DOI:
10.1039/C5SC02630J
(Edge Article)
Chem. Sci., 2016,
7, 339-345
A straightforward method for automated Fmoc-based synthesis of bio-inspired peptide crypto-thioesters†
Received
20th July 2015
, Accepted 22nd September 2015
First published on 23rd September 2015
Abstract
Despite recent advances, the direct Fmoc-based solid phase synthesis of peptide α-thioesters for the convergent synthesis of proteins via native chemical ligation (NCL) remains a challenge in the field. We herein report a simple and general methodology, enabling access to peptide thioester surrogates. A novel C-terminal N-(2-hydroxybenzyl)cysteine thioesterification device based on an amide-to-thioester rearrangement was developed, and the resulting peptide crypto-thioesters can be directly used in NCL reactions with fast N → S shift kinetics at neutral pH. These fast kinetics arise from our bio-inspired design, via intein-like intramolecular catalysis. Due to a well-positioned phenol moiety, an impressive >50 fold increase in the kinetic rate is observed compared to an O-methylated derivative. Importantly, the synthesis of this new device can be fully automated using inexpensive commercially available materials and does not require any post-synthetic steps prior to NCL. We successfully applied this new method to the synthesis of two long naturally-occurring cysteine-rich peptide sequences.
Introduction
Peptide α-thioesters are key intermediates for the convergent synthesis of proteins through native chemical ligation (NCL), a reaction that has revolutionized the field.1 While peptide thioesters can be directly synthesized through Boc-based solid-phase peptide synthesis (SPPS), their access via the more widely used Fmoc-based strategy is not straightforward due to the instability of the thioester moiety to repeated piperidine treatments used for Fmoc deprotection. Most Fmoc-based methodologies developed to date rely on a post-SPPS conversion of a piperidine-stable precursor into a thioester, but despite considerable efforts, no universal strategy has emerged.2 Thanks to their simple implementation, methods such as Dawson's N-acylurea (Nbz)3 and Liu's hydrazides4 for the generation of thioester precursors have recently become popular, but are still associated with several limitations.5 Thus, a reliable and straightforward route to peptide α-thioesters via Fmoc-SPPS is highly desirable.
A number of strategies based on β- or γ-mercapto amide thioesterification devices have recently appeared that exploit an amide-to-thioester rearrangement6,7 (Fig. 1A), which is reminiscent of the first step of intein-promoted in vivo protein splicing.8 In most cases, the N → S acyl shift is reversible and requires acidic conditions to proceed efficiently, meaning that the peptides have to be first converted into thioesters prior to NCL, which is optimal at pH ∼ 7. A few systems, referred to as crypto-thioesters, are able to operate under NCL conditions, thus enabling one-pot reactions (thioester formation and NCL). Cysteine-prolyl esters (CPEs) developed by Aimoto7d (Fig. 1B-b) rely on an elegant intramolecular O → N shift to displace the amide–thioester equilibrium under NCL conditions, but with slow ligation kinetics. Although simple to implement, the α-methyl cysteine crypto-thioesters recently introduced by Offer7j (Fig. 1B-e) are impaired by similarly slow kinetics. In both cases, the limiting factor is likely the disfavored trans-to-cis isomerization of the amide bond to generate the geometrically feasible intramolecular attack of the thiolate. Use of N,N-disubstituted β-mercapto amides can overpass this limitation by disfavoring the unproductive trans conformer. The symmetrical bis-sulfanylethylamides (SEAs, Fig. 1B-c) introduced by Melnyk7g and Liu7h can be used in NCL at pH 6–7 with relatively fast kinetics, but work better at lower pH. Otaka's N-sulfanylethylanilides7e (Sealides, Fig. 1B-d) exploit the electronic effect of the anilide to enhance the electrophilicity of the amide bond. They are efficient NCL substrates at neutral pH only when using a phosphate-based buffer, probably arising from pseudo-intramolecular acid–base catalysis, but the exact mechanism remains elusive.9 Interestingly, while we were preparing this paper, a method appeared based on an N-ethyl cysteine device featuring a free carboxylic acid; peptides equipped with this device can act as crypto-thioesters. The α-acid group was shown to accelerate the reaction compared to an α-amide-containing analogue, with optimum ligation kinetics at pH 5.5, but with very slow kinetics at pH 7.10
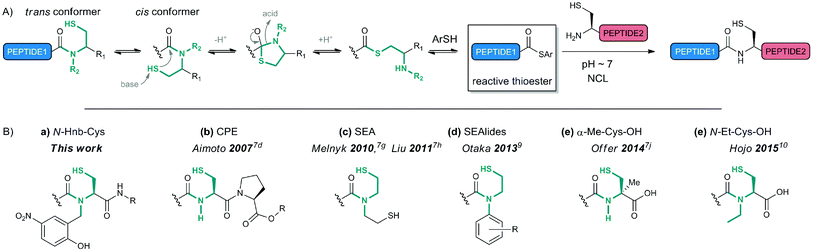 |
| Fig. 1
N → S shift-based in situ synthesis of peptide thioesters for NCL. (A) Mechanism. (B) Crypto-thioesters bearing thioesterification devices operating under NCL conditions. | |
We herein report the design and optimization of a simple and general methodology based on an N-(2-hydroxybenzyl)cysteine device, which can be automatically assembled in the solid-phase using inexpensive commercially available materials. Peptides bearing this device show fast N → S shift kinetics under NCL conditions around neutral pH, likely arising from a biomimetic intein-like intramolecular catalysis mechanism. The synthesis of these novel crypto-thioesters is straightforward and does not require an additional step prior to NCL. We believe that this accessible and robust Fmoc-based thioesterification device provides a significant advance to chemical protein synthesis.
Results and discussion
We based our design on N-alkyl cysteine7c as a scaffold for the design of our thioesterification device, as it can be readily assembled in the solid-phase from inexpensive and commercially available materials.11 However, its N → S shift is usually very slow at neutral pH,12 preventing its direct use in NCL. We focused our efforts on addressing this challenge by taking inspiration from the mechanism of in vivo protein splicing promoted by class 1 inteins. The first step of this process consists of a self-catalyzed N → S acyl shift of an amine into a thioester,8 where a highly conserved histidine residue plays a pivotal role. This N-protonated His is believed to catalyze C–N bond scission through polarization and then protonation of the nitrogen leaving group (Fig. 2A).13 We reasoned that the N-alkyl group of cysteine could be functionalized by an appropriate intramolecular proton donor able to mimic the catalytic histidine side chain, resulting in a much faster N → S shift at neutral pH. Accordingly, we designed an N-(2-hydroxybenzyl)cysteine device (Fig. 2B) leading to a hypothetical thermodynamically favored six-membered transition state for intramolecular N-protonation. The key to this design is a phenol group with a pKa close to neutral, as this would result in a low energy cost for the intramolecular proton transfer at pH 7.
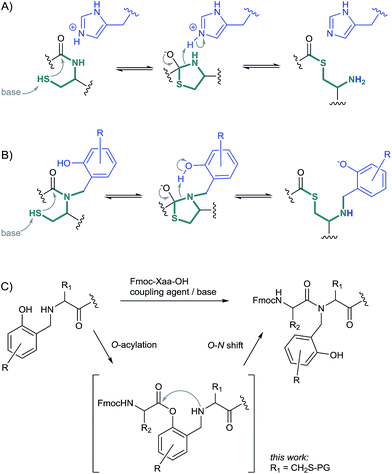 |
| Fig. 2 Rational design of N-(2-hydroxybenzyl)cysteine thioesterification devices. (A) Mechanism of the intein self-catalyzed N → S shift in water. (B) Bio-inspired putative mechanism for the self-catalysis of the N → S shift in N-acyl-N-(2-hydroxybenzyl)cysteine peptides in water. (C) Mechanism of the intramolecular O → N shift in organic solvents shared by N-Hmb peptides and the new N-(2-hydroxybenzyl)cysteine thioesterification devices. PG: protecting group. | |
We expected an additional advantage from the 2-hydroxybenzyl group: acceleration of N-acylation via O-acylation followed by the intramolecular O → N shift (Fig. 2C), as in the N-acylation of 2-hydroxy-5-methoxybenzyl (Hmb) protected amines.14 This could overcome a common drawback encountered with most N → S acyl shift-based thioesterification devices developed so far, the difficult N-acylation of a secondary amine,7g,h,15 to introduce the first residue of the peptide sequence.
Optimization of the hydroxybenzyl group
Three different N-2-hydroxybenzyl groups were introduced on a solid supported cysteine derivative through an automated reductive amination protocol (compounds 2a–c) and were compared with a reference N-ethyl cysteine device (2d). For this preliminary study, a reporter peptide (LYRAG-NH2) was introduced C-terminal to cysteine to facilitate the analysis using LC-MS after TFA-mediated deprotection and cleavage from the resin. Excellent results were obtained with the 2-hydroxy-5-nitrobenzyl (Hnb) group,16 giving quantitative N-acylation yields for a Gly residue under mild amide coupling conditions (HBTU/HOBt) (Table 1, entry 5), while the ethyl-substituted compound (Table 1, entry 7) was unreactive. Hmb or unsubstituted 2-hydroxybenzyl groups (Table 1, entries 1 and 3) showed only moderate assistance. These results are in line with the literature:16 Alewood and collaborators already observed the much superior acyl transfer efficiencies of 2,6- and 2,5-Hnb compared to Hmb. Moreover, the pKa of the hydroxyl group of Hnb is close to 7 (∼6.5, see ESI, Fig. S11†), in line with our requirements discussed above for efficient biomimetic catalysis. We accordingly selected N-Hnb-Cys as our preferred scaffold.
Table 1 Screening of different N-(2-hydroxybenzyl) groups for their ability to enhance the N-acylation yield of a solid-supported S-trityl-protected N-substituted cysteine. PS: polystyrene
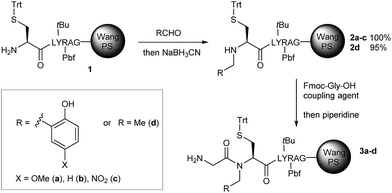
|
Entry |
Resin |
Coupling agenta |
Product |
Yieldb [%] |
All reactions were conducted for 2 h, in DMF, using 10 equiv. Fmoc-Xaa-OH, 9.5 equiv. coupling agent and 20 equiv. iPr2NEt.
Yields determined after TFA-mediated cleavage of the peptide resin using HPLC analysis of the amine-to-amide conversion.
Estimated yields due to closely eluting HPLC peaks.
0.1 equiv. DMAP.
|
1 |
2a
|
HBTU/HOBt |
3a
|
<5c |
2 |
2a
|
HATU |
3a
|
45c |
3 |
2b
|
HBTU/HOBt |
3b
|
11 |
4 |
2b
|
HATU |
3b
|
12 |
5 |
2c
|
HBTU/HOBt |
3c
|
>99 |
6 |
2c
|
HATU |
3c
|
>99 |
7 |
2d
|
HBTU/HOBt |
3d
|
<1 |
8 |
2d
|
HATU |
3d
|
12 |
9 |
2d
|
PyBrop |
3d
|
1 |
10 |
2d
|
HATU/DMAPd |
3d
|
13 |
Optimization of the thiol protecting group of cysteine and linkage to the resin
When exploring the scope of peptides bearing our N-Hnb-Cys thioesterification device, we observed an instability upon purification and storage due to a premature spontaneous N → S shift. Fortunately, the protection of the cysteine thiol as an S-StBu disulfide, stable to Fmoc-SPPS and readily removed under NCL conditions,17 resolved this problem (see ESI, pS24–S29†).18 As the use of a reporter peptide was no longer needed at this point, we looked at introducing Cys(StBu) as either the α-acid or the amide, using a Wang-type or a Rink's amide linker, respectively. Disappointingly, in the former case we were confronted with Cys epimerization19 and β-elimination, followed by the addition of piperidine.20 In the latter case, we observed a TFA-catalyzed hydrolysis21 reaction, leading to a mixture of amide-(7) and acid-terminated (8) compounds (see ESI pS27–S29† for details). Simple incorporation of a Gly spacer between the Rink's linker and the device solved these problems. Preliminary evaluation of NCL reactions with the model cysteinyl peptide 6 showed that this additional residue (compound 9) did not affect the NCL kinetics compared to cysteine α-amide 7, the reaction being even slightly faster than for the α-acid 8 (ESI, Fig. S57†). As expected, cysteine deprotection was very fast, showing the complete removal of the StBu group within minutes.
Study of the N-acylation of the device
N-Acylation of the optimized device was then examined for the twenty proteogenic amino acids, using a standard SPPS protocol (Table 2). In most cases the coupling yields were good to excellent. No significant epimerization was observed, except for Fmoc-Cys(Trt) (7%), which is well known for its propensity for racemization.22 Six residues showed modest N-acylation yields (<50%). In these difficult cases, we looked at further optimizing the coupling, avoiding the use of sensitive or costly reagents, and keeping in mind an easy-to-automate procedure. Three successive couplings of Fmoc-Ser(tBu) resulted in an excellent yield (85%, entry 16). The five-fold coupling of the more demanding Fmoc-Val led to a good 65% yield (entry 21). Alternatively, single coupling under microwave heating at 70 °C led to an excellent 92% yield for Fmoc-Val (entry 22) and 78% for Fmoc-Ile (entry 24). Importantly, the unreacted secondary amine could be quantitatively capped by acetylation, giving a byproduct that can be readily removed during the peptide precipitation step following TFA-based cleavage from the resin.23
Table 2 Acylation of the resin 4 with the 20 different protected proteogenic amino acids under automated coupling conditions. TG: Tentagel
Mechanistic insights
Next, we wanted to assess the validity of our intein-inspired design hypothesis that should result in the self-catalysis of the N → S shift at neutral pH through intramolecular protonation. Here, we synthesized a model peptide equipped with a 2-methoxy-5-nitrobenzylcysteine device (12), designed to inhibit the acid–base properties of the phenol group while not being expected to lead to major changes in terms of steric hindrance compared to Hnb (peptide 9). We were delighted to see that the hydroxyl group very efficiently catalyzes the N → S acyl shift: >50-fold rate enhancement of NCL was measured for 9 compared to 12 under the NCL conditions (Fig. 3), supporting our hypothesis.24
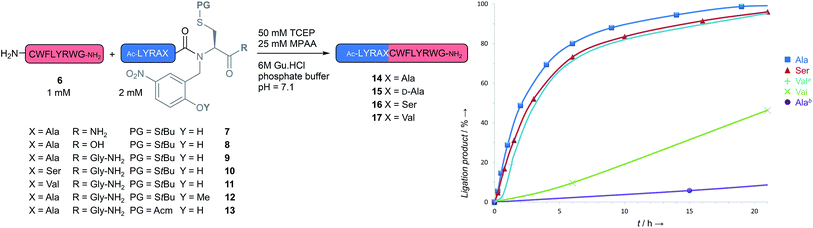 |
| Fig. 3 NCL of the model crypto-thioester peptides 7–11 bearing an N-Hnb-cysteine device, and an analog (12) with the hydroxyl group masked as a methyl ether. a 300 mM MPAA, pH 6.6, 50 °C. b Hnb methyl ether (12). | |
Moreover, the ligation of 9 at different pH values showed the N → S acyl shift/NCL process to be fastest at pH ∼ 6.5, and slightly slower at lower pH (ESI, Fig. S67†). This fits with the pKa of the Hnb group (6.5), suggesting the need for a subtle balance between the phenol group protonation and the thiol group deprotonation (cysteine pKa ∼ 8.5) in influencing the N → S shift. To eliminate a possible N → O shift mechanism, the thiol was protected with a stable acetamidomethyl (Acm) group (13). This NCL-unreactive compound confirms the crucial role of the SH group and provides further data to suggest an intramolecular N → S shift mechanism. Critically, this latter finding also highlights the potential of the N-Hnb-Cys thioester precursors for successive ligations in the N-terminus-to-C-terminus (N-to-C) direction. We are currently evaluating latent crypto-thioesters that could be activated by deprotection of a NCL-stable cysteine protecting group, as has been exploited for related N-methyl Cys,11,25 CPE,26 SEA17 and Sealide27 thioester precursors.
Study of the NCL with model peptides
Next, we turned our attention to the effectiveness of our new thioesterification device in NCL reactions for a range of thioesters bearing different amino acids C-terminal to the N-Hnb-Cys device (Fig. 3). Note that we used relatively dilute conditions for the peptide reactants (∼1 mM) and low concentrations of the 4-mercaptophenylacetic acid catalyst (MPAA, 25 mM).28 Relatively fast NCL kinetics were observed using Ala (peptide 9) and Ser (10) crypto-thioesters, as the reactions were complete after 24 h at 37 °C. For comparison with the NCL kinetics data available in the literature, we determined the apparent second order kinetic constants, with results in the 0.03–0.06 M−1 s−1 range, in line with the reported values for standard NCL with preformed thioesters.29 These data validate the rapid N → S shift promoted by our device. Ligation at valine (crypto-thioester 11) was 10 times slower, as we had anticipated from the steric hindrance of its side chain.30 However, increasing the MPAA concentration to 300 mM, lowering the pH close to the optimum (6.6) and heating to 50 °C led to kinetics comparable to Ala and Ser crypto-thioesters. The latter result suggests that the method could be applied in routine NCL even for peptide sequences known to be kinetically demanding.
Potential epimerization during the N → S-shift/NCL process was rigorously examined by synthesizing a D-Ala-containing product (15) as an HPLC standard. Only trace epimerization (<0.4%) was detected in the crude NCL mixture starting from the L-Ala crypto-thioester 9. We also examined the hydrolysis of the crypto-thioesters into the corresponding acids. All reactions were clean and did not show significant amounts of hydrolysis (<4% for 9 after 24 h and using excess crypto-thioester).
Application of the methodology to two cysteine-rich peptides
Importantly, our new method was applied to the synthesis of two long naturally-occurring cysteine-rich peptide sequences, MT7 and Cg-BigDef1. Muscarinic toxin 7 (MT7), 65 residues, was isolated from green mamba venom.31 MT7 naturally forms a three-finger-fold stabilized by four disulfide bonds. Big defensin 1 (Cg-BigDef1) is a 93-residue host-defense peptide isolated from Japanese oysters and is composed of a hydrophobic N-terminal domain and a β-defensin-like cationic C-terminal domain containing six cysteine residues involved in three disulfide bridges.32
These two peptide sequences were chosen as challenging examples to demonstrate the applicability of our methodology, as their syntheses via the NCL of two segments require long thioesters, 41 and 56 amino acids, respectively. In addition, the N-terminal Cg-BigDef1 domain is a “difficult sequence”,33i.e. hard to synthesize using Fmoc-SPPS.34 Both of the crypto-thioesters (18 and 21) were synthesized and purified in good yields (13% and 7%, see ESI, pS86 and S93†). The two ligations were complete overnight at 1 and 2 mM concentrations, respectively, and showed clean HPLC profiles (Fig. 4). Finally, pure reduced forms of MT7 (20) and Cg-BigDef1 (23) were isolated after HPLC purification in 14% and 18% yields, respectively35 (ESI, Fig. S85 and S93†), demonstrating the utility of the N-Hnb-Cys method.
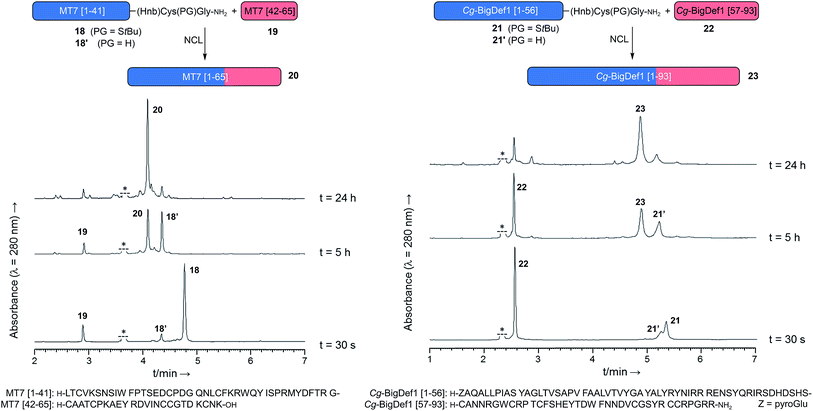 |
| Fig. 4 Application of the N-(2-hydroxy-5-nitrobenzyl)cysteine thioesterification device to the NCL-based syntheses of two long and demanding peptide sequences. | |
Conclusions
In conclusion, we introduced a novel C-terminal thioesterification device, N-Hnb-Cys(StBu), that can rapidly rearrange into a thioester at neutral pH, allowing its use in NCL even under demanding conditions. Fast kinetics likely result from an internal catalysis mechanism provided by a well-positioned phenol moiety arising from a bio-inspired intein-like design. Synthesis from inexpensive commercially available materials and further peptide elongation are straightforward, and can be fully automated on a peptide synthesizer without requiring a post-synthetic step, thus constituting a major advance in the field. Applicability of the strategy was demonstrated by the synthesis of two long naturally occurring cysteine-rich peptide sequences. Further work is ongoing in our laboratory for its application to other synthetically demanding targets.
Acknowledgements
We thank Dr Guillaume Gabant, Dr Cyril Colas and the mass spectrometry platforms of CBM and FR2708 federation for the MS and LC-MS analyses, as well as Hervé Meudal and the CBM NMR platform for the NMR spectra. The Pierre Potier foundation for the chemistry of natural products is gratefully acknowledged for a PhD fellowship for VPT, as well as ANR (JCJC08-0138, SynProt project) and Vaincre la Mucovisidose for financial support. Dr Denis Servent, Dr Gilles Mourier and Dr Pascal Kessler are greatly acknowledged for their expertise and support for the application of the method to MT7, as well as Dr Delphine Destoumieux-Garzón for Cg-BigDef1. Thanks also to Dr Michael Jacobsen and Dr Michael Kay for proof-reading the manuscript.
Notes and references
- P. E. Dawson, T. W. Muir, I. Clark-Lewis and S. B. Kent, Science, 1994, 266, 776–779 CAS.
- F. Mende and O. Seitz, Angew. Chem., Int. Ed., 2011, 50, 1232–1240 CrossRef CAS PubMed.
- J. B. Blanco-Canosa and P. E. Dawson, Angew. Chem., Int. Ed., 2008, 47, 6851–6855 CrossRef CAS PubMed.
- G.-M. Fang, Y.-M. Li, F. Shen, Y.-C. Huang, J.-B. Li, Y. Lin, H.-K. Cui and L. Liu, Angew. Chem., Int. Ed., 2011, 50, 7645–7649 CrossRef CAS PubMed.
- For side reactions observed with the Nbz approach see: S. K. Mahto, C. J. Howard, J. C. Shimko and J. J. Ottesen, ChemBioChem, 2011, 12, 2488–2494 CrossRef CAS PubMed . Note that an article about a second generation Nbz linker was published during the preparation of this manuscript: J. B. Blanco-Canosa, B. Nardone, F. Albericio and P. E. Dawson, J. Am. Chem. Soc., 2015, 137, 7197–7209 CrossRef PubMed . For side reactions observed with the hydrazide approach, see: S. K. Mong, A. A. Vinogradov, M. D. Simon and B. L. Pentelute, ChemBioChem, 2014, 15, 721–733 CrossRef PubMed.
- For reviews on N → S acyl shift-based thioesterification devices, see:
(a) T. Kawakami, Top. Curr. Chem., 2015, 362, 107–135 CrossRef PubMed;
(b) D. Macmillan, A. Adams and B. Premdjee, Isr. J. Chem., 2011, 51, 885–889 CrossRef CAS PubMed.
-
(a) T. Kawakami, M. Sumida, K. Nakamura, T. Vorherr and S. Aimoto, Tetrahedron Lett., 2005, 46, 8805–8807 CrossRef CAS;
(b) F. Nagaike, Y. Onuma, C. Kanazawa, H. Hojo, A. Ueki, Y. Nakahara and Y. Nakahara, Org. Lett., 2006, 8, 4465–4468 CrossRef CAS PubMed;
(c) H. Hojo, Y. Onuma, Y. Akimoto, Y. Nakahara and Y. Nakahara, Tetrahedron Lett., 2007, 48, 25–28 CrossRef CAS;
(d) T. Kawakami and S. Aimoto, Chem. Lett., 2007, 36, 76–77 CrossRef CAS;
(e) S. Tsuda, A. Shigenaga, K. Bando and A. Otaka, Org. Lett., 2009, 11, 823–826 CrossRef CAS PubMed;
(f) J. Kang, J. P. Richardson and D. Macmillan, Chem. Commun., 2009, 407–409 RSC;
(g) N. Ollivier, J. Dheur, R. Mhidia, A. Blanpain and O. Melnyk, Org. Lett., 2010, 12, 5238–5241 CrossRef CAS PubMed;
(h) W. Hou, X. Zhang, F. Li and C.-F. Liu, Org. Lett., 2011, 13, 386–389 CrossRef CAS PubMed;
(i) J.-S. Zheng, H.-N. Chang, F.-L. Wang and L. Liu, J. Am. Chem. Soc., 2011, 133, 11080–11083 CrossRef CAS PubMed;
(j) F. Burlina, G. Papageorgiou, C. Morris, P. D. White and J. Offer, Chem. Sci., 2014, 5, 766–770 RSC.
- For recent reviews on inteins, see:
(a) G. Volkmann and H. D. Mootz, Cell. Mol. Life Sci., 2013, 70, 1185–1206 CrossRef CAS PubMed;
(b) K. V. Mills, M. A. Johnson and F. B. Perler, J. Biol. Chem., 2014, 289, 14498–14505 CrossRef CAS PubMed;
(c) H. H. Shah and T. W. Muir, Chem. Sci., 2014, 5, 446–461 RSC.
- K. Sato, A. Shigenaga, K. Tsuji, S. Tsuda, Y. Sumikawa, K. Sakamoto and A. Otaka, ChemBioChem, 2011, 12, 1840–1844 CrossRef CAS PubMed.
-
(a) Y. Asahina, K. Nabeshima and H. Hojo, Tetrahedron Lett., 2015, 56, 1370–1373 CrossRef CAS;
(b) Y. Asahina, S. Komiya, A. Ohagi, R. Fujimoto, H. Tamagaki, K. Nakagawa, T. Sato, S. Akira, T. Takao, A. Ishii, Y. Nakahara and H. Hojo, Angew. Chem., Int. Ed., 2015, 54, 8226–8230 CrossRef CAS PubMed.
- L. A. Erlich, K. S. Kumar, M. Haj-Yahya, P. E. Dawson and A. Brik, Org. Biomol. Chem., 2010, 8, 2392–2396 CAS.
-
(a) K. S. A. Kumar, L. Spasser, L. A. Erlich, S. N. Bavikar and A. Brik, Angew. Chem., Int. Ed., 2010, 49, 9126–9131 CrossRef CAS PubMed;
(b) M. Haj-Yahya, B. Fauvet, Y. Herman-Bachinsky, M. Hejjaoui, S. N. Bavikar, S. V. Karthikeyan, A. Ciechanover, H. A. Lashuel and A. Brik, Proc. Natl. Acad. Sci. U. S. A., 2013, 110, 17726–17731 CrossRef CAS PubMed;
(c) Y. Ruff, V. Garavini and N. Giuseppone, J. Am. Chem. Soc., 2014, 136, 6333–6339 CrossRef CAS PubMed.
- J. Binschik and H. D. Mootz, Angew. Chem., Int. Ed., 2013, 52, 4260–4264 CrossRef CAS PubMed.
- T. Johnson, M. Quibell, D. Owen and R. C. Sheppard, J. Chem. Soc., Chem. Commun., 1993, 369–372 RSC.
- K. Sakamoto, K. Sato, A. Shigenaga, K. Tsuji, H. Hibino, Y. Nishiuchi and A. Otaka, J. Org. Chem., 2012, 77, 6948–6958 CrossRef CAS PubMed.
- L. P. Miranda, W. D. Meutermans, M. L. Smythe and P. F. Alewood, J. Org. Chem., 2000, 65, 5460–5468 CrossRef CAS PubMed.
- See for example: L. Raibaut, H. Adihou, R. Desmet, A. F. Delmas, V. Aucagne and O. Melnyk, Chem. Sci., 2013, 4, 4061–4066 RSC.
- Instability of thioesterification devices upon handling and purification is a known problem that can be solved by thiol protection to inhibit a premature N → S shift. For example, SEA crypto-thioesters7g can be post-synthetically oxidized to give stable compounds. See also ref. 10a.
- Y. Tawaragi, K. Fuchimura, S. Tanaka, N. Minamino, K. Kangawa and H. Matsuo, Biochem. Biophys. Res. Commun., 1991, 175, 645–651 CrossRef CAS PubMed.
- J. Lukszo, D. Patterson, F. Albericio and S. A. Kates, Lett. Pept. Sci., 1996, 3, 157–166 CrossRef CAS.
-
(a) C. J. Creighton, T. T. Romoff, J. H. Bu and M. Goodman, J. Am. Chem. Soc., 1999, 121, 6786–6791 CrossRef CAS;
(b) M. Teixidó, F. Albericio and E. Giralt, J. Pept. Res., 2005, 65, 153–166 CrossRef PubMed.
- Y. Han, F. Albericio and G. Barany, J. Org. Chem., 1997, 62, 4307–4312 CrossRef CAS PubMed.
- Note that a simple adjustment of the initial amount of resin allows the SPPS of the crypto-thioester sequence to be conducted at the desired scale.
- The hydroxyl group could assist the reaction via a different mechanism to the one we postulated, such as stabilization of the oxyanion moiety of the tetrahedral intermediate. Note that the activation of the amide by hydrogen bonding between the phenol group and the carbonyl oxygen seems unlikely: NMR analysis of a small model peptide showed no significant differences in the cis/trans ratio between the hydroxy and methoxy compounds (see ESI pS14–S23†). A hydrogen bond would be expected to stabilize the cis form and thus modify the ratio. DFT studies are planned to help better understand the mechanism.
- K. S. A. Kumar, S. N. Bavikar, L. Spasser, T. Moyal, S. Ohayon and A. Brik, Angew. Chem., Int. Ed., 2011, 50, 6137–6141 CrossRef CAS PubMed.
- T. Kawakami and S. Aimoto, Tetrahedron Lett., 2007, 48, 1903–1905 CrossRef CAS.
- K. Sato, A. Shigenaga, K. Kitakaze, K. Sakamoto, D. Tsuji, K. Itoh and A. Otaka, Angew. Chem., Int. Ed., 2013, 52, 7855–7859 CrossRef CAS PubMed.
- E. C. B. Johnson and S. B. H. Kent, J. Am. Chem. Soc., 2006, 128, 6640–6646 CrossRef CAS PubMed.
-
(a) S. B. Pollock and S. B. H. Kent, Chem. Commun., 2011, 47, 2342–2344 RSC;
(b) F. Saito, H. Noda and J. W. Bode, ACS Chem. Biol., 2015, 10, 1026–1033 CrossRef CAS PubMed.
- T. M. Hackeng, J. H. Griffin and P. E. Dawson, Proc. Natl. Acad. Sci. U. S. A., 1999, 96, 10068–10073 CrossRef CAS.
- G. Mourier, S. Dutertre, C. Fruchart-Gaillard, A. Ménez and D. Servent, Mol. Pharmacol., 2003, 63, 26–35 CrossRef CAS PubMed.
- R. D. Rosa, A. Santini, J. Fievet, P. Bulet, D. Destoumieux-Garzón and E. Bachère, PLoS One, 2011, 6, e25594 CAS.
- I. Coin, M. Beyermann and M. Bienert, Nat. Protoc., 2007, 2, 3247–3256 CrossRef CAS PubMed.
- Standard Fmoc-SPPS of Cg-BigDef [1–42] C-terminal α-amide gave extremely poor results and low elongation yields, probably arising from its difficult hydrophobic sequence. Considering these results, we optimized the synthesis of the cryptothioester 21 by incorporating multiple coupling steps.
- These isolated yields can appear rather low considering the excellent conversion yield, as determined from the HPLC chromatograms of the crude NCL mixtures, see Fig. 4. We attribute this loss of material to the non-optimized workup and HPLC purification protocols..
Footnote |
† Electronic supplementary information (ESI) available: Detailed synthetic procedures, characterization and kinetics studies. See DOI: 10.1039/c5sc02630j |
|
This journal is © The Royal Society of Chemistry 2016 |
Click here to see how this site uses Cookies. View our privacy policy here.