC (0.32 mg, 1.70 × 10−3 mmol), NHS (0.20 mg, 1.70 × 10−3 mmol). White solid. Rh (DLS): 124.55 ± 7.41 nm. ζ potential −45.58 ± 1.16 mV. FTIR (cm−1):
3425 (NH st), 2946 (νs CH2, CH3), 1759 (C
O, st), 1628 (NH δ y N–C
O st sim), 1188 (SO3 st as, CH2, CH3 PLGA), 1095 (νs COC).
NP(PBS)G2(CBS)(SCH2CH2CH2SO3Na)4 (3). Starting from PLGA (18.4 mg, 1.84 × 10−3 mmol), 93 (2.60 mg, 2.21 × 10−3 mmol), EDC (0.53 mg, 2.75 × 10−3 mmol), NHS (0.32 mg, 2.75 × 10−3 mmol). White solid. Rh (DLS) 30.83 ± 11.20 nm. ζ potential −26.17 ± 3.77 mV. FTIR (cm−1):
3471 (NH st), 2924 (νs CH2, CH3), 1751 (C
O, st), 1643 (NH δ y N–C
O st sim), 1188 (SO3 st as, CH2, CH3 PLGA).
NP(PBS)G3(CBS)(SCH2CH2CH2SO3Na)8 (4). Starting to PLGA PLGA (39.8 mg, 3.98 × 10−3 mmol), 94 (11.4 mg, 4.78 × 10−3 mmol), EDC (1.14 mg, 5.97 × 10−3 mmol), NHS (0.69 mg, 5.97 × 10−3 mmol). White solid. Rh (DLS) 26.93 ± 1.67 nm. ζ potential see two peaks −10, −50 mV. FTIR (cm−1):
3471 (NH st), 2924 (νs CH2, CH3), 1751 (C
O, st), 1643 (NH δ y N–C
O st sim), 1188 (SO3 st as, CH2, CH3 PLGA).
Biomedical methods
Cells. Blood samples were obtained from healthy anonymous donors from the transfusion centers of Albacete and Madrid following national guidelines (Specific legislation on biobanks in Spain).36 Peripheral blood mononuclear cells (PBMC) were isolated on a Ficoll-Hypaque density gradient (Rafer, Spain) following the current procedures of Spanish HIV HGM BioBank (Spanish HIV BioBank: a model of cooperative HIV research).37 In both cases, the samples were from anonymous donors, therefore being not necessary, under the Spanish laws, to include any statement about informed consent for any experimentation with human subjects from the institutional committees. PBMC were cultured in RPMI 1640 medium (Gibco, UK) supplemented with 10% heat-inactivated FBS, 1% L-glutamine and antibiotic cocktail (125 mg mL−1 ampicillin, 125 mg mL−1 cloxacillin and 40 mg mL−1 gentamicin; Sigma, St-Louis, MO, USA). PBMC were cultured with 60 mg mL−1 of interleukin-2 (IL-2, Bachem, Switzerland) and stimulated with phytohemagglutinin (PHA, 1 μg mL−1, Remel, Santa Fe, USA) for 48 h. HEC-1A (ATCC) is a human epithelial cell line derived from a human endometrial carcinoma (uterus mucosa carcinoma) and was grown in McCoy's 5A Medium Modified (Biochrom AG, Germany) supplemented with 10% FBS and antibiotics cocktail.
Virus production. Virus stocks were prepared by amplification of X4-HIV-1NL4.3 in MT-2 cell line (ATCC). Physical titers of all HIV-1 stocks were evaluated by quantification of HIV p24gag antigen by ELISA kit (Innogenetics, Belgium).
Cell viability assays. Cell viability of HEC-1A and PBMC cells was determined by measuring the metabolic activity of the cells after 24 hours using the MTT (3-(4,5-dimethylthiazol-2-yl)-2,5-diphenyltetrazolium bromide) (Promega, Madison, WI, USA) assay following manufacturer's instructions. We included dextran (negative control) to evaluate its innocuous effect in cell cultures, dymethyl sulfoxide (DMSO) 10% (Sigma-Aldrich) as positive control of cell death and PBS as negative control. Each experiment was performed in triplicate.
Inhibition of HIV replication. Cells were treated with the desired compounds for 1 h. They were washed and then were infected for 3 h with 100 ng per 106 cells of X4-HIV-1NL4.3 strain and further extensively washed. After 24 h, supernatant of infected human epithelial cells were collected and HIV was measured using the p24gag ELISA kit.
Results and discussion
Synthesis of dendronized nanoparticles
PLGA nanoparticles (NP) with two different size distributions were selected (see Table 1). These nanoparticles were obtained by solvent evaporation from template O/W nano-emulsions, which were, in turn, prepared by the phase inversion composition (PIC) method.32,34 The template nano-emulsions were formulated using the same components: 4 wt% PLGA in ethyl acetate as the oil component, polysorbate 80 as the surfactant, and 90 wt% of aqueous phase; the oil-to-surfactant (O/S) weight ratio was 70/30. The aqueous phase was (a) distilled water (NPH2O) which presented a hydrodynamic radius by DLS of 138.41 ± 35.99 nm and a hard sphere diameter of 100.95 ± 69.75 nm or (b) 0.16 M phosphate buffered saline (PBS) solution, where smaller nanoparticles (NPPBS) were formed, with hydrodynamic radii of 20.24 ± 6.98 and hard sphere diameters of 32.75 ± 8.42 nm. In both cases, after nanoparticle formation, the terminal carboxylic acid group for each polymer chain is ideally directed to the surface of the nanoparticle, since it is the hydrophilic part of the polymeric chain, and it can be accessible for its chemical modification. These carboxylic acid moieties provide nanoparticles with a negative surface charge, as can be measured by zeta potential ζ (−33.88 and −12.60 mV for aqueous and PBS nanoparticles respectively). Therefore, anionic carbosilane dendrons with an amino group at the focal point [NH2Gn(CBS)(SCH2CH2CH2SO3Na)m] (where n = 2, m = 4 (I); n = 3, m = 8 (II); and CBS denotes carbosilane scaffold, see also Fig. 1) were selected to be coupled through the formation of a peptidic bond with the carboxylic acid groups presented at the surface of the nanoparticles. In order to prepare the desired dendronized nanoparticles, the usual synthetic protocol for the preparation of a peptidic bond was followed.30,38 The activation of the carboxylic acid groups of the nanoparticle was first performed in the presence of excess of 1-ethyl-3-(3-dimethylaminopropyl)carbodiimide (EDC) and N-hydroxysuccinimide (NHS) in an acidic aqueous media (pH = 4) (Scheme 1). The NPH2O generate a pH ca. 5 because of the presence of the carboxylic groups while those prepared in PBS buffer afford a pH around 7.2. The pH was adjusted to 4 by addition of orthophosphoric acid. After the addition of EDC and NHS, the solutions were stirred for 2 h, and centrifuged for 30 min at 5000 rpm. The solution was then removed thus eliminating the EDC and NHS present in the solution and the nanoparticle pellet redissolved in water. Small nanoparticles (prepared in PBS) could not be centrifuged due to their smaller size. Nevertheless, the sample was allowed to proceed to the next reaction step and the purification was performed after the dendronization was completed.
Table 1 Physicochemical characterization data of NPH2O, NPPBS, carbosilane dendrons (I, II), dendronized NPH2O (compounds 1, 2) and NPPBS (compounds 3, 4)
|
ζ (mV) |
Rh (nm) |
NPH2O |
−33.88 ± 0.23 |
138.41 ± 35.99 |
NPPBS |
−12.60 ± 1.19 |
20.24 ± 6.98 |
(I) |
−54.63 ± 0.67 |
35.21 ± 17.05 |
(II) |
−61.03 ± 0.21 |
19.48 ± 9.58 |
1 |
−48.23 ± 0.67 |
114.91 ± 5.82 |
2 |
−45.58 ± 1.16 |
124.55 ± 7.41 |
3 |
−26.17 ± 3.77 |
30.83 ± 11.20 |
4 |
−10/−50 |
26.93 ± 1.67 |
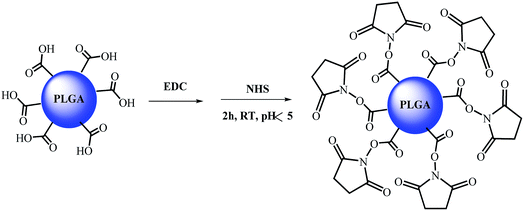 |
| Scheme 1 Synthesis of activated nanoparticles. | |
Finally, both the nanoparticle dispersions and water solutions of the desire dendrons were treated with triethylamine (Et3N) (0.1 μL) during 30 min prior to their mixing during one night at room temperature. The desired products were purified by dialysis against 1 L of distilled water during one day with cellulose membranes with a cutoff limit of 10
000–12
000 Da, in order to eliminate the excess of the reagents and the free dendrons. In a first synthesis, the dendronized nanoparticles were freeze-dried to obtained the desire products [NP(X)Gn(CBS)(SCH2CH2CH2SO3Na)m] (where X = H2O, n = 2, m = 4 (1); n = 3, m = 8 (2); and X = PBS, n = 2, m = 4 (3); n = 3, m = 8 (4)) as white solids (see Scheme 2) that allow their complete characterization. However, as these compounds cannot be later dissolved in water solution, in a subsequent synthesis they were kept in solution after their purification to be used in the biomedical assays.
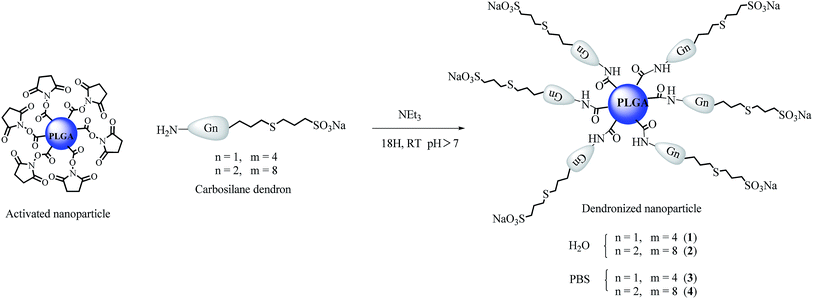 |
| Scheme 2 Synthesis of dendronized nanoparticles 1–4. | |
Attempts to dendronize partially the surface of the nanoparticle using sub-stoichiometric ratio, i.e., leaving some free carboxylic acids that could be used in further functionalizations, failed because no reproducible results were obtained. It could be caused by a non-homogeneous functionalization of the nanoparticle surface, giving rise to some regions more functionalized than others. In addition, a precipitation of the nanoparticles occurred, which could be attributed to the fact that the partial functionalization leaves free acid groups able to initiate the hydrolysis of the NPs in the acid media needed for the dendronization process.
Characterization of dendronized nanoparticles
A qualitative Kaiser test was performed, as described in the literature, in order to detect the presence of free amine groups39 as a way to determine the absence of the free carbosilane dendron in the purified product. The performed Kaiser test confirmed the complete purification of the dendronized nanoparticles.
Zeta potential (ζ) was measured in order to follow the changes in the nanoparticle surfaces. Although dendronization with a dendron that supports sulfonate groups in the periphery maintains an anionic surface charge, a change in the obtained values could be expected. As it can be observed from Table 1, ζ values obtained for all dendronized NPs were still negative and were in the middle between the experimental values found for dendrons and free NPs, indicating a change in the surface of original NPs. However, the use of different generations dendrons has not a big influence in the charge enhancement, probably because a bigger size on the dendron also implies some degree of steric hindrance around the nanoparticle. This steric hindrance can also be pointed out as responsible for the presence of two different populations (−10/−50 mV) in dendronized nanoparticle 4 prepared from NPPBS and the third generation dendron (II). In addition, the similar sizes observed for the free NPPBS and third generation dendron (see Table 1) can make the coupling reaction difficult for the same steric reasons. Besides, the functionalized NPH2O (1 and 2) produced more electrostatically stable systems than those generated from NPPBS regarding to the ζ data obtained. However, it is worth mentioning that nanoparticle stability is not only defined by their electrostatic stability; they can be also stabilized sterically. Colloidal stability of NPPBS is higher than those of NPH2O since their sizes are smaller.
The hydrodynamic sizes of the dendronized nanoparticles prepared from NPH2O and NPPBS were measured by DLS (see Table 1). Although changes in hydrodynamic radii are small, a tendency to form smaller size in dendronized nanoparticles 1 and 2 (obtained from NPH2O) is observed. This fact can be explained by the decrease of the hydration layer surrounding nanoparticles, due to the more hydrophilic nature of the dendronized nanoparticles as compared with the non-dendronized nanoparticles, whose surface is hydrophobic due to the PLGA polymer. In the case of dendronized nanoparticles 3 and 4 (obtained from NPPBS) bigger size are detected, probably as consequence of their small size where the surface addition of a dendron layer is noted.
Compounds 1–4 were freeze-dried along with the free carbosilane dendrons and free nanoparticles used in their synthesis, to perform their analysis by means of infrared spectroscopy (FTIR) (see Fig. 2). The presence of a strong band caused by the vibration of the carbonyl bonds presented in the polymeric chains that formed the nanoparticles hides other possible vibrations in this area (1000–1500 cm−1), such as the one arising from the carbonyl group in the new amide bond between the dendron and the nanoparticle. However, a weak band located at 1627 cm−1 corresponding to the N–H bending vibration along with the N–H stretching band around 3500 cm−1 were tentatively attributed to the amide group. In Fig. 2 the IR spectra of the free nanoparticle, free dendron and compound 1, 2 and 4 are depicted. Therefore, by FTIR, some indications of the nanoparticle functionalization were obtained.
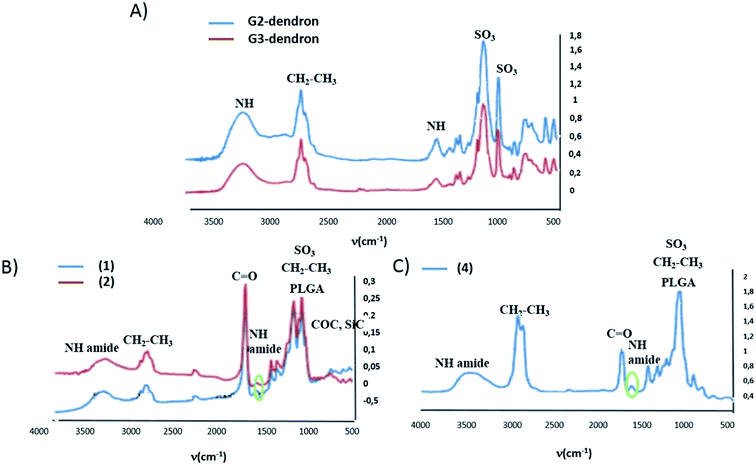 |
| Fig. 2 FTIR spectra, (A) of G2 and G3 dendrons, (B) PLGA nanoparticles 1 and 2 prepared from NP with water as the aqueous phase, (C) PLGA nanoparticles 4 prepared from NP with PBS as the aqueous phase. | |
To further confirm NP dendronization, thermogravimetric analysis was carried out in freeze-dried samples of free nanoparticles, free dendron and dendronized compound 1 (see Fig. 3). Thermal decomposition of compound 1 (Fig. 3a) starts with the loss of a small amount of water (1.2%) around 100 °C associated to water evaporation. The second loss is the major one, when the sample loses 76% of its mass, extended from 163 °C to 407 °C and ascribed to the polymeric degradation, as it is produced at the same range as described previously for PLGA nanoparticles.40–42 There is still a small loss, 7.3%, initiated at 409 °C, attributed to the carbosilane scaffold.43,44 The derivation of the profiles (Fig. 3C) confirms chemical changes because the profile of the product does not match with the physical addition of the nanoparticle and the dendron profiles.
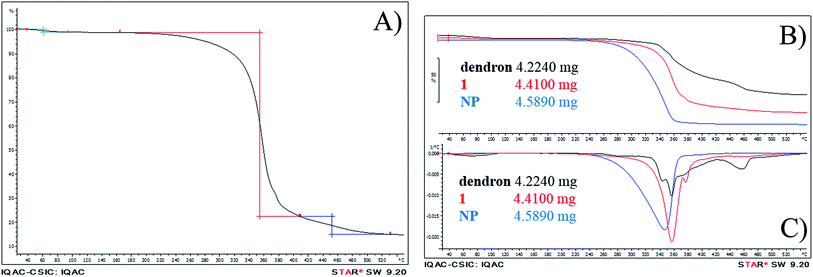 |
| Fig. 3 (A) TGA profile of compound 1; (B) TGA profiles of dendron I (black), compound 1 (red) and non-functionalized nanoparticle (blue); (C) derivation of the TGA profiles of dendron I (black), compound 1 (red) and non-functionalized nanoparticle (blue). | |
Biological evaluation
Preliminary biomedical experiments were carried out to determine the potential activity of the synthesized compounds 1–4 in their use as anti-HIV agents. Biocompatibility assays testing the dendronized nanoparticles (1–4), along with the free PLGA nanoparticles (NPH2O and NPPBS) and carbosilane dendrons (I and II), were carried out by using human endometrial epithelial cell line HEC-1A as a model for the first barrier of protection against HIV infection in the viral transmission process. In addition, peripheral blood mononuclear cells (PBMC), one of the main target of the HIV infection in the immune system were used, as model for a second barrier of protection. MTT assays were performed in both cell cultures at 24 h with increasing concentrations of the desired compounds and the toxicity threshold established at 80% of metabolic activity. As expected, non-functionalized PLGA nanoparticles (NPH2O and NPPBS) were non-cytotoxic in HEC-1A cells, independently on the concentration tested (see Fig. 4). However, after dendron-functionalization, a concentration dependent cytotoxicity was observed. Only compound 1 can be used in all tested concentrations. Second and third generation free dendrons (tested concentrations correspond to 20, 10, 5 and 1 μM) along with all other dendronized nanoparticles show toxicity at the tested concentrations, with some exceptions regarding low concentration solutions of free dendrons and compound 3. Second generation dendrons, and nanoparticles decorated with them, present a similar toxicity profile than the corresponding compounds with the third generations. The same cytotoxicity assessment was performed on PBMC cells (see Fig. 5), where less cytotoxicity was found in general. Dendron-functionalized nanoparticles are non-cytotoxic below 0.045 μg μL−1 (5–4.5 μM) with the exception of NP 2 which is only biocompatible below 0.02 μg μL−1. Beside this, the data show that the biocompatibility profile on PBMC was higher than that observed on HEC-1A cell line.
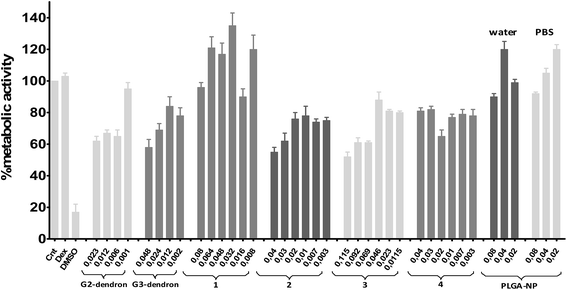 |
| Fig. 4 Viability at 24 h in the HEC-1A cell line by MTT. Cellular viability compared to positive control (non-treated cells). Compounds concentrations expressed in μg μl−1. | |
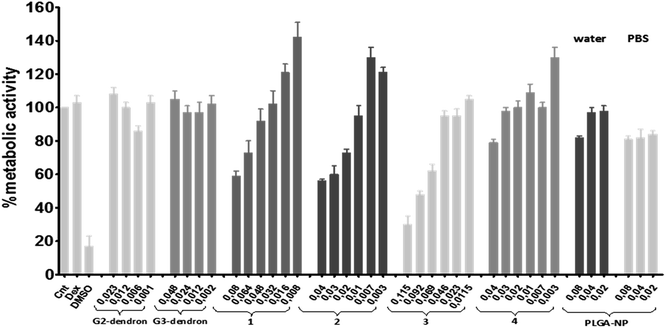 |
| Fig. 5 Viability at 24 h in PBMC by MTT. Cellular viability compared to positive control (non-treated cells). Compounds concentrations expressed in μg μl−1. | |
Anti-HIV activity in PBMCs has been performed and the results shown in Fig. 6. PBMC were pre-treated with free dendrons, free nanoparticles and compounds 1–4 for 1 hour, and then infected with X4-HIV-1NL4.3 for 3 h. The HIV-1 infection was followed by quantification of p24gag antigen production in the supernatant of the culture cells after 24 h. An inhibitory effect of p24gag antigen production was observed with all tested compounds 1–4. However, concentrations over or above 0.05 μg μL−1 cannot be considered for compound 4 and none of the tested ones for compound 2, as they are over the toxicity limit for these compounds. G2 dendron present low-moderate antiviral activity, while G3 dendron is very active (≥95%) from 0.012 μg μL−1 (5 μM). Analogously, low activity was observed for non-dendronized NPH2O and NPPBS. Regarding dendronized nanoparticles 1 and 3, which have been modified with a second generation dendron with only four anionic peripheral groups, high inhibition profiles over 95% were obtained. However, the corresponding dendron alone inhibits as far as 70% at the higher concentration measured. Therefore, in this case, the dendronization of the soft NPs induces a different and positive response toward the action against the X4-HIV-1 strain. By increasing the size from free G2 dendron to dendronized NP 1, a more efficient inhibition was detected, suggesting an enhancement of the virus- or cell-receptor nanosystem interactions. Respecting to NP 3, its size is comparable to that showed for the second generation dendron I. However, the different behavior must be ascribed to the formation of a dynamic aggregate in the case of I that may diminish the interactions towards the virion compared to the compact structure of the nanoparticle. The average size of the HIV-1 virion is about 145 nm,45 while the size of NP 1 is ca. 230 nm and NP 3 of ca. 60 nm. Recently, anionic polyvalent AuNP reported elsewhere,46 have shown that larger systems than vesicular stomatitis virus nanoparticle act as efficient cross-linkers between virions, whereas smaller AuNps decorate the surface of individual virus particles with reduced action. Probably, the same situation may arise for NP 1 and 3, although no differences in the activity were obtained in our case. G3 dendron with eight negative charges exhibits similar activity that NP 1 and 3, although at the lower concentration measured the positive effect of the nanoparticle nature is still noted. Furthermore, higher inhibitions for free G3 dendron and dendronized nanoparticles 1 and 3 were measured when compared with previously described spherical anionic carbosilane dendrimers.20,46 While spherical dendrimers have sixteen negative charges, the third generation dendron and dendronized systems contains only eight and four anionic groups per dendritic wedge respectively. This feature confirms how the topology and size may influence on the therapeutic response necessary to achieve better antiviral properties.
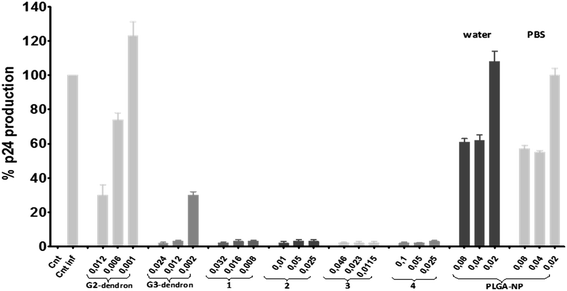 |
| Fig. 6 p24gag antigen production compared to control of non-treated infected on PBMC cells (Cnt, control and inf, HIV-1 infection), quantified by ELISA in pre-treatment conditions. Compounds concentrations expressed in μg μl−1. | |
Conclusions
We have reported an easy and versatile synthetic route to dendronized PLGA nanoparticles prepared in water or PBS with anionic carbosilane dendrons. The obtained compounds have been thoroughly characterized in order to confirm the formation of the desired link between dendrons and the PLGA NPs. Dendronized NP along with the corresponding carbosilane dendrons were investigated as antiviral agents. This study constitutes the first evaluation of modified PLGA nanoparticles as pharmaceutical drugs per se, as far as we concerned. The inhibitory behavior of the presented compounds implies an increase on the antiviral activity for the G2 dendrons when they are supported on PLGA nanoparticles. The topology and size of these new systems could be responsible for the higher activity observed, suggesting an enhancement of the virus-receptors or cell-receptor nanosystem interactions. The excellent HIV inhibitory activities concerning both G3 dendrons and G2 dendronized nanoparticles open a wide range of new possibilities, where dendrons could be attached to other nanostructured materials providing new and potential antiviral systems to explore. In addition, the use of nanoparticles, enables the more controlled therapy in terms of arriving to the desired site of action and producing a local response when nanoparticles are functionalized, decreasing side effects.47 Moreover, these findings could represent a promising advancement in the development of anti-HIV treatments, since, apart from the great results found here thanks to the anti-HIV activity produced by the dendronization, some drugs could be encapsulated in these nanoparticles to achieve a combined therapy to treat HIV.
Acknowledgements
This work has been supported by grants CTQ-2014-54004-P and CTQ2011-29336-CO3-O1 (from MINECO), CCG2014/EXP010 (from UAH), 2014-SGR-1655 (from Generalitat de Catalunya) and Consortium NANODENDMED ref S2011/BMD-2351 (CM). CIBER-BBN as an initiative funded by the VI National R&D&i Plan 2008–2011, Iniciativa Ingenio 2010, Consolider Program, CIBER Actions and financed by the Instituto de Salud Carlos III with assistance from the European Regional Development Fund. Fondo Europeo de Desarrollo Regional (FEDER), RETIC PT13/0010/0028, Fondo de Investigacion Sanitaria (FIS) (grant number PI13/02016, PI14/00882 (JLJ) and PI12/0014), Comunidad de Madrid (grant numbers S-2010/BMD-2332), CYTED 214RT0482 to HGUGM. This work has been (partially) funded by the RD12/0017/0037, project as part of the project as part of the Acción Estratégica en Salud, Plan Nacional de Investigación Científica, Desarrollo e Innovación Tecnológica 2008–2011 (HGUGM) and "Programa de Investigación de la Consejería de Sanidad de la Comunidad de Madrid” to JLJ. This work was supported partially by a Marie Curie International Research Staff Exchange Scheme Fellowship within the 7th European Community Framework Program, project No. PIRSES-GA-2012-316730 NANOGENE, co-financed by the Polish Ministry of Science and Higher Education (grant No. W21/7 PR/2013). Cristina Fornaguera acknowledges AGAUR for their Predoctoral Fellowship (grant FI-DGR 2012). We want to particularly acknowledge to the Spanish HIV HGM BioBank that is supported by the Spanish Instituto de Salud Carlos III and is integrated in the Spanish AIDS Research Network. The authors thank Dr Ma Isabel Clemente, of Culture Unit (IiSGM, Madrid, Spain) for their technical assistance.
References
- S. Nayak and L. A. Lyon, Angew. Chem., Int. Ed., 2005, 44, 7686–7708 CrossRef CAS PubMed.
- M. Elsabahy and K. L. Wooley, Chem. Soc. Rev., 2012, 41, 2545–2561 RSC.
- J. Estelrich, M. Quesada-Perez, J. Forcada and J. Callejas-Fernandez, RSC Nanosci. Nanotechnol., 2014, 34, 1–18 CAS.
- C. E. Astete and C. M. Sabliov, J. Biomater. Sci., Polym. Ed., 2006, 17, 247–289 CrossRef CAS PubMed.
- F. Danhier, E. Ansorena, J. M. Silva, R. Coco, A. Le Breton and V. Préat, J. Controlled Release, 2012, 161, 505–522 CrossRef CAS PubMed.
- S. Mohammadi-Samani and B. Taghipour, Pharm. Dev. Technol., 2015, 20, 385–393 CrossRef CAS PubMed.
- C. I. C. Crucho and M. T. Barros, Polymer, 2015, 68, 41–46 CrossRef CAS.
- J. Kreuter, Adv. Drug Delivery Rev., 2014, 71, 2–14 CrossRef CAS PubMed.
- E. Kiss, G. Gyulai, C. B. Penzes, M. Idei, K. Horvati, B. Bacsa and S. Bosze, Colloids Surf., A, 2014, 458, 178–186 CrossRef CAS.
- D. Pawar, S. Mangal, R. Goswami and K. S. Jaganathan, Eur. J. Pharm. Biopharm., 2013, 85, 550–559 CrossRef CAS PubMed.
- M. Mooguee, Y. Omidi and S. Davaran, J. Pharm. Sci., 2010, 99, 3389–3397 CrossRef CAS PubMed.
- S. Ribeiro, N. Hussain and A. T. Florence, Int. J. Pharm., 2005, 298, 354–360 CrossRef CAS PubMed.
- K. Nazemi, P. Azadpour, F. Moztarzadeh, A. M. Urbanska and M. Mozafari, Mater. Lett., 2015, 138, 16–20 CrossRef CAS.
- M. Mehrasa, M. A. Asadollahi, K. Ghaedi, H. Salehi and A. Arpanaei, Int. J. Biol. Macromol., 2015, 79, 687–695 CrossRef CAS PubMed.
- F. S. T. Mirakabad, K. Nejati-Koshki, A. Akbarzadeh, M. R. Yamchi, M. Milani, N. Zarghami, V. Zeighamian, A. Rahimzadeh, S. Alimohammadi, Y. Hanifehpour and S. W. Joo, Asian Pac. J. Cancer Prev., 2014, 15, 517–535 CrossRef.
- K. Zhao, W. Li, T. Huang, X. Luo, G. Chen, Y. Zhang, C. Guo, C. Dai, Z. Jin, Y. Zhao, H. Cui and Y. Wang, PLoS One, 2013, 8, e80374 Search PubMed.
- F. Fay and C. J. Scott, Drug Discovery Today, 2010, 15, 1087–1088 CrossRef.
- C. Fornaguera, A. Dols-Perez, G. Caldero, M. J. Garcia-Celma, J. Camarasa and C. Solans, J. Controlled Release, 2015, 211, 134–143 CrossRef CAS PubMed.
- D. Sepulveda-Crespo, J. Sanchez-Rodriguez, M. Jesus Serramia, R. Gomez, F. Javier De La Mata, J. Luis Jimenez and M. Angeles Munoz-Fernandez, Nanomedicine, 2015, 10, 899–914 CrossRef CAS PubMed.
- J. Sanchez-Rodriguez, L. Diaz, M. Galan, M. Maly, R. Gomez, F. Javier de la Mata, J. L. Jimenez and M. Angeles Munoz-Fernandez, J. Biomed. Nanotechnol., 2015, 11, 1783–1798 CrossRef CAS PubMed.
- E. Vacas-Cordoba, F. J. De la Mata, R. Gomez, M. Pion and M. Angeles Munoz-Fernandez, AIDS
Res. Hum. Retroviruses, 2014, 30, A204 CrossRef.
- D. Sepulveda-Crepo, J. Sanchez-Rodriguez, M. Jesus Serramia, A. Lopez, E. Alonso, R. Gomez, F. Javier De La Mata, J. Luis Jimenez and M. Angeles Munoz-Fernandez, AIDS Res. Hum. Retroviruses, 2014, 30, A205 CrossRef.
- M. Galan, E. Fuentes-Paniagua, F. Javier de la Mata and R. Gomez, Organometallics, 2014, 33, 3977–3989 CrossRef CAS.
- E. Fuentes-Paniagua, C. E. Pena-Gonzalez, M. Galan, R. Gomez, F. Javier de la Mata and J. Sanchez-Nieves, Organometallics, 2013, 32, 1789–1796 CrossRef CAS.
- R. Andres, E. de Jesus, F. J. de la Mata, J. C. Flores and R. Gomez, Eur. J. Inorg. Chem., 2005, 3742–3749 CrossRef CAS.
- J. I. Paez, M. Martinelli, V. Brunetti and M. C. Strumia, Polymers, 2012, 4, 355–395 CrossRef.
- J. I. Paez, E. A. Coronado and M. C. Strumia, J. Colloid Interface Sci., 2012, 384, 10–21 CrossRef CAS PubMed.
- K. R. Gopidas, J. K. Whitesell and M. A. Fox, J. Am. Chem. Soc., 2003, 125, 6491–6502 CrossRef CAS PubMed.
- S. T. Kim, A. Chompoosor, Y.-C. Yeh, S. S. Agasti, D. J. Solfiell and V. M. Rotello, Small, 2012, 8, 3253–3256 CrossRef CAS PubMed.
- L. Costantino, F. Gandolfi, L. Bossy-Nobs, G. Tosi, R. Gurny, F. Rivasi, M. Angela Vandelli and F. Forni, Biomaterials, 2006, 27, 4635–4645 CrossRef CAS PubMed.
- S. Ribeiro, S. G. Rijpkema, Z. Durrani and A. T. Florence, Int. J. Pharm., 2007, 331, 228–232 CrossRef CAS PubMed.
- G. Caldero, M. J. Garcia-Celma and C. Solans, J. Colloid Interface Sci., 2011, 353, 406–411 CrossRef CAS PubMed.
- C. Fornaguera, S. Grijalvo, M. Galan, E. Fuentes-Paniagua, F. Javier de la Mata, R. Gomez, R. Eritja, G. Caldero and C. Solans, Int. J. Pharm., 2015, 478, 113–123 CrossRef CAS PubMed.
- C. Solans and I. Solé, Curr. Opin. Colloid Interface Sci., 2012, 17, 246–254 CrossRef CAS.
- M. Galan, E. Fuentes-Paniagua, F. J. de la Mata and R. Gomez, Organometallics, 2014, 33, 3977–3989 CrossRef CAS.
- I. M. García-Merino, I. Consuegra, J. L. Jiménez and M. Á. Muñoz-Fernández, Biopreserv. Biobanking, 2015, 13, 207–211 CrossRef PubMed.
- I. García-Merino, N. de las Cuevas, J. L. Jiménez, J. Gallego, C. Gómez, C. Prieto, M. J. Serramía, R. Lorente and M. Á. Muñoz-Fernández, Retrovirology, 2009, 6, 1–5 CrossRef PubMed.
- T. Betancourt, J. D. Byrne, N. Sunaryo, S. W. Crowder, M. Kadapakkam, S. Patel, S. Casciato and L. Brannon-Peppas, J. Biomed. Mater. Res., Part A, 2009, 91, 263–276 CrossRef PubMed.
- E. Kaiser, R. L. Colescot, C. D. Bossinge and P. I. Cook, Anal. Biochem., 1970, 34, 595–598 CrossRef CAS PubMed.
- M. Oroujzadeh, S. Mehdipour-Ataei and M. Esfandeh, Eur. Polym. J., 2013, 49, 1673–1681 CrossRef CAS.
- X. F. Li, C. J. Zhao, H. Lu, Z. Wang and H. Na, Polymer, 2005, 46, 5820–5827 CrossRef CAS.
- F. Wang, T. Chen and J. Xu, Macromol. Chem. Phys., 1998, 199, 1421–1426 CrossRef CAS.
- S.-J. Wang, X.-D. Fan, X. Liu, J. Kong, Y.-Y. Liu and X. Wang, Polym. Int., 2007, 56, 764–772 CrossRef CAS.
- H. Kudo, Y. Fujiwara, M. Miyasaka and T. Nishikubo, J. Polym. Sci., Part A: Polym. Chem., 2010, 48, 5746–5751 CrossRef CAS.
- J. A. G. Briggs, T. Wilk, R. Welker, H.-G. Kräusslich and S. D. Fuller, EMBO J., 2003, 22, 1707–1715 CrossRef CAS PubMed.
- V. Briz, D. Sepulveda-Crespo, A. R. Diniz, P. Borrego, B. Rodes, F. J. de la Mata, R. Gomez, N. Taveira and M. A. Munoz-Fernandez, Nanoscale, 2015, 7, 14669–14683 RSC.
- C. Vauthier and K. Bouchemal, Pharm. Res., 2008, 26, 1025–1058 CrossRef PubMed.
Footnote |
† Electronic supplementary information (ESI) available. See DOI: 10.1039/c6ra13461k |
|
This journal is © The Royal Society of Chemistry 2016 |
Click here to see how this site uses Cookies. View our privacy policy here.