DOI:
10.1039/C5SC04015A
(Edge Article)
Chem. Sci., 2016,
7, 2506-2515
Polymer coordination promotes selective CO2 reduction by cobalt phthalocyanine†
Received
22nd October 2015
, Accepted 1st February 2016
First published on 2nd February 2016
Abstract
Cobalt phthalocyanine (CoPc) is a known electrocatalyst for the carbon dioxide reduction reaction (CO2RR) that, when adsorbed onto edge-plane graphite (EPG) electrodes, shows modest activity and selectivity for CO production along with co-generation of H2. In contrast, electrodes modified with CoPc immobilized in a poly-4-vinylpridine (P4VP) film show dramatically enhanced activity and selectivity compared to those modified with CoPc alone. CoPc-P4VP films display a faradaic efficiency of ∼90% for CO, with a turnover frequency of 4.8 s−1 at just −0.75 V vs. RHE. Two properties of P4VP contribute to enhancing the activity of CoPc: (1) the ability of individual pyridine residues to coordinate to CoPc and (2) the high concentration of uncoordinated pyridine residues throughout the film which may enhance the catalytic activity of CoPc through secondary and other outer coordination sphere effects. Electrodes modified with polymer-free, five-coordinate CoPc(py) films (py = pyridine) and with CoPc catalysts immobilized in non-coordinating poly-2-vinylpyridine films were prepared to independently investigate the role that each property plays in enhancing CO2RR performance of CoPc-P4VP. These studies show that a synergistic relationship between the primary and outer coordination sphere effects is responsible for the enhanced catalytic activity of CoPc when embedded in the P4VP membrane.
Introduction
The selective electrochemical reduction of CO2 into fuels is an important strategy for the development renewable energy sources. Polycrystalline Cu is currently the state-of-the-art heterogeneous electrocatalyst for carbon dioxide reduction reactions (CO2RR), producing 2–6 e− products at potentials as positive as ∼−0.9 V vs. RHE in aqueous neutral, and near-neutral solutions.1–6 However, Cu-catalyzed CO2RR tends to yield a large distribution of carbon containing products, and the operating potentials are quite negative compared to the thermodynamic potentials of the major products.
In contrast, molecular catalysts show propensity to form a single, typically 2–4 e− CO2 reduction products.7–23 Cobalt phthalocyanine (CoPc) is a well-known molecular CO2RR catalyst.24–32 When adsorbed to graphite electrodes, CoPc is reported to reduce CO2 to CO in aqueous solution. However there is significant co-generation of H2 produced from the reduction of protons in the aqueous electrolyte. Previous studies have shown that incorporating CoPc into a poly-4-vinylpyridine (P4VP) membrane increases the catalyst’s selectivity for the production CO from CO2 over the evolution of H2 from water.33,34 The exact mechanisms for the observed P4VP-enhanced activity and selectivity for CO2 reduction remain unclear. Two properties of the P4VP membrane are believed to contribute to the enhancement of CoPc as a CO2RR catalyst. First, individual pyridine residues of P4VP can coordinate to the square planar cobalt center of CoPc. Axial coordination of pyridine has been implicated in enhanced CO2RR activity for CoPc and cobalt porphyrin catalysts.25,26,28,33,34 Second, the uncoordinated pyridine residues throughout the membrane, which form the secondary and outer coordination spheres of CoPc, also may contribute to the increased activity. In acidic solution some proportion of the pyridine residues will be protonated,33,35,36 and the presence of these protonated residues may enable secondary coordination sphere effects such as hydrogen bonding interactions that can stabilize activated intermediates, and outer sphere effects like the availability of protons around catalyst active sites. However, the mechanism by which P4VP enhances CO2RR by CoPc has not yet been explicitly studied. It is important to determine how P4VP increases the activity and selectivity of CoPc for CO2 reduction, as such information could aid designs of improved immobilized molecular CO2RR catalyst systems that may find wider applications in the emerging field of solar-fuels technology.
In the current work, the properties of P4VP are assessed independently in order determine how each affects CO2RR by CoPc. Electrodes modified with CoPc and CoPc-P4VP were studied using controlled potential electrolysis (CPE) to better understand the base activity of CoPc and the increase in activity that coincides with P4VP encapsulation. As previously reported, large increases in activity and selectivity were observed for CoPc-P4VP over the parent CoPc. We present more a detailed evaluation of CPE data to provide a more comprehensive study of their properties. In particular, we find that CoPc-P4VP is remarkably active for the selective generation of CO, operating in pH 5 solution with an average current density of 2 mA cm−2 and a turnover frequency of 4.8 s−1 with nearly 90% faradaic efficiency for CO production at −1.25 V vs. SCE (−0.73 V vs. RHE). This operating potential is only −0.61 V from the thermodynamic potential of CO production (E0 = −0.125 V vs. RHE) making the CoPc-P4VP system among the most active molecular catalyst reported for the selective reduction of CO2 to any single product in aqueous solution.34,37–44
To investigate the influence of axial coordination of CoPc on the CO2RR activity in the absence of the polymer film, electrodes modified with axially coordinated CoPc(py) were prepared from deposition solutions of CoPc in the presence of pyridine. Likewise, to separate the intrinsic properties of the polymer from axial coordination effects, electrodes with four-coordinate CoPc, and five-coordinate CoPc(py) encapsulated in a non-coordinating poly-2-vinylpyridine (P2VP) membrane were prepared (Fig. 1). Our results suggest that there is a synergistic relationship between axial coordination and the chemical environment imposed by the P4VP membrane that leads to dramatic enhancements in activity observed for CoPc-P4VP.
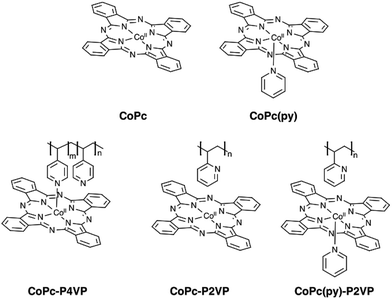 |
| Fig. 1 Proposed molecular structures of CoPc, CoPc-P4VP, CoPc(py), CoPc-P2VP, and CoPc(py)-P2VP. | |
Results
CoPc and CoPc-P4VP
Electrodes modified with CoPc and CoPc-P4VP were prepared to investigate the CO2RR catalytic activity of the free catalyst and the effect of the P4VP support. Films were deposited onto an EPG disc electrode by casting a solution in DMF on the electrode surface and allowing the solvent to evaporate at room temperature. Cyclic voltammograms (CVs) of both films show a broad Co(II/I) reduction between −0.4 and −0.7 V vs. SCE, and a more well defined phthalocyanine centered reduction at ca. −0.9 V vs. SCE (Fig. S1 and S2†). Because the peaks in the CVs were quite broad, the electrochemically active coverage of the catalyst could not be readily determined. Therefore, the total deposited coverage of 1.3 × 10−9 mol cm−2, calculated from the 5 μL of 0.05 mM CoPc solution deposited on each 5 mm diameter electrode, was used in all subsequent calculations. This loading of CoPc was held constant for each deposited film in this study.
Cobalt phthalocyanine is known to be a competent HER catalyst.45–48 Under an atmosphere of N2, scans negative of the phthalocyanine reduction show the onset of a catalytic wave attributed to HER at approximately −1.04 V vs. SCE for CoPc modified electrodes and −1.08 vs. SCE for electrodes modified with CoPc-P4VP. Under a CO2 atmosphere, the onset of the catalytic wave shifts slightly positive to −1.0 V vs. SCE, for both films as shown in Fig. 2. The peak current in the presence of CO2 of both films in static CVs is approximately 0.75 mA cm−2. When performed at 1600 rpm (to ensure steady state delivery of substrate to the electrode surface), rotating disk electrode voltammograms (RDEVs) of CoPc-P4VP display much greater peak currents than CoPc (Fig. 3A and B, respectively). The more negative onset potential for H2 evolution with CoPc-P4VP modified electrodes suggests that the P4VP film suppresses HER compared to free CoPc. However, the onset of the catalytic wave under CO2 was not affected by the presence of the polymer film indicating that P4VP does not similarly suppress CO2RR activity of CoPc.
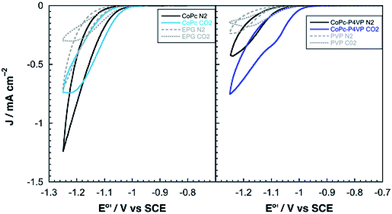 |
| Fig. 2 Representative static cyclic voltammograms of (left) CoPc and (right) CoPc-P4VP under N2 and CO2. | |
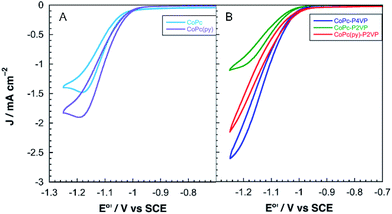 |
| Fig. 3 Representative rotating disk electrode voltammograms at 1600 rpm of (A) the free catalysts CoPc (light blue) and CoPc(py) (purple) and (B) polymer immobilized catalysts CoPc-P4VP (dark blue), CoPc-P2VP (green) and CoPc(py)-P2VP (red) under an atmosphere of CO2. | |
CPE experiments were performed at −1.25 V vs. SCE to assess faradaic efficiencies for CO2 reduction by CoPc and CoPc-P4VP modified electrodes (Fig. 4). Electrolyses were conducted for 2 h in a gas-tight, two-compartment electrolysis cell under a CO2 atmosphere in a constantly stirred, CO2 saturated, 0.1 M aqueous NaH2PO4 buffer solution at pH 4.7. The results of these experiments are summarized in Table 1. Nearly four times as much charge was passed using CoPc-P4VP modified electrodes compared to CoPc modified electrodes. Over the 2 h experiments, current densities averaging 0.62 mA cm−2 and 2.0 mA cm−2 were observed for CoPc and CoPc-P4VP, respectively. The only products observed for both films were CO and H2. No liquid products were detected for either CoPc or CoPc-P4VP within our detection limits (∼10 μM). Faradaic efficiencies (ε) for CO are 36% for CoPc and 89% for CoPc-P4VP. εH2 is 41% for CoPc and only 5% for CoPc-P4VP films. Turnover numbers (TON) for CO of 4500 and 34
000 were determined for CoPc and CoPc-P4VP, respectively. From these TONs, empirical turnover frequencies (TOFs) could be determined for the production of CO (Table 1). It is important to note that, because the total deposited catalyst coverage was used to determine TON and TOF values, these values are likely a lower limit of the actual values. While the previous study of CoPc immobilized in 100% P4VP did not report faradaic efficiency data for CoPc and CoPc-P4VP films, selectivity data is reported in the form of the ratio of CO to H2.33 For CoPc, the observed CO/H2 ratio of 0.9
:
1 is in good agreement with the previously reported ratio of ∼1.5
:
1. However, in our hands, H2 production by CoPc-P4VP is significantly reduced. The previous study reported a CO/H2 ratio of 4
:
1, while we observe a CO/H2 ratio of 19
:
1. This difference in selectivity could be due to a difference in P4VP membrane thickness—5 μL of the 1% w/v P4VP deposition solution to prepare the modified electrodes in this study whereas only 2 μL was used in the earlier report.
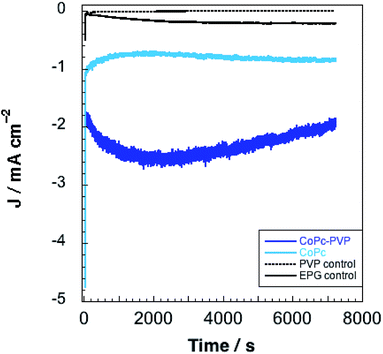 |
| Fig. 4 Representative electrolyses for CoPc, CoPc-P4VP, EPG, and EPG-P4VP conducted at −1.25 V vs. SCE. | |
Table 1 Results obtained from 2 h CPE experiments at −1.25 V vs. SCE for CoPc modified electrodes. The reported values are averages of measurements from at least three experiments with independently prepared electrodes. Errors are given as standard deviations except for those for εtotal which were calculated as standard errors
|
Charge/C |
ε
CO
|
TONCO (2 h) |
TOFCO (s−1) |
ε
H2
|
ε
total
|
CoPc
|
0.58 ± 0.24 |
36 ± 7% |
4.5 ± 2.4 × 103 |
0.6 ± 0.3 |
41 ± 8% |
77 ± 10% |
CoPc(py)
|
0.83 ± 0.48 |
68 ± 3% |
1.2 ± 0.7 × 104 |
1.6 ± 1.0 |
19 ± 5% |
87 ± 6% |
CoPc-P4VP
|
1.9 ± 0.20 |
89 ± 3% |
3.4 ± 0.4 × 104 |
4.8 ± 0.6 |
5 ± 1% |
94 ± 3% |
CoPc-P2VP
|
0.36 ± 0.08 |
73 ± 8% |
5.6 ± 1.8 × 103 |
0.8 ± 0.2 |
12 ± 3% |
85 ± 9% |
CoPc(py)-P2VP
|
1.76 ± 0.27 |
83 ± 5% |
3.0 ± 0.5 × 104 |
4.2 ± 0.7 |
6 ± 5% |
89 ± 7% |
A series of control experiments were conducted to ensure that the observed CO production was due to the electrocatalytic reduction of CO2 by CoPc. The results of these control experiments are provided in Tables S1–S3.† To summarize, potentiostatic electrolysis experiments were conducted with unmodified and P4VP coated EPG electrodes. For EPG electrodes under 1 atm CO2, the major product was hydrogen. For P4VP coated EPG electrodes under 1 atm CO2, hydrogen was also observed, but in much smaller amounts compared with the bare EPG electrodes due to the very low charge passed during the experiments. In both cases only very small amounts of CO could be observed (0.17 μmol), less than 2% of the amount of CO generated by CoPc-P4VP under identical conditions. Additionally, electrolyses were conducted with CoPc and CoPc-P4VP under an atmosphere of N2. As expected the only product observed was H2 for both systems.
Axial ligand effect
To investigate the influence of axial coordination on CO2RR activity of CoPc in the absence of the polymer film, electrodes modified with the pyridine coordinated catalyst, CoPc(py), were prepared using a deposition solution of CoPc in neat pyridine. A large excess of the axial ligand was employed in the deposition solutions to ensure the equilibrium would favor the axially coordinated species.49 RDEVs of the CoPc(py) films under an atmosphere of CO2, at 1600 rpm, display a positive shift in the onset potential of the catalytic wave, compared to CoPc, and a significant increase in the peak catalytic current over the parent complex, as shown in Fig. 3A. During 2 h electrolysis experiments, CoPc(py) films passed ∼1.5 times more charge than CoPc, with average current of 0.96 mA cm−2 (Fig. S6†). Faradaic efficiencies for CO with CoPc(py) were more than double the parent catalyst at 68%. Only 19% of the total charge passed went towards the production of H2 in the CoPc(py) film. No liquid products were detected. The TON for CO with the five-coordinate complex was around 12
000, with a TOFCO of 1.6 s−1.
Enhanced CO2RR performance has been observed with some heterogeneous catalysts after the addition of free pyridine to the electrolysis solution.50–52 CPE experiments were conducted with CoPc films in the presence of added pyridine and 2,6-lutidine to determine whether the presence of free ligand increases CO2RR activity. The results of these experiments are summarized in the ESI (Table S3†). Electrolyses preformed using CoPc modified electrodes with 0.05 mM added pyridine or 2,6-lutidine showed no significant change in the amount of charge passed or in the faradaic efficiencies for CO or H2 compared to CoPc alone. The concentration of added pyridine and 2,6-lutidine in these experiments is orders of magnitude higher than the concentration of pyridine that could be achieved from the complete dissociation of pyridine from CoPc(py) into solution (6.3 nM). Because no increase in activity or selectivity was observed with the addition of free pyridine, it appears that the axial ligand remains bound to CoPc in films of CoPc(py) during catalysis.
Polymer encapsulation
To separate the secondary and outer coordination sphere effects of the polymer membrane from the axial coordination effects, modified EPG electrodes were prepared with CoPc encapsulated in a poly-2-vinylpyridine (P2VP) membrane. Steric congestion around the pyridine nitrogens in P2VP should prevent coordination to CoPc.33 Though P2VP is not capable of coordinating, it should maintain the secondary and outer coordination sphere properties of P4VP. CoPc-P2VP films were deposited under the same conditions as described for the deposition of CoPc-P4VP.
RDEVs of CoPc-P2VP under CO2 show the onset of catalysis at potentials similar to CoPc, with peak currents lower than those of the parent catalyst (Fig. 3B). Measurements of CO2 catalysis showed that CoPc-P2VP films passed much less charge during the electrolyses than CoPc-P4VP, and the average current density was 0.39 mA cm−2. However, CoPc-P2VP operated with εCO = 73%, much higher than CoPc, and εH2 for CoPc-P2VP was only 12%. Compared to CoPc, CoPc-P2VP showed practically identical TONCO and TOFCO during the 2 h electrolyses. These experiments indicate that while the P2VP support does not increase the activity of CoPc for CO2 reduction, it does suppress HER.
Electrodes modified with CoPc(py) dispersed in a P2VP membrane (CoPc(py)-P2VP) were prepared to reintroduce the axial ligand to CoPc in the P2VP catalysts films. These films were deposited from a 19
:
1 DMF/pyridine solution containing 0.05 mM CoPc and 1% w/v P2VP. Incorporating a more active, five-coordinate CoPc catalyst in the P2VP film increased CO2RR activity by nearly an order of magnitude. The onset potential of CO2RR catalysis in RDEVs of CoPc(py)-P2VP occurred at nearly the same potential as CoPc-P4VP, and the peak current was much larger than CoPc-P2VP (Fig. 3B). Average current densities of 1.9 mA cm−2 were observed for the CoPc(py)-P2VP system, which are nearly the same as for CoPc encapsulated in P4VP. The total charge passed in bulk electrolyses increased to 1.7 C for CoPc(py)-P2VP from 0.36 C with CoPc-P2VP. Faradaic efficiency for CO was increased from 73% without pyridine to 83%, and εH2 decreased to 6%. By reintroducing an axial ligand to CoPc in P2VP the CO2RR performance was restored to the level observed for CoPc-P4VP.
Discussion
In agreement with previous reports, the encapsulation of CoPc in P4VP results in a dramatic increase of activity and selectivity for CO2RR over free CoPc. The present study examines the activities and product distributions of these catalyst films in greater detail in an effort to better understand the underlying cause of this phenomenon. By itself, CoPc is an unremarkable CO2RR catalyst that displays poor selectivity and relatively low activity for the reduction of CO2 over proton reduction. Once immobilized in P4VP, the selectivity for CO production jumps to nearly 90% with close to an order of magnitude increase in the TONCO. Under the conditions described herein, CoPc-P4VP displays an even higher selectivity for CO production over HER than has been previously reported.33 In fact, CoPc-P4VP is among the most active and selective molecular CO2 reduction catalysts yet studied in aqueous solutions (Table 2). Additionally, at −0.73 V vs. RHE, or a 0.61 V overpotential for the production of CO, the high activity and selectivity are notable, even in comparison to heterogeneous catalysts like copper, which at this potential non-selectively produces CO and formate with lower faradaic efficiencies along with a large amount of H2 under similar conditions,1–6 and gold, which is among the most active and selective polycrystalline metal catalysts for the reduction of CO2 to CO.5,53,54 It is clear that the P4VP membrane alters the chemical environment of CoPc in a way that promotes the reduction of CO2. We hypothesize that both the ability of P4VP to coordinate CoPc, and the secondary and outer coordination sphere effects that arise from the partial protonation of free pyridine residues throughout the polymer film are the major contributors to the increased activity, as illustrated in Fig. 5. Though polymer encapsulation is a common way of immobilizing molecular catalysts onto an electrode surface,19,41,55–64 the use of a polymer membrane that offers this combination of properties is rare.25,39,48,56,65–69
Table 2 A comparison of reported molecular CO2RR electrocatalysts which display high activity and/or selectivity in aqueous media to CoPc-P4VP and CoPc(py)-P2VP
Catalyst |
Activity/mA cm−2 |
V vs. RHE |
pH |
Products (ε) |
TOF/s−1 |
Ref. |
These TOF values were recalculated from the literature report using the total loading of catalyst cast onto the surface, as opposed to the amount detected by CV. We believe using the total amount of catalyst deposited provides a more accurate comparison to other reported values in the literature.
Reported TOF values based on the electroactive surface coverage of the catalyst.
Solution phase catalyst at 2.0 mg mL−1 concentration.
No time information was provided for the electrolysis in the report, so activity and TOF could not be calculated for the electrolysis. However, the reported Koutecky–Levich analysis in the manuscript yields a TOF of 5.2 s−1.
Estimated pH of 0.1 M NaClO4 saturated with CO2.
No empirical TOF based on electrolysis data was provided. However, the authors did report a TOF value calculated from CV data of 7.3 s¬1.
|
CoPc-P4VP
|
2.0 ± 0.2 |
−0.73 |
4.7 |
CO (89 ± 3%), H2 (5 ± 1%) |
CO: 4.8 |
This study |
CoPc(py)-P2VP
|
1.9 ± 0.2 |
−0.73 |
4.7 |
CO (83 ± 5%), H2 (6 ± 5%) |
CO: 4.2 |
This study |
CoPc-(90% P4VP, 10% polystyrene)/BPG |
NR |
−0.70 |
4.4 |
CO (71.6%), H2 (21.0%) |
CO: 3.1a (EA 41)b |
34
|
CoPc-(90% P4VP, 10% polystyrene)/BPG |
NR |
−0.66 |
6.8 |
CO (77.2%), H2 (16.6%) |
CO: 2.9a (EA 51)b |
34
|
COF-367-Co |
∼3.5 |
−0.67 |
7.3 |
CO (91%), H2 (20%) |
CO: 0.05a (EA 0.5)b |
37
|
COF-367-Co(1%) |
∼0.5 |
−0.67 |
7.3 |
CO (48%), H2 (51%) |
CO: 0.2a (EA 2.6)b |
37
|
[Mn(bpy(tBu)2)(CO)3Br]/Nafion/MWCNT |
0.2 |
−0.75 |
7 |
CO (46%), H2 (44%) |
CO: 0.0005 |
38
|
Ni(cyclam)-PALAc |
NA |
−0.17 |
8 |
CO (92%) |
NA |
39
|
Poly(Cr(vinylterpy)2) |
NRd |
−0.52 |
5.8e |
HCHO (87%) |
NRd |
40
|
Re[(bpy)(CO3)Br]/Nafion |
0.002 |
−0.65 |
7 |
HCO2H (48%), CO (16.5%), H2 (39%) |
CO: 0.002, HCO2H: 0.006 |
41
|
Co(Ch)/MWCNT |
NR |
−0.83 |
4.6 |
CO (89%) |
CO: 0.04 |
42
|
Ir-Pincer (2MeCN) |
0.60 |
−1.0 |
6.95 |
HCOOH (93%), H2 (7%) |
NRf |
43
|
Ni(cyclam) |
0.64–0.97 |
−0.67 |
5 |
CO (84 ± 4%) |
NA |
44
|
Ni(MTC) |
0.64–0.97 |
−0.67 |
5 |
CO (88 ± 7%) |
NA |
44
|
Ni(MCC) |
0.64–0.97 |
−0.67 |
5 |
CO (92 ± 2%) |
NA |
44
|
Ni(HTC) |
0.64–0.97 |
−0.67 |
5 |
CO (88 ± 7%) |
NA |
44
|
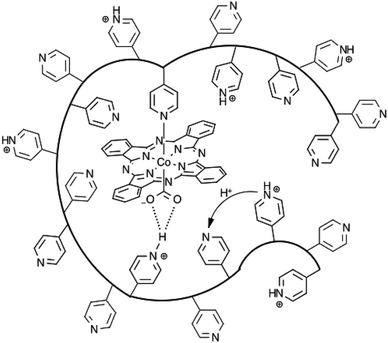 |
| Fig. 5 An illustration of CoPc immobilized in P4VP. Three properties of the polymer membrane which are suggested to be important to the activity and selectivity of CoPc-PVP are illustrated. Pyridine residues that can coordinate to CoPc, the ability for uncoordinated pyridine residues to act as proton relays, and the hydrogen bonding interactions that may occur between protonated pyridines and activated CO2. | |
Porphyrin and phthalocyanine catalysts often display enhanced catalytic activity upon the coordination of an axial ligand.26,28,70–72 The ability of P4VP to act as an axial ligand contributes to the enhanced CO2RR activity and selectivity of CoPc-P4VP modified electrodes. Other than in a P4VP film, the only previous study that suggests an axial ligand effect on CO2RR selectivity with CoPc comes from an IR spectroelectrochemical study which suggests that CoPc films deposited from neat pyridine are more selective for CO2RR over HER than films deposited from THF.73 The enhanced activity of CoPc(py) shows that the coordinating ligand effect is responsible for the increases in the rate of CO2 reduction. As shown in Fig. 6, axial coordination raises the energy of the cobalt dz2 orbital. When the metal center is reduced to Co(I), filling the dz2 orbital, the metal becomes a stronger nucleophile, and is better able to bind and activate the Lewis acidic carbon of CO2. The significant decrease in εH2 with CoPc(py) compared to CoPc may be attributable to the CO2 binding outcompeting proton reduction. This also suggests that CO2 reduction takes place via a CO2 binding event rather than the formation of a cobalt hydride that then goes on to react with CO2,26,34 as the formation of such a hydride would presumably also be the first step in HER.
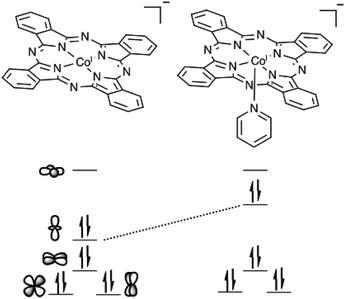 |
| Fig. 6 Relative energies of the cobalt d orbitals in the 1 e− reduced forms of CoPc and CoPc(py). Shown is the energy increase of the cobalt dz2 orbital that results the coordination of the axial pyridine. | |
The primary and outer coordination spheres of enzyme active sites are specially adapted to efficiently perform difficult catalytic transformations for the production of a single desired product. Protein film voltammetry has been used to demonstrate that enzymes adsorbed on electrode surfaces can perform electrocatalytic reactions, including CO2RR, with very high TON,74,75 although low surface coverages limit the overall activity. These studies have also established the importance of electron and proton relays in these reactions.76–78 In molecular systems, the importance of the hydrogen bonding in the secondary coordination sphere to stabilize reactive intermediates has been demonstrated by several groups.79–85 In particular, second sphere proton relays incorporated into a ligand scaffold can lead to high electrocatalytic activity in reactions that require the delivery of multiple protons.86–89 The P4VP membrane imbues many of these secondary coordination sphere effects to CoPc without the need for synthetically challenging ligand modifications.
While CoPc-P2VP showed virtually no increase in TON for CO over free CoPc, HER activity was greatly diminished. Combined with the decrease in HER performance displayed by CoPc-P4VP under N2 and in the EPG-P4VP control experiments, it is clear that the polymer film helps to suppress proton reduction pathways. It may be that limited diffusion of water through the film is the reason for the lower activity for HER. In the case of polymer free CoPc modified electrodes, the catalyst is directly exposed to solution where there is a large local concentration of protons. In the polymer immobilized systems, the catalyst is not exposed to the solvent directly and the permeability of water through the PVP membrane at this pH is limited, so the main source of available protons in the polymer film is likely from protonated pyridine residues.33,35,36 At pH 4.7 approximately 20% of the pyridine residues in the film are protonated.33 It is reasonable to conclude that increase in selectivity for CO over H2 with CoPc-P2VP compared to CoPc alone could be due to a weak acid effect from the protonated pyridine residues.90 While these pyridinium residues may be acidic enough to act as a proton donor to activated CO2 intermediates, they may also be basic enough to suppress HER activity. The selectivity for CO with CoPc-P4VP is much higher than with CoPc-P2VP. This suggests that in addition to the outer sphere weak acid effect, the primary sphere effect of axial coordination on the selectivity for CO2RR over HER, demonstrated by CoPc(py) is also an important factor for this catalytic selectivity.
Beyond limiting HER activity, the polymer membrane effects additional CO2RR rate enhancements, but only for the more active five-coordinate CoPc catalysts CoPc-P4VP and CoPc(py)-P2VP. Protonated pyridine residues in close proximity to the CoPc(L) catalyst active site (L = py, P4VP) may be able to stabilize the [(L)Co(II)Pc-CO2]− intermediate through hydrogen bonding interactions, and may increase the rate of proton transfer to the activated [(L)Co(II)Pc-CO2]− complex (Fig. 7). Again though, these secondary coordination sphere effects only result in rate enhancements with the five-coordinate CoPc(L) catalysts. This is demonstrated by comparing the observed TOFCO of CoPc-P2VP and CoPc(py)-P2VP to the TOFCO of the catalysts without the polymer film. No enhancement of CO2RR activity was observed when the four-coordinate CoPc was immobilized in the P2VP membrane compared to the catalyst outside of the polymer membrane. Alternatively, a large increase in TOFCO was observed for CoPc(py)-P2VP compared to polymer free films of CoPc(py). The chemical environment around the catalyst active sites in CoPc-P2VP and CoPc(py)-P2VP films is likely unaffected by the presence of the axial pyridine, yet only in the case of CoPc(py)-P2VP does being in this chemical environment lead to increased catalytic activity. A plausible explanation for this phenomenon is that the presence of the axial ligand may change the rate limiting step of CO2 reduction from the formation of Co(II)Pc-CO2− with the four-coordinate catalyst, to the subsequent proton transfer steps in the case of the five-coordinate catalyst. If this is the case, the secondary coordination sphere effects would have no influence of the rate of CO2 reduction by CoPc, but would result in the dramatic increases in CO2RR activity observed for the more active CoPc(L) catalysts.
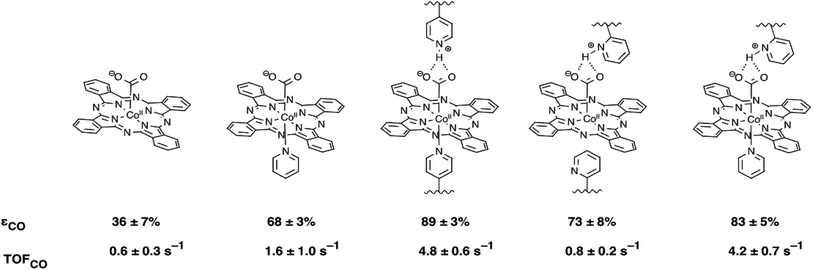 |
| Fig. 7 Proposed activated CO2 complexes and secondary coordination sphere interaction for each catalyst film studied. Also shown are the faradaic efficiencies and turnover frequencies for each catalyst system determined from CPE measurements. | |
Conclusions
Immobilization of cobalt phthalocyanine in poly-4-vinylpyridine dramatically improves its activity as a catalyst for the reduction of CO2 to CO. The polymer membrane slows the competing HER catalytic pathway while also increasing rate of CO2RR compared to the polymer free catalyst. It is clear from the results obtained for CoPc(py) and CoPc-P2VP that neither the primary, or secondary and outer coordination sphere effects alone are responsible for the large increases in CO2RR activity. These effects must be combined, as is the case in CoPc-P4VP and CoPc(py)-P2VP, to produce a CoPc CO2RR catalyst that is highly active and selective. In this way CoPc-P4VP and CoPc(py)-P2VP mimic enzymes, where all aspects of the catalyst chemical environment are critical to the function of the system overall.
We propose that this electrode-modification strategy using functional polymer membranes can be applied more generally to the field of CO2 reduction catalysis. The ability of PVP films to suppress HER without inhibiting CO2RR is in itself of importance considering that the cogeneration of H2 by CO2 reduction catalysts, both molecular and heterogeneous, in aqueous media limits CO2RR product selectivity. Additionally, the incorporation of groups other than pyridine in the polymer chain could allow us to engineer the chemical environment around catalyst active sites in a facile way, without the need for complicated ligand synthesis. Each component of these catalyst films could be selected based on its ability to maximize the efficiency and selectivity of the system as a whole. This approach could lead to the development of new highly active catalyst films which are not only selective for a single highly reduced product, but also cost effective and easily prepared.
Experimental
Materials
Ultrapure water (18.2 MΩ cm resistivity) was purified with a Thermo Scientific Barnstead Nanopure water purification system. Carbon dioxide (CO2, Alphagaz–1 grade, 99.99%) and helium (He, Alphagaz–1 grade, 99.999%) were purchased from Air–Liquide and used as received. Nitrogen (N2) was boil-off gas from a liquid nitrogen source and used without further purification. CoPc (97%) was obtained from Alfa Aesar and used as received. P4VP (average Mw ∼ 160
000) and P2VP (average Mw ∼ 159
000) were purchased from Sigma Aldrich and used as received. N,N-Dimethylformamide (DMF, ACS grade) was purchased from VWR. Pyridine (ACS grade) was purchased from Alfa Aesar. Deuterium oxide (D2O, 99.9%) was purchased from Cambridge Isotope Labs, Inc.
Preparation of modified electrodes
All catalyst films were prepared from deposition solutions containing 0.05 mM CoPc. The parent CoPc was deposited from solutions in DMF, as were the polymer encapsulated CoPc films. Deposition solutions for polymer-encapsulated CoPc films contained 1% w/v of the polymer. For films of CoPc(py), neat pyridine was used as the solvent in place of DMF. Modified electrodes were prepared by evaporation of 5 μL of the deposition solution on an edge-plane graphite disc electrode (5 mm diameter, effective electrode area: 0.13 cm2, Pine Research Instrumentation). For all modified electrodes used in this study, the coverage of deposited CoPc was 1.3 × 10−9 mol cm−2. Prior to catalyst deposition, the electrodes were cleaned by manually polishing the surface with 600 grit Carbimet SiC grinding paper (Buehler) followed by sonication in ultrapure water for 10 min.
Electrochemical methods
Experiments were conducted using a Bio-Logic SP200 potentiostat/galvanostat with a built-in electrochemical impedance spectroscopy (EIS) analyzer or a Bio-Logic VMP3 multichannel potentiostat/galvanostat with a built-in EIS analyzer. The modified working electrodes were mounted in a Pine Instrument Company E6-series ChangeDisk rotating disk electrode assembly in an MSR rotator. The auxiliary electrodes were carbon rods (99.999%, Strem), and the reference electrodes were commercial saturated calomel electrodes (SCE) (CH-Instruments) that were externally referenced to a solution of ferrocene monocarboxylic acid (Sigma-Aldrich) in a 0.2 M phosphate buffer at pH 7 (0.284 V vs. SCE).91 The supporting electrolyte solution was 0.1 M aqueous NaH2PO4 (BioUltra ≥ 99.0%, Sigma) with the pH adjusted to pH 5 by the addition of 1 M aqueous NaOH. When saturated with CO2 the pH of the electrolyte solution was measured to be 4.71. The pH of electrolyte solutions were measured with a VWR Symphony multiparameter meter with a Thermo Scientific Orion refillable Ag/AgCl pH electrode filled with Orion Ag/AgCl reference electrode filling solution. The pH meter was calibrated with a 4 point calibration curve at pH = 1.68, 4.00, 7.00, and 10.00. Data were recorded using the Bio-Logic EC-Lab software package.
Electrochemical measurements
Cyclic voltammetry (CV) and rotating disc electrode (RDE) experiments were conducted in a glass two-chamber U-cell. One chamber held the working and reference electrodes in ∼150 mL of solution, and the other chamber held the auxiliary electrode in ∼20 mL of solution. The two chambers were separated by a fine-porosity glass frit. Both chambers were purged with either N2 or CO2 for ∼20 min prior to each experiment and then blanketed with N2 or CO2 during data collection. CO2 and N2 were water-saturated by bubbling though gas-washing bottle filled with ultrapure water.
Controlled potential electrolyses
Experiments were conducted in a custom, two-chamber U-cell. The working and reference electrodes were held in a gas tight chamber with a total volume of either 81 or 91 mL and filled with solution to provide 41 mL of headspace. The second chamber held the auxiliary electrode in 15 mL of solution and was open to air. The two chambers were separated by a Nafion 117 cation exchange membrane (Aldrich). The chemically-modified working electrode was held in a Pine Instrument Company RDE internal hardware kit and mounted in a custom PEEK sleeve. Prior to each electrolysis experiment the cell was purged with CO2 for at least 1 h and then sealed under an atmosphere of CO2. All experiments were conducted at least three time with independently prepared electrodes. All values reported are the averages of these repetitions and errors are reported as standard deviations.
To allay the concern that the use of a carbon-rod auxiliary electrode with a Nafion cation exchange membrane might lead to the formation of formate that can cross over into the catholyte solution, and to validate the choice of experimental conditions, electrolyses were performed using platinum mesh electrode as the auxiliary and a Selemion AMV anion exchange membrane (AGC Engineering Co., Ltd). No difference in activity or product distribution was observed between electrolysis experiments using platinum mesh electrodes and Selemion ion exchange membranes compared to those using carbon rod electrodes and Nafion ion exchange membranes.
Product detection and quantification
Gaseous products (i.e. CO and H2) in the headspace were quantified using an Agilent Technologies 7890A Permanent Gas and Hydrogen Analyzer GC system with two analyzer channels. Using a valve system, column configuration, and method developed by Agilent Technologies, gases were separated so that H2 was detected on one channel using an N2 carrier gas, and all other gases were detected on the second channel using a He carrier gas. All gases were detected with a thermal conductivity detector (TCD), and chromatographs were analyzed using the Agilent OpenLAB CDS ChemStation software. A Pressure-Lok A–2 gas-tight analytical syringe (10 mL, Valco VICI Precision Sampling, Inc.) was used to collect 8 mL aliquots from the working electrode chamber headspace. Prior to each injection, the sample loop was purged with N2, then an aliquot was injected directly into the 6 mL sample loop. The presence of liquid products in the catholyte was investigated using Presat solvent suppression NMR spectroscopy on a 400 MHz Bruker cryoprobe spectrometer using 900 μL of the catholyte solution containing 100 μL D2O for signal locking, and 3.2 mM DMF as an internal standard.3 Faradaic efficiencies were determined by dividing the total moles products for each product by the moles of electrons calculated from the amount of charge passed during the controlled-potential electrolysis measurements, accounting for the number of electrons required to produce each product.
Acknowledgements
We are very grateful to Professor Harry Gray and Dr Xenia Amashukeli for helpful discussions and enthusiastic input during the preparation of this manuscript. We acknowledge additional useful discussions with Dr Ivonne M. Ferrer regarding the initial acquisition and analysis of controlled-potential electrolysis data. This work was supported by the California Energy Commission Agreement 500-11-023 “Accelerating the Development of Liquid Fuels Directly from Sunlight”.
Notes and references
- R. J. Lim, M. Xie, M. A. Sk, J.-M. Lee, A. Fisher, X. Wang and K. H. Lim, Catal. Today, 2013, 233, 169–180 CrossRef.
- K. J. P. Schouten, E. Pérez Gallent and M. T. M. Koper, ACS Catal., 2013, 3, 1292–1295 CrossRef CAS.
- K. P. Kuhl, E. R. Cave, D. N. Abram and T. F. Jaramillo, Energy Environ. Sci., 2012, 5, 7050–7059 CAS.
- M. Gattrell, N. Gupta and A. Co, J. Electroanal. Chem., 2006, 594, 1–19 CrossRef CAS.
- K. P. Kuhl, T. Hatsukade, E. R. Cave, D. N. Abram, J. Kibsgaard and T. F. Jaramillo, J. Am. Chem. Soc., 2014, 136, 14107–14113 CrossRef CAS PubMed.
- Y. Hori, A. Murata and R. Takahashi, J. Chem. Soc., Faraday Trans. 1, 1989, 85, 2309–2326 RSC.
- C. W. Machan, C. J. Stanton III, J. E. Vandezande, G. F. Majetich, H. F. Schaefer III, C. P. Kubiak and J. Agarwal, Inorg. Chem., 2015, 54, 8849–8856 CrossRef CAS PubMed.
- D. C. Lacy, C. C. L. McCrory and J. C. Peters, Inorg. Chem., 2014, 53, 4980–4988 CrossRef CAS PubMed.
- I. Hod, M. D. Sampson, P. Deria, C. P. Kubiak, O. K. Farha and J. T. Hupp, ACS Catal., 2015, 6302–6309 CrossRef CAS.
- N. Elgrishi, M. B. Chambers and M. Fontecave, Chem. Sci., 2015, 6, 2522–2531 RSC.
- J. D. Blakemore, A. Gupta, J. J. Warren, B. S. Brunschwig and H. B. Gray, J. Am. Chem. Soc., 2013, 135, 18288–18291 CrossRef CAS PubMed.
- M. D. Sampson, J. D. Froehlich, J. M. Smieja, E. E. Benson, I. D. Sharp and C. P. Kubiak, Energy Environ. Sci., 2013, 6, 3748–3755 CAS.
- C. Costentin, S. Drouet, G. Passard, M. Robert and J.-M. Savéant, J. Am. Chem. Soc., 2013, 135, 9023–9031 CrossRef CAS PubMed.
- J. L. Inglis, B. J. MacLean, M. T. Pryce and J. G. Vos, Coord. Chem. Rev., 2012, 256, 2571–2600 CrossRef CAS.
- M. Bourrez, F. Molton, S. Chardon-Noblat and A. Deronzier, Angew. Chem., Int. Ed., 2011, 50, 9903–9906 CrossRef CAS PubMed.
- E. E. Benson, C. P. Kubiak, A. J. Sathrum and J. M. Smieja, Chem. Soc. Rev., 2008, 38, 89 RSC.
- M. Isaacs, J. C. Canales, M. J. Aguirre, G. Estiu, F. Caruso, G. Ferraudi and J. Costamagna, Inorg. Chim. Acta, 2002, 339, 224–232 CrossRef CAS.
- W. Leitner, Angew. Chem., Int. Ed., 1995, 34, 2207–2221 CrossRef CAS.
- J. P. Collin and J. P. Sauvage, Coord. Chem. Rev., 1989, 93, 245–268 CrossRef CAS.
- T. R. O'Toole, B. P. Sullivan, M. R. M. Bruce, L. D. Margerum, R. W. Murray and T. J. Meyer, J. Electroanal. Chem., 1989, 259, 217–239 CrossRef.
- M. Hammouche, D. Lexa, J. M. Savéant and M. Momenteau, J. Electroanal. Chem., 1988, 249, 347–351 CrossRef CAS.
- M. Beley, J. P. Collin, R. Ruppert and J. P. Sauvage, J. Am. Chem. Soc., 1986, 108, 7461–7467 CrossRef CAS PubMed.
- C. R. Cabrera and H. D. Abruña, J. Electroanal. Chem., 1986, 209, 101–107 CrossRef CAS.
- M. Isaacs, F. Armijo, G. Ramírez, E. Trollund, S. R. Biaggio, J. Costamagna and M. J. Aguirre, J. Mol. Catal. A: Chem., 2005, 229, 249–257 CrossRef CAS.
- T. Abe, H. Imaya, T. Yoshida, S. Tokita, D. Schlettwein, D. Wöhrle and M. Kaneko, J. Porphyrins Phthalocyanines, 1997, 01, 315–321 CrossRef CAS.
- H. Aga, A. Aramata and Y. Hisaeda, J. Electroanal. Chem., 1997, 437, 111–118 CrossRef CAS.
- J. H. Zagal, Coord. Chem. Rev., 1992, 119, 89–136 CrossRef CAS.
- T. Atoguchi, A. Aramata, A. Kazusaka and M. Enyo, J. Electroanal. Chem., 1991, 318, 309–320 CrossRef CAS.
- K. Kusuda, R. Ishihara, H. Yamaguchi and I. Izumi, Electrochim. Acta, 1986, 31, 657–663 CrossRef CAS.
- S. Kapusta and N. Hackerman, J. Electrochem. Soc., 1984, 131, 1511–1514 CrossRef CAS.
- C. M. Lieber and N. S. Lewis, J. Am. Chem. Soc., 1984, 106, 5033–5034 CrossRef CAS.
- S. Meshitsuka, M. Ichikawa and K. Tamaru, J. Chem. Soc., Chem. Commun., 1974, 158–159 RSC.
- T. Abe, T. Yoshida, S. Tokita, F. Taguchi, H. Imaya and M. Kaneko, J. Electroanal. Chem., 1996, 412, 125–132 CrossRef.
- T. Yoshida, K. Kamato, M. Tsukamoto, T. Iida, D. Schlettwein, D. Wöhrle and M. Kaneko, J. Electroanal. Chem., 1995, 385, 209–225 CrossRef.
- G. J. Samuels and T. J. Meyer, J. Am. Chem. Soc., 1981, 103, 307–312 CrossRef CAS.
- J. M. Calvert and T. J. Meyer, Inorg. Chem., 1982, 21, 3978–3989 CrossRef CAS.
- S. Lin, C. S. Diercks, Y.-B. Zhang, N. Kornienko, E. M. Nichols, Y. Zhao, A. R. Paris, D. Kim, P. Yang, O. M. Yaghi and C. J. Chang, Science, 2015, 349, 1208–1213 CrossRef CAS PubMed.
- J. J. Walsh, C. L. Smith, G. Neri, G. F. S. Whitehead, C. M. Robertson and A. J. Cowan, Faraday Discuss., 2015, 183, 147–160 RSC.
- D. Saravanakumar, J. Song, N. Jung, H. Jirimali and W. Shin, ChemSusChem, 2012, 5, 634–636 CrossRef CAS PubMed.
- J. A. Ramos Sende, C. R. Arana, L. Hernandez, K. T. Potts, M. Keshevarz-K and H. D. Abruña, Inorg. Chem., 1995, 34, 3339–3348 CrossRef CAS.
- T. Yoshida, K. Tsutsumida, S. Teratani, K. Yasufuku and M. Kaneko, J. Chem. Soc., Chem. Commun., 1993, 631–633 RSC.
- S. Aoi, K. Mase, K. Ohkubo and S. Fukuzumi, Chem. Commun., 2015, 51, 10226–10228 RSC.
- P. Kang, T. J. Meyer and M. Brookhart, Chem. Sci., 2013, 4, 3497–3502 RSC.
- J. Schneider, H. Jia, K. Kobiro, D. E. Cabelli, J. T. Muckerman and E. Fujita, Energy Environ. Sci., 2012, 5, 9502–9510 CAS.
- A. Koca, M. Kasimsener, M. Kocak and A. Gul, Int. J. Hydrogen Energy, 2006, 31, 2211–2216 CrossRef CAS.
- O. Osmanbas, A. Koca, M. Kandaz and F. Karaca, Int. J. Hydrogen Energy, 2008, 33, 3281–3288 CrossRef CAS.
- A. Koca, A. Kalkan and Z. A. Bayır, Electrochim. Acta, 2011, 56, 5513–5525 CrossRef CAS.
- F. Zhao, J. Zhang, T. Abe, D. Wöhrle and M. Kaneko, J. Mol. Catal. A: Chem., 1999, 145, 245–256 CrossRef CAS.
- T. Nyokong, Polyhedron, 1995, 14, 2325–2329 CrossRef CAS.
- E. E. B. Cole, M. F. Baruch, R. P. L'Esperance, M. T. Kelly, P. S. Lakkaraju, E. L. Zeitler and A. B. Bocarsly, Top. Catal., 2014, 58, 15–22 CrossRef.
- A. J. Morris, R. T. McGibbon and A. B. Bocarsly, ChemSusChem, 2011, 4, 191–196 CrossRef CAS PubMed.
- E. B. Cole, P. S. Lakkaraju, D. M. Rampulla, A. J. Morris, E. Abelev and A. B. Bocarsly, J. Am. Chem. Soc., 2010, 132, 11539–11551 CrossRef PubMed.
- Y. Hori, A. Murata, K. Kikuchi and S. Suzuki, J. Chem. Soc., Chem. Commun., 1987, 728–729 RSC.
- Y. Chen, C. W. Li and M. W. Kanan, J. Am. Chem. Soc., 2012, 134, 19969–19972 CrossRef CAS PubMed.
- J. J. Walsh, G. Neri, C. L. Smith and A. J. Cowan, Chem. Commun., 2014, 50, 12698–12701 RSC.
- T. Abe and M. Kaneko, Prog. Poly. Sci., 2003, 28, 1441–1488 CrossRef CAS.
- T. Hirose, Y. Maeno and Y. Himeda, J. Mol. Catal. A: Chem., 2003, 193, 27–32 CrossRef CAS.
- A. Jarzebinska, P. Rowinski, I. Zawisza, R. Bilewicz, L. Siegfried and T. Kaden, Anal. Chim. Acta, 1999, 396, 1–12 CrossRef CAS.
- T. Yoshida, T. Iida, T. Shirasagi, R.-J. Lin and M. Kaneko, J. Electroanal. Chem., 1993, 344, 355–362 CrossRef CAS.
- B. A. Retamal, M. E. Vaschetto and J. Zagal, J. Electroanal. Chem., 1997, 431, 1–5 CrossRef CAS.
- R. M. Latonen, X. J. Wang, P. Sjoberg-Eerola, J. E. Eriksson, M. Bergelin and J. Bobacka, Electrochim. Acta, 2012, 68, 25–31 CrossRef CAS.
- H. D. Abruña, Coord. Chem. Rev., 1988, 86, 135–189 CrossRef.
- A. R. Guadalupe and H. D. Abruña, Anal. Chem., 1985, 57, 142–149 CrossRef CAS.
- W. J. Albery and A. R. Hillman, Annu. Rep. Prog. Chem., Sect. C: Phys. Chem., 1981, 78, 377 RSC.
- R. D. L. Smith and P. G. Pickup, Electrochem. Commun., 2010, 12, 1749–1751 CrossRef CAS.
- A. Zhang, W. Zhang, J. Lu, G. G. Wallace and J. Chen, Electrochem. Solid-State Lett., 2009, 12, E17–E19 CrossRef CAS.
- J. Chlistunoff and J.-M. Sansiñena, J. Phys. Chem. C, 2014, 118, 19139–19149 CAS.
- R. Wang, W. Jiao and B. Gao, Appl. Surf. Sci., 2009, 255, 7766–7772 CrossRef CAS.
- F. Zhao, J. Zhang, D. Wöhrle and M. Kaneko, J. Porphyrins Phthalocyanines, 2000, 4, 31–36 CrossRef CAS.
- M. Xu, C. Li, H. Ren, L. Ding, K. Xu and J. Geng, J. Mol. Catal. A: Chem., 2014, 390, 69–75 CrossRef CAS.
- S. Samanta, P. K. Das, S. Chatterjee and A. Dey, J. Porphyrins Phthalocyanines, 2015, 19, 92–108 CrossRef CAS.
- R. Cao, R. Thapa, H. Kim, X. Xu, M. G. Kim and Q. Li, Nat. Commun., 2013, 4, 2076 Search PubMed.
- P. A. Christensen, A. Hamnett and A. V. G. Muir, J. Electroanal. Chem., 1988, 241, 361–371 CrossRef CAS.
- M. Can, F. A. Armstrong and S. W. Ragsdale, Chem. Rev., 2014, 114, 4149–4174 CrossRef CAS PubMed.
- V. C. C. Wang, M. Can, E. Pierce, S. W. Ragsdale and F. A. Armstrong, J. Am. Chem. Soc., 2013, 135, 2198–2206 CrossRef CAS PubMed.
- J. Hirst, J. L. C. Duff, G. N. L. Jameson, M. A. Kemper, B. K. Burgess and F. A. Armstrong, J. Am. Chem. Soc., 1998, 120, 7085–7094 CrossRef CAS.
- C. Léger, S. J. Elliott, K. R. Hoke, L. J. C. Jeuken, A. A. K. Jones and F. A. Armstrong, Biochemistry, 2003, 42, 8653–8662 CrossRef PubMed.
- F. A. Armstrong, H. A. Heering and J. Hirst, Chem. Soc. Rev., 1997, 26, 169–179 RSC.
- R. L. Shook and A. S. Borovik, Inorg. Chem., 2010, 49, 3646–3660 CrossRef CAS PubMed.
- S. A. Cook and A. S. Borovik, Acc. Chem. Res., 2015, 150716113856002 Search PubMed.
- C. K. Chang, Y. Liang, G. Aviles and S.-M. Peng, J. Am. Chem. Soc., 2002, 117, 4191–4192 CrossRef.
- C.-Y. Yeh, A. Christopher, J. Chang and D. G. Nocera, J. Am. Chem. Soc., 2001, 123, 1513–1514 CrossRef CAS PubMed.
- G. E. Wuenschell, C. Tetreau, D. Lavalette and C. A. Reed, J. Am. Chem. Soc., 1992, 114, 3346–3355 CrossRef CAS.
- C. M. Moore and N. K. Szymczak, Chem. Sci., 2015, 6, 3373–3377 RSC.
- E. M. Matson, Y. J. Park and A. R. Fout, J. Am. Chem. Soc., 2014, 136, 17398–17401 CrossRef CAS PubMed.
- J. T. bays, N. Priyadarshani, M. S. Jeletic, E. B. Hulley, D. L. Miller, J. C. Linehan and W. J. Shaw, ACS Catal., 2014, 4, 3663–3670 CrossRef CAS.
- A. D. Wilson, R. H. Newell, M. J. McNevin, J. T. Muckerman, A. M. Rakowski DuBois and D. L. DuBois, J. Am. Chem. Soc., 2005, 128, 358–366 CrossRef PubMed.
- G. M. Jacobsen, J. Y. Yang, B. Twamley, A. D. Wilson, R. M. Bullock, M. R. DuBois and D. L. DuBois, Energy Environ. Sci., 2008, 1, 167–174 CAS.
- M. L. Helm, M. P. Stewart, R. M. Bullock, M. R. DuBois and D. L. DuBois, Science, 2011, 333, 863–866 CrossRef CAS PubMed.
- C. Riplinger, M. D. Sampson, A. M. Ritzmann, C. P. Kubiak and E. A. Carter, J. Am. Chem. Soc., 2014, 136, 16285–16298 CrossRef CAS PubMed.
- E. Liaudet, F. Battaglini and E. J. Calvo, J. Electroanal. Chem., 1990, 293, 55–68 CrossRef CAS.
Footnotes |
† Electronic supplementary information (ESI) available: Representative cyclic voltammograms of modified electrodes, representative current–time plots from controlled potential electrolyses, and tabulated results from control experiments. See DOI: 10.1039/c5sc04015a |
‡ Present address: Department of Chemistry, University of Michigan, Ann Arbor, MI, 48109 (USA). |
|
This journal is © The Royal Society of Chemistry 2016 |
Click here to see how this site uses Cookies. View our privacy policy here.