DOI:
10.1039/C5SC04476F
(Edge Article)
Chem. Sci., 2016,
7, 1904-1909
Chemo- and regioselective oxygenation of C(sp3)–H bonds in aliphatic alcohols using a covalently bound directing activator and atmospheric oxygen†
Received
21st November 2015
, Accepted 26th November 2015
First published on 27th November 2015
Abstract
Chemically reactive directing groups (directing activators) represent a promising strategy for mild and regioselective C(sp3)–H functionalization. The use of a radical N-oxyl directing activator promoted the aerobic oxygenation of benzylic, propargylic, tertiary, and unactivated acyclic methylene C(sp3)–H bonds in aliphatic alcohols with γ- (or δ-) selectivity under mild conditions (room temperature to 50 °C). The reaction was unaffected by the presence of various oxidation-sensitive functional groups, which proved to be problematic in previously reported studies on the oxidation of C(sp3)–H bonds. Structural modifications on the directing activator altered the regioselectivity, and thus provided an ultra-remote aerobic C(sp3)–H oxygenation. The observed reactivity and regioselectivity could be rationalized in terms of the intramolecular conformational accessibility of the N-oxyl radical and the electronic characteristics of C(sp3)–H bonds.
Introduction
The functionalization of unactivated C–H bonds is a research area that is currently being investigated intensively. Especially for the streamlined synthesis of complex drug lead molecules, containing a multitude of C(sp3)–H bonds, a controlled functionalization of these bonds can be a powerful synthetic tool.1 In order to apply C(sp3)–H functionalizations to drug lead syntheses, two key features are necessary: (1) mild and clean reaction conditions to assure functional group tolerance,2 and (2) site-selectivity due to the commonly encountered presence of multiple C–H bonds.3
We were thus interested in a site-selective oxygenation of alcohols that is able to target C(sp3)–H bonds located remotely from the hydroxy group,4 using clean and abundant aerobic oxygen (O2) as the oxidant.5 This unprecedented reaction pattern should provide conceptually improved synthetic routes to various biologically active drug leads, containing multiple functional groups based on oxygen. Ideally, these routes should exhibit a high redox economy,6 and generate a minimum of potentially toxic waste. The three main obstacles to overcome in the development of such reactions are: (1) the applicability to ubiquitous, but unreactive acyclic methylene C(sp3)–H bonds;7 (2) the conversion of such unreactive C(sp3)–H bonds at a specific position, while simultaneously overriding the innately higher reactivity of the C–H bonds at α-position with respect to the oxygen atom;8 and (3) the low efficiency of triplet O2 in initiation of the oxygenation reaction.
Herein, we describe the use of a chemically reactive directing group or “directing activator” (DA)9,10 in order to circumvent the aforementioned obstacles, thus expecting to produce the entropically preferred unimolecular transition states leading to a mild and chemoselective cleavage of specific C–H bonds, including very challenging methylene C(sp3)–H bonds (Scheme 1-1).11
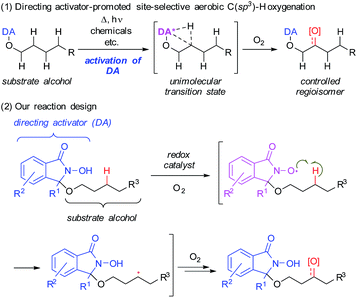 |
| Scheme 1 Chemo- and regioselective intramolecular oxidation of C(sp3)–H bonds in aliphatic alcohols using a novel directing activator. | |
Results and discussion
Optimization of reaction conditions
We devised a novel DA inspired by Ishii's N-hydroxyphthalimide (NHPI) chemistry (Scheme 1-2),12 which is based on an N-oxyl radical, generated from the N-hydroxyamide moiety of the DA in the presence of O2. This moiety is able to cleave C(sp3)–H bonds homolytically to produce a carbon radical. By covalently attaching the DA to the hydroxy group of substrate alcohols, this C(sp3)–H activation step should become an intramolecular process. Thus, it should be possible to selectively cleave specific C(sp3)–H bonds that can engage in suitable spatial contact with the in situ-generated N-oxyl radical of the DA.13 Subsequently, the thus generated carbon radical would be trapped by O2 to produce the corresponding oxygenated alcohols or ketones.14
Based on this reaction design, we began our investigation of aerobic C–H oxygenation by modifying the DA structure and performing a screening of oxygenation conditions using DA-bound alcohol substrates (Table 1). In order to attach covalently the substrate alcohols to the DA, one of the two imide carbonyl groups in NHPI was transformed into an aminal group, leading to an N-hydroxyisoindolinone structure. As the reactivity of N-oxyl radicals towards C–H cleavage follows their electron-deficiency,15 we tried to introduce electron-withdrawing groups and found that especially the introduction of a trifluoromethyl group at the 3-position of the DA (R1 = CF3) was effective. Then we screened suitable metal catalysts that could promote N-oxyl radical formation from the N-hydroxy group (see Scheme 5) using 1-butanol-derivative 1a as the substrate and 2,2,2-trifluoroethanol (TFE) as the solvent (Table 1, entries 1–11). We found that Co(OAc)2 (entry 6) and Mn(OAc)3·2H2O (entry 8) were effective. Although the α-C(sp3)–H bond adjacent to the ether oxygen atom is the innately more reactive site,8 it was the less reactive γ-C(sp3) atom that was predominantly oxygenated. Metal salts bearing counterions other than acetate (i.e., acac, NO3, halide, and OTf) showed very low reactivity.16 The reaction was cleaner with the combinational use of Co(OAc)2 and Mn(OAc)3·2H2O, and the desired C–H oxygenation product 2a was obtained in 67% NMR yield (entry 10). The catalyst loading could be reduced to 5 mol% of each metal without loss of efficiency (entry 11). A DA with a simple alkyl substitution (R1 = Et), instead of a CF3 group, did not show satisfactory performance (entry 12). Introduction of another CF3 moiety on the aromatic ring of the DA did not improve the result (entry 13). Other types of DA modification resulted in production of complex reaction mixtures.16
Table 1 Investigation of a metal catalyst and DA

|
Entry |
Metal (mol%) |
R1 |
R2 |
Yielda |
Yields were calculated from the 1H NMR spectra of crude reaction mixtures using 1,1,2,2-tetrachloroethane as an internal standard. Isolated yields are given in parentheses.
The reaction time was 18 h.
|
1 |
None |
CF3 |
H |
0 |
2 |
CuOAc (20) |
CF3 |
H |
0 |
3 |
Cu(OAc)2 (20) |
CF3 |
H |
0 |
4 |
Fe(OAc)2 (20) |
CF3 |
H |
0 |
5 |
Fe(OH)(OAc)2 (20) |
CF3 |
H |
0 |
6 |
Co(OAc)2 (20) |
CF3 |
H |
40 |
7 |
Mn(OAc)2 (20) |
CF3 |
H |
3 |
8 |
Mn(OAc)3·2H2O (20) |
CF3 |
H |
36 |
9 |
Co(OAc)2 (10) + Mn(OAc)2 (10) |
CF3 |
H |
16 |
10 |
Co(OAc)2 (10) + Mn(OAc)3·2H2O (10) |
CF3 |
H |
67 |
11 |
Co(OAc)2 (5) + Mn(OAc)3·2H2O (5) |
CF3 |
H |
67b(62) |
12 |
Co(OAc)2 (5) + Mn(OAc)3·2H2O (5) |
Et |
H |
0 |
13 |
Co(OAc)2 (5) + Mn(OAc)3·2H2O (5) |
CF3 |
CF3 |
62 |
On the basis of this study, we established that a DA containing an N-hydroxy-3-trifluoromethylisoindolinone moiety and reaction conditions using Co(OAc)2 (5 mol%), Mn(OAc)3·2H2O (5 mol%), and O2 (1 atm) in TFE (0.1 M) at 40 °C represent optimal conditions (condition A). The use of a fluoroalcohol solvent was crucial for high reactivity, as fluoroalcohols are able to stabilize radicals, dissolve molecular oxygen, and are resistant to oxidation.17
Applying the cobalt-catalyzed conditions to the oxygenation of tertiary C(sp3)–H bonds, however, resulted in the formation of complex product mixtures. For example, exposing 1i to condition A afforded 2i and C–C bond-cleaved products (Scheme 2).18 As the presence of these decomposition products may be explained by the formation of hydroperoxide intermediates, we carried out a screening of the reductants in order to ensure optimal in situ reduction conditions for such problematic species. We found that Me2S provided the best results, as this additive was not susceptible to oxidation using the Co/O2 catalytic system in the absence of substrates 1.19 Further optimization finally allowed us to identify the use of Co(OAc)2 (1 mol%), Me2S (1.2 equiv.), and O2 (1 atm) in TFE (0.1 M) at 40 °C (condition B) as the best set of conditions for the oxygenation of tertiary C(sp3)–H bonds.
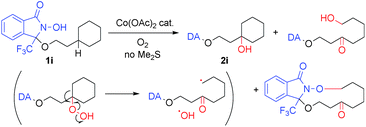 |
| Scheme 2 Undesired C–C bond cleavage of 1ivia decomposition of hydroperoxide intermediate. | |
Substrate scope and limitations
Using these conditions allowed the oxygenation of a broad variety of DA-bound alcohols as shown in Table 2. Applying condition A converted simple methylene C(sp3)–H bonds of aliphatic alcohols (1a–1g) regioselectively into the corresponding C
O bonds (2a–2g). Using condition B, the oxygenation of tertiary C(sp3)–H bonds proceeded generally in higher yield (2h–2r). The condition B was applicable to a gram-scale reaction of 1h without significant loss of efficiency.16 Using either condition A or B allowed the oxygenation of benzylic and propargylic C(sp3)–H bonds, which proceeded rapidly, even at lower temperature (2s–2ae).20
Table 2 Reaction scope with respect to alcohol substratesa
Isolated yields are described and yields in parentheses were calculated from the 1H NMR spectra of crude reaction mixtures using 1,1,2,2-tetrachloroethane as an internal standard.
The product was obtained as a cyclic hemiacetal.
2 mol% of Co(OAc)2 were used.
Starting materials 1 and products 2 were obtained as diastereoisomer mixtures.
2.2 equiv. of Me2S were used.
2 equiv. of Me2S were used.
0.05 M.
0.3 mol% Co(OAc)2 were used.
3 equiv. of Me2S were used.
0.5 mol% Co(OAc)2 were used and Me2S was added after 1 was consumed.
|
|
Our approach, based on using a radical DA and molecular oxygen, thus provided access to previously unattained C–H oxidation protocols. Especially the following three points should be worth noting: firstly, the C–H oxygenation proceeded only at specific and predictable positions depending on the accessibility of the N-oxyl radical moiety in DA. For example, the very challenging substrate 1d possesses a flexible alkyl chain, but was converted into a 1.2
:
1 mixture of γ-oxo (2d) and δ-oxo (2d′) products. Conversely, the corresponding α-, β-, and ε-oxo products were not detected or detected only in trace amounts. Oxygenation of substrates 1e–1g, containing an ester or a phthalimide moiety, occurred exclusively at the γ-position.21 Moreover, β-tertiary C–H bonds were observed to be significantly less reactive than γ-tertiary C–H bonds (2ivs.2j; 1j was unreactive in 24 h at 40 °C). Benzylic C–H oxygenations also showed a similar reactivity tendency.16 Substrates containing two or more tertiary, benzylic, and propargylic positions such as (R)-1k, 1l, 1z, 1aa, and 1ac afforded products that were selectively oxygenated at the γ-position. For DA-bound (+)-menthol, i.e. a diastereoisomer mixture of (R)-1k and (S)-1k, containing three tertiary C(sp3)–H bonds, only one specific C–H bond of (R)-1k was converted into a C–OH bond. The corresponding product (R)-2k exhibited a partial isopulegol hydrate structure and was obtained within 2 h in almost quantitative yield. However, diastereomer (S)-1k was completely unreactive. The contrasting reactivity between these two diastereomers is probably due to the accessibility of the C–H bond to the intramolecular N-oxyl radical moiety, as suggested by the X-ray structure of O-(4-nitrobenzyl)-(R)-1k and molecular modeling.16 This notion is supported particularly by the observation that the chemoselectivity can be switched, depending on the position of a specific C–H bond to the N-oxyl radical: in case of 1o, oxygenation was selective towards a γ-tertiary C–H bond rather than towards a propargyl C–H bond, whereas oxygenation of 1ac was selective towards a propargyl C–H bond rather than towards a tertiary C–H bond.
Secondly, various oxidation-sensitive functional groups were tolerated, due to the mild reaction conditions employed that avoid the use of reactive oxidants. Thus, C–H oxygenation could be conducted in the presence of electron-rich (hetero)aromatic rings (2u, 2ad and 2ae), haloarenes (2v and 2x), a silyl arene (2y), aryl and alkyl boronates (2q and 2w), a terminal hydroxy group (2m), an acetal (2n), ethers (2n, 2p, and 2u), a C
C double bond (2p), and a C
C triple bond (2o and 2aa–2ac).
Thirdly, the DA approach was able to override the innate reactivity difference between C–H bonds. The observed regioselectivity in the reaction of (R)-1k differed from that in the previously reported Fe- or Ru-catalyzed C(sp3)–H hydroxylation of O-acylmenthol.22 In addition, the γ-tertiary C–H bond was selectivity oxygenated in the reactions of 1m, 1n, 1o, and 1p, even though those compounds contain more reactive α-hydroxy (1m), acetal methylene (1n), propargylic (1o), and allylic (1p) C–H bonds.
An extension to ultra-remote aerobic C–H oxygenation
The regioselectivity can be changed via the linker structure. As shown in Scheme 3, Kemp's triacid23-based “long-arm” DA exclusively oxygenated the remote benzylic position of 3-(4-ethylphenyl)propan-1-ol (3), thus demonstrating complementary regioselectivity to the oxygenation of 1z (Table 2). The DA-promoted remote C–H oxidation was pioneered by Breslow,24 but selective and remote C–H oxygenation with use of molecular oxygen has not yet been reported in the literature. This result thus provides an opportunity for a controlled switching of the oxygenation site by designing a suitable linker between the target C(sp3)–H bond and the reactive N-oxyl radical moiety.20
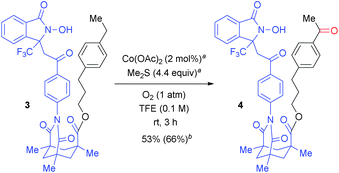 |
| Scheme 3 Selective ultra-remote aerobic C–H oxygenation. a 1 mol% Co(OAc)2 and 2.2 equiv. of Me2S were added at 0 h and 1.5 h. b Isolated yield is described and yield in parenthesis was calculated from the 1H NMR spectra of the crude mixture using 1,1,2,2-tetrachloroethane as an internal standard. | |
Confirmation of intramolecularity
To verify the intramolecular nature of the current C(sp3)–H oxygenation, we conducted several control experiments (Scheme 4). Intermolecular C–H oxygenation of O-protected 1 or 3 (O-Me-1a, O-Me-1l, O-Me-1z, O-(2-TMS-ethyl)-3) promoted by an equimolar amount of DA-bound methanol (1af) or acetophenone (1ag) produced a significantly different profile compared to oxygenation, shown in Table 2 and Scheme 3. Thus, intermolecular methylene oxygenation of O-Me-1a in the presence of 1af resulted in complete recovery of unchanged O-Me-1a after 20 h at 80 °C (Scheme 4-a), while 1a was converted to the corresponding ketone 2a in 67% yield after 18 h at 40 °C (Table 2). As for tertiary C–H oxygenation, while 1l was converted to the corresponding γ-alcohol 2l in 88% yield for 6 h at 40 °C (Table 2), O-Me-1l remained unaffected in the intermolecular oxygenation with 1af for 24 h at 60 °C. The oxygenation started to proceed at 80 °C, but the η-alcohol was obtained as the major product in low yield (<15% yield, Scheme 4-b). In the case of benzylic oxygenation, 1z was converted to ketone 2z in 72% yield in 31 h at 40 °C with perfect γ-selectivity (Table 2), while the intermolecular oxygenation of O-Me-1z with 1af proceeded only at 60 °C, and the sterically less-hindered remote benzylic position was predominantly oxygenated in moderate yield (40%, Scheme 4-c). Compound 3 was converted to 4 in 3 h at room temperature in 66% yield with perfect remote selectivity (Scheme 3), while O-(2-TMS-ethyl)-3 with 1ag required a raised temperature (50 °C), and the position selectivity was moderate (Scheme 4-d).
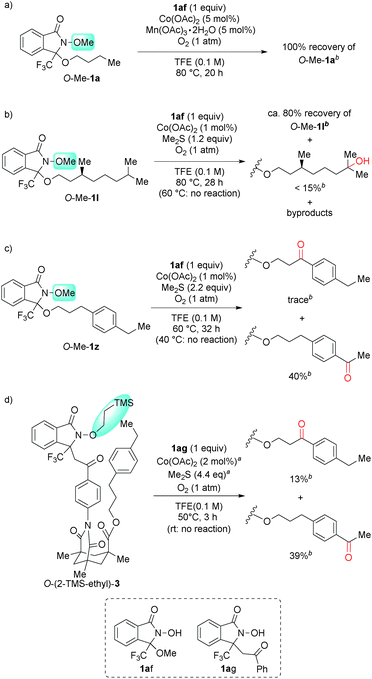 |
| Scheme 4 Confirmation of an intramolecular reaction mechanism. a 1 mol% Co(OAc)2 and 2.2 equiv. of Me2S were added at 0 h and 1.5 h. b The yield was calculated from the 1H NMR spectra of the crude mixture using 1,1,2,2-tetrachloroethane as an internal standard. | |
These contrasting results in four types of substrates strongly suggest that an intramolecular DA-promoted C–H activation is crucial for the success of the method described herein.
Plausible reaction mechanism
We propose a plausible reaction mechanism in Scheme 5. The initiation step must be the one-electron oxidation of the N-hydroxy group of 1 by Co(III) species, which are generated through the reaction between Co(II) and O2.25 The thus-generated N-oxyl radical 5 abstracts a hydrogen atom of a C–H bond at a proximate position, generating carbon radical species 6. Trapping 6 with molecular oxygen, the resulting alkyl peroxy radical 7 is quenched by a hydroperoxy radical generated in the initiation step to produce alkyl peroxide 8, or through intramolecular hydrogen abstraction from DA, generating 9. In the case of tertiary C–H oxygenation, undesired decomposition pathway from 8 (see Scheme 2)18 is suppressed by in situ reduction with Me2S to produce the corresponding tertiary alcohols. In the case of methylene oxygenation, the corresponding ketone is produced either through β-elimination from alkylperoxy species 8 or 9, or oxidation of the secondary alcohol generated from 8 with Me2S.
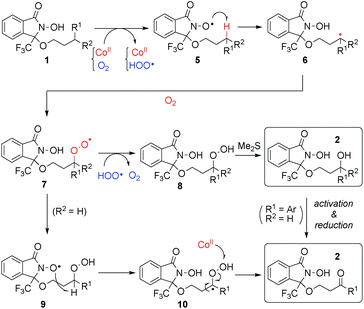 |
| Scheme 5 Plausible reaction mechanism. | |
Conclusions
In conclusion, we have developed a method for the regioselective C(sp3)–H oxygenation of aliphatic alcohols, using an N-oxyl radical group as a directing activator. Benzylic, propargylic, tertiary, and even the very challenging acyclic methylene C(sp3)–H bonds were thus converted to C
O or C–OH bonds under mild conditions (room temperature to 50 °C), while high functional group tolerance was maintained. Molecular oxygen was used as the stoichiometric oxidant, and the reactions proceeded regioselectively at the γ (and δ) position(s), whereas the α, β, and other positions beyond the δ position remained intact. This regioselectivity can be explained in terms of the intramolecular accessibility of the reactive N-oxyl radical site, despite the low regioselectivity between γ and δ positions in electronically non-biased substrates is a current limitation that must be solved in future works. Preliminary structural tuning of the DA led to an alteration of the regioselectivity, providing a selective ultra-remote aerobic C–H oxygenation. Although laborious synthesis of DA-bound substrates has remained problematic at this stage, devising catalytic applications of DAs will overcome this limitation. Efforts in such a direction are currently ongoing in our laboratory.
Acknowledgements
This work was supported in part by JSPS Scientific Research (C) (K. O.) and ERATO from JST (M. K.). We would like to thank Dr Toshiaki Sonobe and Dr Tatsuhiko Yoshino for their assistance with the X-ray analysis.
Notes and references
- For reviews on C(sp3)–H functionalization applied to the synthesis of complex molecules, see:
(a) K. Godula and D. Sames, Science, 2006, 312, 67–72 CrossRef CAS PubMed;
(b) W. R. Gutekunst and P. S. Baran, Chem. Soc. Rev., 2011, 40, 1976–1991 RSC;
(c) D. Y.-K. Chen and S. W. Youn, Chem.–Eur. J., 2012, 18, 9452–9474 CrossRef CAS PubMed;
(d) J. Yamaguchi, A. D. Yamaguchi and K. Itami, Angew. Chem., Int. Ed., 2012, 51, 8960–9009 CrossRef CAS PubMed;
(e) M. C. White, Science, 2012, 335, 807–809 CrossRef CAS PubMed;
(f) J. L. Jeffrey and R. Sarpong, Chem. Sci., 2013, 4, 4092–4106 RSC.
- J. Wencel-Delord, T. Dörge and F. Glorius, Chem. Soc. Rev., 2011, 40, 4740–4761 RSC.
-
(a) R. Giri, B.-F. Shi, K. M. Engle, N. Maugel and J.-Q. Yu, Chem. Soc. Rev., 2009, 38, 3242–3272 RSC;
(b) T. Newhouse and P. S. Baran, Angew. Chem., Int. Ed., 2011, 50, 3362–3374 CrossRef CAS PubMed;
(c) J. Mahatthananchai, A. M. Dumas and J. W. Bode, Angew. Chem., Int. Ed., 2012, 51, 10954–10990 CrossRef CAS PubMed.
- For recent reviews on remote C–H functionalization, see:
(a) I. Franzoni and C. Mazet, Org. Biomol. Chem., 2014, 12, 233–241 RSC;
(b) J. Yang, Org. Biomol. Chem., 2015, 13, 1930–1941 RSC.
- For recent reviews on aerobic unactivated C–H functionalization, see:
(a) A. E. Wendlandt, A. M. Suess and S. S. Stahl, Angew. Chem., Int. Ed., 2011, 50, 11062–11087 CrossRef CAS PubMed;
(b) E. Roduner, W. Kaim, B. Sarkar, V. B. Urlacher, J. Pleiss, R. Gläser, W.-D. Einicke, G. A. Sprenger, U. Beifuß, E. Klemm, C. Liebner, H. Hieronymus, S.-F. Hsu, B. Plietker and S. Laschat, ChemCatChem, 2013, 5, 82–112 CrossRef CAS.
- N. Burns, P. S. Baran and R. W. Hoffmann, Angew. Chem., Int. Ed., 2009, 48, 2854–2867 CrossRef CAS PubMed.
- For selected leading references on the site-selective functionalization of acyclic methylene C(sp3)–H bonds guided by directing groups, see:
(a) Y. Zhao, W.-L. Yim, C. Tan and Y.-Y. Yeung, Org. Lett., 2011, 13, 4308–4311 CrossRef CAS PubMed;
(b) S. Kawamorita, R. Murakami, T. Iwai and M. Sawamura, J. Am. Chem. Soc., 2013, 135, 2947–2950 CrossRef CAS PubMed;
(c) F.-J. Chen, S. Zhao, F. Hu, K. Chen, Q. Zhang, S.-Q. Zhang and B.-F. Shi, Chem. Sci., 2013, 4, 4187–4192 RSC;
(d) G. Shan, X. Yang, Y. Zong and Y. Rao, Angew. Chem., Int. Ed., 2013, 52, 13606–13610 CrossRef CAS PubMed.
- Bond-dissociation energies of C–H bonds adjacent to heteroatoms are generally lower than those adjacent to alkyl groups; see: S. J. Blanksby and G. B. Ellison, Acc. Chem. Res., 2003, 36, 255–263 CrossRef CAS PubMed.
- For selected leading references on DA-promoted site-selective C(sp3)–H oxygenation, see:
(a) P. A. Grieco and T. L. Stuk, J. Am. Chem. Soc., 1990, 112, 7799–7801 CrossRef CAS;
(b) D. Yang, M.-K. Wong, X.-C. Wang and Y.-C. Tang, J. Am. Chem. Soc., 1998, 120, 6611–6612 CrossRef CAS;
(c) K. Chen, J. M. Richter and P. S. Baran, J. Am. Chem. Soc., 2008, 130, 7247–7249 CrossRef CAS PubMed;
(d) S. Kasuya, S. Kamijo and M. Inoue, Org. Lett., 2009, 11, 3630–3632 CrossRef CAS PubMed;
(e) H. F. Motiwala, B. Gülgeze and J. Aubé, J. Org. Chem., 2012, 77, 7005–7022 CrossRef CAS PubMed;
(f) Y.-F. Wang, H. Chen, X. Zhu and S. Chiba, J. Am. Chem. Soc., 2012, 134, 11980–11983 CrossRef CAS PubMed;
(g) X. Chu, Y.-F. Wang, W. Ren, F.-L. Zhang and S. Chiba, Org. Lett., 2013, 15, 3214–3217 CrossRef PubMed;
(h) T. Hashimoto, D. Hirose and T. Taniguchi, Angew. Chem., Int. Ed., 2014, 53, 2730–2734 CrossRef CAS PubMed.
- For a review on (protected) alcohols as directing groups in C–H functionalization, see: F. Mo, J. R. Tabor and G. Dong, Chem. Lett., 2014, 43, 264–271 CrossRef CAS.
- For the acceleration of reaction rates by intramolecular reactivity patterns, see:
(a) M. L. Page and W. P. Jencks, Proc. Natl. Acad. Sci. U. S. A., 1971, 68, 1678–1683 CrossRef CAS PubMed;
(b) R. Pascal, Eur. J. Org. Chem., 2003, 1813–1824 CrossRef CAS;
(c) K. L. Tan, ACS Catal., 2011, 1, 877–886 CrossRef CAS;
(d) K. L. Tan, Nat. Chem., 2012, 4, 253–254 CrossRef CAS PubMed.
-
(a) Y. Ishii, S. Sakaguchi and T. Iwahama, Adv. Synth. Catal., 2001, 343, 393–427 CrossRef CAS;
(b) F. Recupero and C. Punta, Chem. Rev., 2007, 107, 3800–3842 CrossRef CAS PubMed.
-
(a) G. Majetich and K. Wheless, Tetrahedron, 1995, 51, 7095–7129 CrossRef CAS;
(b) Ž. Čeković, Tetrahedron, 2003, 59, 8073–8090 CrossRef;
(c) S. Chiba and H. Chen, Org. Biomol. Chem., 2014, 12, 4051–4060 RSC.
- For the selective aerobic oxygenation of alkenes using N-oxyl radical DAs, see:
(a) V. A. Schmidt and E. J. Alexanian, Angew. Chem., Int. Ed., 2010, 49, 4491–4494 CrossRef CAS PubMed;
(b) V. A. Schmidt and E. J. Alexanian, Chem. Sci., 2012, 3, 1672–1674 RSC.
-
(a) R. A. Sheldon and I. W. C. E. Arends, J. Mol. Catal. A: Chem., 2006, 251, 200–214 CrossRef CAS;
(b) T. Sonobe, K. Oisaki and M. Kanai, Chem. Sci., 2012, 3, 3249–3255 RSC.
- For details, see the ESI†.
- For reports on the beneficial effects of TFE in C–H functionalization, see:
(a) J. P. Bégué, D. B. Delpon and B. Crousse, Synlett, 2004, 18–29 Search PubMed;
(b) I. A. Shuklov, N. V. Dubrovina and A. Börner, Synthesis, 2007, 2925–2943 CrossRef CAS.
- S. Sakaguchi, S. Kato, T. Iwahama and Y. Ishii, Bull. Chem. Soc. Jpn., 1998, 71, 1237–1240 CrossRef CAS.
- Reducing agents other than Me2S (such as e.g. NaBH3CN, NaBH(OAc)3, Me4NBH(OAc)3, ascorbic acid, BHT, Et3SiH, TsNHNH2, Ph3P, and P(OEt)3) were tested but found to be unsuitable.
- To obtain oxygenated alcohols, DAs were cleaved under reductive (for 2h, 2aa, and 2ae) or hydrolytic (for 4) conditions. See the ESI† for details.
- The extremely high regioselectivity observed in these substrates is partially due to the deactivation of the δ-positions by the inductive effect of the electron-withdrawing groups at the ε-positions.
-
(a) M. S. Chen and M. C. White, Science, 2007, 318, 783–787 CrossRef CAS PubMed;
(b) L. Gómez, I. Garcia-Bosch, A. Company, J. Benet-Buchholz, A. Polo, X. Sala, X. Ribas and M. Costas, Angew. Chem., Int. Ed., 2009, 48, 5720–5723 CrossRef PubMed;
(c) E. McNeil and J. Du Bois, Chem. Sci., 2012, 3, 1810–1813 RSC. Du Bois' oxaziridine catalyst promoted non-selective reaction:
(d) N. D. Litvinas, B. H. Brodsky and J. Du Bois, Angew. Chem., Int. Ed., 2009, 48, 4513–4516 CrossRef CAS PubMed; Baran's method showed the same regioselectivity; see ref. 9c.
- D. S. Kemp and K. S. Petrakis, J. Org. Chem., 1981, 46, 5140 CrossRef CAS.
- For a pioneering work of non-aerobic ultra-remote C(sp3)–H functionalization using DA, see:
(a) R. Breslow and M. A. Winnik, J. Am. Chem. Soc., 1969, 91, 3083–3084 CrossRef CAS;
(b) R. Breslow and S. W. Baldwin, J. Am. Chem. Soc., 1970, 92, 732–733 CrossRef CAS;
(c) R. Breslow, J. A. Dale, P. Kalicky, S. Y. Liu and W. N. Washburn, J. Am. Chem. Soc., 1972, 94, 3276–3278 CrossRef CAS PubMed.
- I. A. Opeida, A. L. Plekhov, O. V. Kushch and M. A. Kompanets, Russ. J. Phys. Chem. A, 2012, 86, 366–368 CrossRef CAS.
Footnotes |
† Electronic supplementary information (ESI) available: Experimental details, including procedures, syntheses and characterization of new products; 1H, 13C, and 19F NMR spectra. CCDC 1415615. For ESI and crystallographic data in CIF or other electronic format, see DOI: 10.1039/c5sc04476f |
‡ These authors contributed equally to this work. |
|
This journal is © The Royal Society of Chemistry 2016 |
Click here to see how this site uses Cookies. View our privacy policy here.