DOI:
10.1039/C6SC00940A
(Edge Article)
Chem. Sci., 2016,
7, 6919-6927
Non-bonding 1,5-S⋯O interactions govern chemo- and enantioselectivity in isothiourea-catalyzed annulations of benzazoles†
Received
29th February 2016
, Accepted 2nd July 2016
First published on 4th July 2016
Abstract
Isothiourea-catalyzed annulations between 2-acyl benzazoles and α,β-unsaturated acyl ammonium intermediates are selectively tuned to form either lactam or lactone heterocycles in good yields (up to 95%) and high ee (up to 99%) using benzothiazole or benzoxazole derivatives, respectively. Computation gives insight into the significant role of two 1,5-S⋯O interactions in controlling the structural preorganization and chemoselectivity observed within the lactam synthesis with benzothiazoles as nucleophiles. When using benzazoles the absence of a second stabilizing non-bonding 1,5-S⋯O interaction leads to a dominant C–H⋯O interaction in determining structural preorganization and lactone formation.
Introduction
Nitrogen-containing heterocycles are of wide-spread importance in pharmaceutical, agrochemical and material science industries.1 In particular, benzazoles have found broad-reaching applications as bioactive compounds in medicinal chemistry, with a range of therapeutic treatments exploiting their anti-bacterial, anti-fungal, anti-parasitic and anti-cancer properties.2 In addition, they are key components of useful ligands3 as well as organic semiconductors and dyes.4 The prevalence of the benzazole motif in these applications has led the synthetic community to develop numerous methodologies for the use of benzazole containing nucleophiles for the rapid synthesis of complex heterocycles.5
Despite this interest, catalytic enantioselective functionalization of benzazole derivatives has received limited attention, with only a small number of enantioselective protocols developed to date.6 As a representative example of such an approach, Lam has shown that benzazoles undergo catalytic enantioselective nickel-catalyzed Michael-additions to nitroalkenes, giving the desired products in high yields, moderate to excellent dr and good to excellent enantioselectivity (Scheme 1, eqn (1)).7 As part of our ongoing research employing isothioureas8 in catalysis,9 we recently developed an enantioselective annulation process utilizing α,β-unsaturated acyl ammonium intermediates.10,11 In this annulation process, reaction of this intermediate with symmetrical 1,3-dicarbonyl nucleophiles generates functionalized esters in high ee after ring-opening through a postulated Michael addition-lactonization/ring-opening process (17 examples, up to 96% ee). Notably, preliminary results using unsymmetrical 2-phenacylbenzothiazole as a nucleophile gave functionalized lactams preferentially (∼85
:
15 lactam
:
lactone), resulting from preferential N- rather than O-cyclization, through a Michael addition-lactamization process in up to 86% ee in three isolated examples (Scheme 1, eqn (2)).
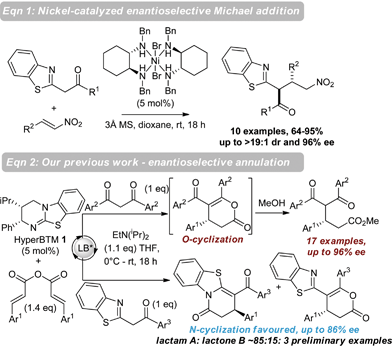 |
| Scheme 1 Previous work using benzazoles in enantioselective catalysis. Eqn (1) nickel-catalyzed Michael addition to nitroalkenes; eqn (2) isothiourea-catalyzed enantioselective annulation with α,β-unsaturated acyl ammonium intermediates. | |
This manuscript builds upon the intriguing chemoselectivity observed in the preferential formation of lactams in this latter process, and subsequently explores the effect of changing both carbonyl substitution and the heteroatom within a series of acylbenzazole nucleophiles. As a result, we have developed a highly chemoselective method to access either lactam A or lactone B heterocyclic products in excellent enantioselectivity through use of acylbenzothiazole or acylbenzoxazole derivatives respectively (Scheme 2). Furthermore, through computations, the role that non-bonding 1,5-S⋯O interactions and C–H⋯O interactions play in governing the unusual regioselectivity of these processes is highlighted. The importance of non-bonding S⋯O interactions has been widely recognized in structural and medicinal chemistry in the solid state (commonly ascribed to a stabilizing nO to σ* interaction),12 and has been used as a key controlling element to rationalize enantioselective isothiourea-catalyzed reactions.13 While the origin of this interaction is still under debate,14 and is the focus of ongoing work within our research groups, the demonstration of alternative examples of how non-bonding S⋯O interactions can facilitate selectivity in catalysis could lead to its broader utilization, akin to the current widespread use of hydrogen bonding and other non-bonding interactions in synthesis.15 To the best of our knowledge, S⋯O interactions have not been invoked to describe the origins of chemoselectivity in a catalytic reaction.
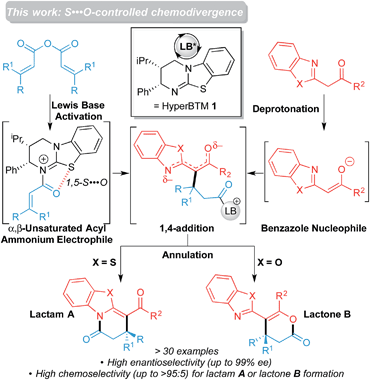 |
| Scheme 2 Chemo- and enantioselective isothiourea-catalyzed annulation of acylbenzazoles with α,β-unsaturated acyl ammonium intermediates. | |
Results and discussion
Probing the effects of acyl and benzazole substituents on annulation chemo- and enantioselectivity
Initial investigations sequentially probed substituent effects on the chemo- and enantioselectivity of this annulation process within a series of acylbenzazoles, with variation of both the acyl substituent and heterocycle tested (Scheme 3). Consistent with our previous studies, using homoanhydrides as α,β-unsaturated acyl ammonium precursors with isothiourea HyperBTM 1 (5 mol%) in bench-grade THF, 2-phenacylbenzothiazole gave preferentially lactam product 4A (88
:
12 lactam 4A
:
lactone 4B), with 4A isolated in 86% yield and 83% ee that was recrystallized to give 4A in 68% yield and 97% ee. A small amount of the lactone constitutional isomer 4B was also isolated (9% yield, 86% ee).16 The potential for isomerization of lactone 4B to the lactam 4A was investigated under a range of conditions. Treatment of the minor lactone product 4B with base, with base and HyperBTM, or to the reaction conditions, led to no interconversion of lactone to lactam, consistent with the observed product ratios arising from kinetic control (see ESI† for further details). The incorporation of electron donor benzothiazole amides and esters resulted in the exclusive formation of lactams 2A and 3A as single constitutional isomers in excellent ee (97% and 93% ee) and in good yields respectively. Further studies probed the effect of variation within the heterocyclic portion of the benzazole. While using 2-phenacylbenzothiazole leads to preferential formation of lactam 4A, remarkably, 2-phenacylbenzoxazole afforded exclusively lactone product (>95
:
5 5B
:
5A) with the lactone 5B isolated in 95% yield and 98% ee. The seemingly trivial substrate change from benzothiazole to benzoxazole in this system promotes a change in chemoselectivity in the annulation process to selectively facilitate lactone (O-cyclization) rather than lactam (N-cyclization) product formation.
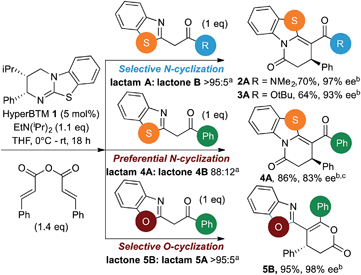 |
| Scheme 3 Probing the effects of acyl and benzazole substituents on annulation chemo- and enantioselectivity. aRatio of constitutional isomers arising from either N- or O-cyclization calculated from 1H NMR spectra of crude reaction product. bee values obtained via chiral HPLC. cFollowing a single recrystallization ee could be enhanced to 97%. | |
Scope and generality
To demonstrate the generality of these chemo- and enantioselective annulation processes, and facilitate direct comparison across a range of substrates, the use of 2-phenacylbenzothiazole, 2-phenacylbenzoxazole and 2-N,N-dimethylacetamidobenzothiazole as nucleophiles was fully investigated with a range of anhydrides (Table 1).
Table 1 Chemo- and enantioselective formation of lactam or lactone products
Consistent with the model studies, chemoselective formation of either lactam or lactone products (>95
:
5 ratio of constitutional isomers) was achieved by using 2-N,N-dimethylacetamidobenzothiazole or 2-phenacylbenzoxazole, with excellent enantioselectivity (90–99% ee) observed across a range of anhydrides. Using 2-phenacylbenzothiazole led to preferential lactam formation (typically ∼85
:
15 lactam
:
lactone), albeit with reduced enantioselectivity (typically >80% ee). For all acylbenzazole nucleophiles, variation of aryl substitution within the anhydride was tolerated, including electron donating (4-MeOC6H4), electron withdrawing (4-CF3C6H4), and 3-BrC6H4 substitution. Sterically demanding 2-BrC6H4 substitution led to no reaction with 2-phenacylbenzoxazole, while reactions using 2-N,N-dimethylacetamidobenzothiazole or 2-phenacylbenzothiazole gave acceptable to good product yields, with excellent enantioselectivity in the amide series. Heteroaryl (2-furyl, 3-furyl, and 3-thiophenyl) substituents were also successfully incorporated (90–99% ee), as were methyl and ester substitution. In the 2-phenacylbenzothiazole derived series, the ee of lactam and lactone products were approximately equivalent, except for 16A/16B (81% and 33% ee respectively) bearing a 2-Br substituent. The origin of this variation in ee is currently unexplained, despite extensive synthetic and computational investigations.17
Excited by the high chemo- and enantiocontrol observed, the scope of this process was expanded to the synthesis of challenging all-carbon quaternary centers (Scheme 4). Trisubstituted homoanhydrides were used as α,β-unsaturated acyl ammonium precursors, allowing limited access to stereogenic quaternary centers for the first time in this methodology. Initial studies employed 3-methylbut-2-enoic anhydride 32 and gave the expected achiral lactam product 33 in good yield (Scheme 4).
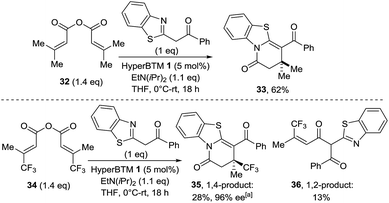 |
| Scheme 4 Generation of all-carbon quaternary centres.a | |
Unfortunately, when (2E)-3-phenylbut-2-enoic anhydride was examined under the same conditions, no reaction was observed. The use of (2E)-4,4,4-trifluoro-3-methylbut-2-enoic anhydride 34 proved compatible with this methodology,18 leading to cyclized lactam product 35 containing a stereogenic quaternary trifluoromethyl group in moderate yield but 96% ee. Notably no lactone products were observed in this annulation process, although 1,2-addition product 36 was isolated in 13% yield as a side-product.
Scale-up and derivatizations
To demonstrate the potential further utility of the heterocyclic products obtained, scale-up and derivatization through palladium-catalyzed cross-coupling reactions was tested. 3-BrC6H4-substituted lactam 14A was readily prepared on gram scale in high yield and enantioselectivity (1.15 g, 75%, 96% ee). Subjecting lactam 14A to Suzuki coupling generated 37 in 70% yield with no erosion of enantioselectivity; similarly, Heck reaction of 14A with methyl acrylate afforded 38 in 89% yield and 97% ee (Scheme 5).
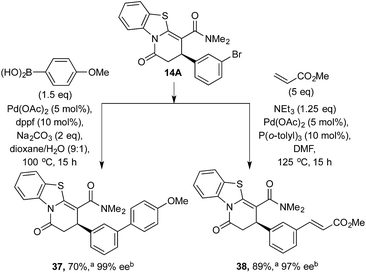 |
| Scheme 5 Product derivatization through cross coupling. a Isolated yield. b ee values obtained via chiral HPLC. | |
Computational details and mechanism
Computations were undertaken to provide insight into the observed chemoselectivity when using the benzoxazole and benzothiazole nucleophiles (X = O or S, respectively). For this purpose, we have specifically computed the intermediates and transition structures involved in the formation of products 4A (lactam) and 4B (lactone) using 2-phenacylbenzothiazole, and 5B (lactone) using 2-phenacylbenzoxazole. All energy refinements and geometries were computed in solution using the implicit polarizable continuum model PCM with tetrahydrofuran as solvent (M06-2X/6-31+G(d,p)/PCM(THF)//M06-2X/6-31G(d)/PCM(THF)19).20 The M06-2X DFT method has been successfully used to rationalize mechanisms and selectivities of synthetic reactions by us and others.21 Given the zwitterionic nature of many of the intermediates in the reaction, we also took into account the ability of M06-2X to accurately evaluate dispersion-heavy and ionic systems relative to the less computationally expensive B3LYP method.22 The catalytic cycle is shown in Fig. 1.23 Stepwise N-acylation of HyperBTM leads to the α,β-unsaturated acyl ammonium intermediate. Stereodetermining 1,4-addition of the anionic benzazole nucleophile and proton transfer leads to the pre-cyclization intermediate, which can either lactamize or lactonize. Restoration of the carbonyl π-bond releases the product and regenerates HyperBTM 1.
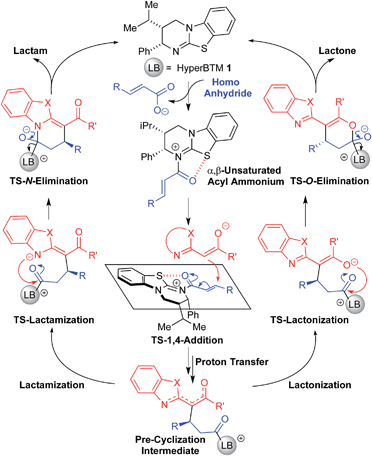 |
| Fig. 1 Catalytic cycle of the isothiourea-catalyzed annulations between 2-acyl benzazoles and homoanhydrides to form the lactam (left) or the lactone (right) using benzothiazole (X = S) or benzoxazole (X = O) derivatives, respectively. | |
S⋯O interaction.
Considering this mechanistic scheme, within all key reactive intermediates and transition states where S- and O-atoms contain 1,5-connectivity (such as from the carbonyl C
O and isothiourea S within the acylammonium intermediate, or 2-phenacylbenzothiazole carbonyl-O and benzothiazole-S), these atoms are co-planar. The internuclear distances (within the range of 2.53–2.70 Å) are significantly less than the sum of the van der Waals radii (3.4 Å).24 These observations are consistent with an attractive force between the S- and O-atoms and in line with previous computations by Tantillo and Romo13b as well as by Houk and Birman.13c Unique to this system, however, is how this interaction dominates the structural preorganization of all key reactive intermediates and transition states of this annulation process.
S⋯O interaction in the enantiocontrol of 1,4-addition.
All stable conformations of the α,β-unsaturated acyl ammonium intermediate exhibit coplanarity of the 1,5-O and S atoms. This is corroborated by the crystal structure of this intermediate which show the S–O being coplanar at a distance of 2.48 Å.10a In addition, both anionic nucleophiles prefer the planar arrangement (Fig. 2), with the 1,5-S–O syn conformation favored by ∼7 kcal mol−1 in the case of benzothiazole. Taken together, these factors rigidify and planarize both the electrophilic α,β-unsaturated acyl ammonium intermediate and the incoming nucleophile, dramatically simplifying the stereochemical model. Nucleophilic 1,4-addition occurs anti to the catalyst stereodirecting groups on the less hindered face. The computed enantioselectivities of 99% in both cases are in reasonable agreement with experiments (83% and 98% ee for 4A and 5B, respectively, Scheme 3).
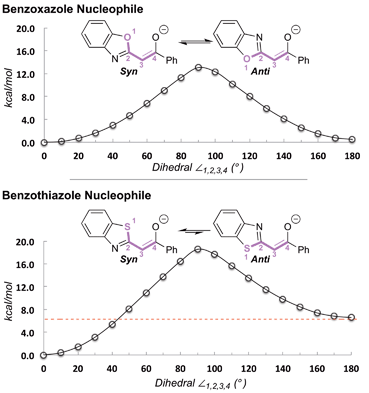 |
| Fig. 2 Conformational preferences of anionic benzoxazole and benzothiazole nucleophiles.25 | |
Lactamization vs. lactonization.
The interplay between S⋯O and C–H⋯O interactions26 (between the anionic nucleophile atoms and C–H α-to the positively-charged nitrogen of the acylated HyperBTM) governs cyclization chemoselectivity. Fig. 3 shows computed model complexes analogous to the pre-cyclization intermediate, featuring truncated simplified structures of both HyperBTM catalyst and benzazole nucleophiles. In the oxazole model system, the conformation with one S⋯O and one C–H⋯O interaction is favored by 2.5 kcal mol−1 over the conformation featuring the unfavorable O⋯O. However, in the thiazole model, the conformation featuring two S⋯O interactions, rather than one S⋯O and one C–H⋯O, is preferred by 3.7 kcal mol−1.
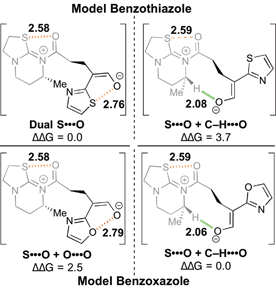 |
| Fig. 3 Model systems probing the relative energetic values (in kcal mol−1) between S⋯O and C–H⋯O nonbonding interactions.26 | |
These preferences carry over to the cyclization transition structures (Fig. 4). In the benzoxazole case, both annulations occur via a boat-like six-membered transition structure anti to the catalyst stereodirecting groups (phenyl and isopropyl) to minimize steric occlusion. The Favored-Lactonization-(X = O)-TS is preferred over the Disfavored-Lactamization-(X = O)-TS (ΔG‡ = 11.1 and 14.3 kcal mol−1, respectively) due to a stabilizing C–H⋯O involving the ortho C–H of the catalyst and the incoming oxygen atom. In the latter, a β-C–H is involved in a repulsive interaction with the incoming benzoxazole. The computed selectivity of 99
:
1 matches well with the experimental selectivity of 98
:
2 seen with lactone 5B.
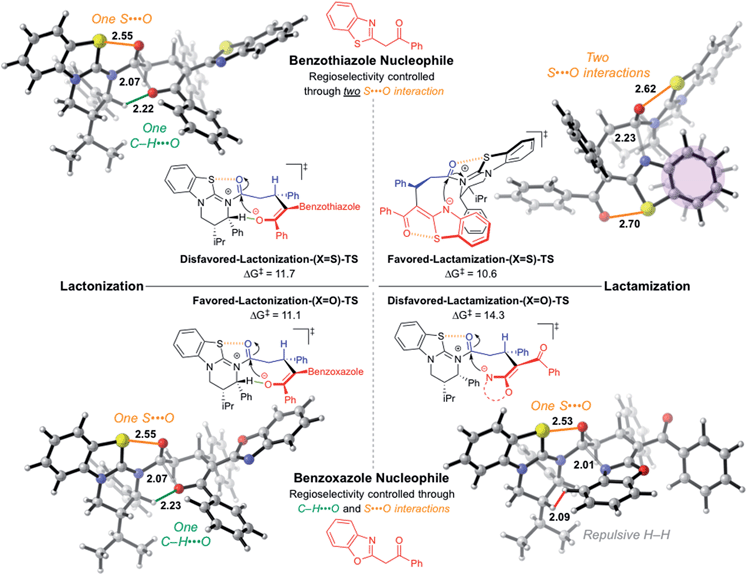 |
| Fig. 4 Computed chemoselectivity determining cyclization transition structures for benzoxazole and thiazole nucleophiles. All transition structures are stepwise (tetrahedral intermediate formation followed by HBTM release) except for favored-lactamization-(X = S)-TS (see ESI† for computed reaction coordinates). Forming bonds shown in grey. S⋯O interactions shown in orange, C–H⋯O highlighted in green, and van der Waals repulsion shown in red. Aromatic interaction shaded in purple. Relative energy values given in kcal mol−1. Structure images rendered using CYLview.28 | |
The benzothiazole lactone closure occurs exactly as the benzoxazole case through the Disfavored-Lactonization-(X = S)-TS (ΔG‡ = 11.7 kcal mol−1). The Favored-Lactamization-(X = S)-TS has a lower barrier (ΔG‡ = 10.6 kcal mol−1), and the computed selectivity of 88
:
12 matches experiments. Interestingly, lactamization occurs on the same face as the catalyst stereodirecting groups, previously thought to be disfavored due to the steric occlusion.
Two key stabilizing interactions are present in benzothiazole lactamization that are not found in lactonization: (1) π-stacking of the catalyst phenyl and the fused benzene of the benzothiazole ring,27 and (2) a second 1,5-S⋯O interaction within the former benzothiazole nucleophile. The switch in chemoselectivity in favor of lactam formation using the benzothiazole is attributed to the penalty of breaking the 1,5-S⋯O present within the benzothiazole nucleophile for the lactonization process to proceed.
Conclusions
To conclude, we have demonstrated the scope and limitations of the organocatalytic enantioselective functionalization of a range of benzazole nucleophiles using the isothiourea HyperBTM 1 and α,β-unsaturated homoanhydrides as α,β-unsaturated acyl ammonium precursors. The chemoselectivity observed during the cyclization is influenced by the nature of the benzazole and the carbonyl employed within the acylbenzazole, with benzothiazole preferentially using the ring-nitrogen to extrude the catalyst, whereas the benzoxazole moiety prefers to cyclize through the β-carbonyl substituent. Computations elucidated the importance of non-covalent 1,5-S⋯O interactions in determining the chemoselectivity within these processes. Specifically, the use of benzothiazole nucleophiles allows two stabilizing 1,5-S⋯O interactions in the preferred lactamization transition structure, while benzoxazole contains one stabilizing 1,5-S⋯O and one C–H⋯O interaction in the lactonization transition structure. Future research within our laboratories is aimed at harnessing the collaboration between theory and experiments towards the development of isothiourea Lewis base catalysts in new enantioselective transformations.
Acknowledgements
We thank the Royal Society (URF to ADS), the EPSRC (ERTR – grant code EP/J500549/1) and the European Research Council under the European Union's Seventh Framework Programme (FP7/2007–2013) ERC grant agreement no. 279850 (CF). We also thank the EPSRC UK National Mass Spectrometry Facility at Swansea University. PHYC is the Bert and Emelyn Christensen professor of OSU, and gratefully acknowledges financial support from the Vicki & Patrick F. Stone family, the National Science Foundation (NSF, CHE-1352663), and the computing infrastructure in part provided by the NSF Phase-2 CCI, Center for Sustainable Materials Chemistry (NSF CHE-1102637). DMW also acknowledges financial support from the N. L. Tartar Fellowship, Johnson Research Fellowship, and Bruce Graham Memorial Scholarship.
Notes and references
-
(a) R. Dua, S. Shrivastava, S. K. Sonwane and S. K. Srivastava, Adv. Biol. Res., 2011, 5, 120–144 CAS;
(b)
T. Eicher and S. Hauptmann, The Chemistry of Heterocycles: Structure, Reactions, Syntheses, and Applications, Wiley-VCH, Weinheim, Germany, 2nd edn, 2003 Search PubMed.
-
(a) R. S. Keri, M. R. Patil, S. A. Patil and S. Budagumpi, Eur. J. Med. Chem., 2015, 89, 207–251 CrossRef CAS PubMed;
(b) S. Noel, S. Cadet, E. Gras and C. Hureau, Chem. Soc. Rev., 2013, 42, 7747–7762 RSC;
(c) Y. Bansal and O. Silakari, Bioorg. Med. Chem., 2012, 20, 6208–6236 CrossRef CAS PubMed;
(d) C. S. Demmer and L. Bunch, Eur. J. Med. Chem., 2014, 97, 778–785 CrossRef PubMed.
-
(a) J. Kuwabara, T. Namekawa, M.-A. Haga and T. Kanbara, Dalton Trans., 2012, 41, 44–46 RSC;
(b) C. Zhang, S.-B. Yu, X.-P. Hu, D.-Y. Wang and Z. Zheng, Org. Lett., 2010, 12, 5542–5545 CrossRef CAS PubMed;
(c) B. Wang, S. Wang, C. Xia and W. Sun, Chem.–Eur. J., 2012, 18, 7332–7335 CrossRef CAS PubMed.
- F. S. Rodembusch, F. P. Leusin, L. F. da Costa Medina, A. Brandelli and V. Stefani, Photochem. Photobiol. Sci., 2005, 4, 254–259 CAS.
- For selected recent examples, see:
(a) Q. Cai, Z. Li, J. Wei, L. Fu, C. Ha, D. Pei and K. Ding, Org. Lett., 2010, 12, 1500–1503 CrossRef CAS PubMed;
(b) H. De Silva, S. Chatterjee, W. P. Henry and C. U. Pittman Jr, Synthesis, 2012, 44, 3453–3464 CrossRef CAS.
-
(a) L. M. Stanley and J. F. Hartwig, J. Am. Chem. Soc., 2009, 131, 8971–8983 CrossRef CAS PubMed;
(b) L. Li, B.-A. Song, P. S. Bhadury, Y.-P. Zhang, D.-Y. Hu and S. Yang, Eur. J. Org. Chem., 2011, 2011, 4743–4746 CAS;
(c) H.-X. He, W. Yang and D.-M. Du, Adv. Synth. Catal., 2013, 355, 1137–1148 CrossRef CAS;
(d) K. Xu, N. Thieme and B. Breit, Angew. Chem., Int. Ed., 2014, 53, 2162–2165 CrossRef CAS PubMed;
(e) H.-X. He and D.-M. Du, Eur. J. Org. Chem., 2014, 2014, 6190–6199 CrossRef CAS.
- C. Fallan and H. W. Lam, Chem.–Eur. J., 2013, 18, 11214–11218 CrossRef PubMed.
- For seminal work on isothiourea catalysis see:
(a) V. B. Birman and X. Li, Org. Lett., 2006, 8, 1351–1354 CrossRef CAS PubMed;
(b) V. B. Birman, H. Jiang, X. Li, L. Guo and E. W. Uffman, J. Am. Chem. Soc., 2006, 128, 6536–6537 CrossRef CAS PubMed;
(c) M. Kobayashi and S. Okamoto, Tetrahedron Lett., 2006, 47, 4347–4350 CrossRef CAS;
(d) V. B. Birman and X. Li, Org. Lett., 2008, 10, 1115–1118 CrossRef CAS PubMed;
(e) Y. Zhang and V. B. Birman, Adv. Synth. Catal., 2009, 351, 2525–2529 CrossRef CAS PubMed;
(f) C. Joannesse, C. P. Johnston, C. Concellón, C. Simal, D. Philp and A. D. Smith, Angew. Chem., Int. Ed., 2009, 48, 8914–8918 CrossRef CAS PubMed. For recent reviews, see:
(g) L. C. Morrill and A. D. Smith, Chem. Soc. Rev., 2014, 43, 6214–6226 RSC;
(h) J. E. Taylor, S. D. Bull and J. M. J. Williams, Chem. Soc. Rev., 2012, 41, 2109–2121 RSC.
- For selected examples see
(a) D. Belmessieri, L. C. Morrill, C. Simal, A. M. Z. Slawin and A. D. Smith, J. Am. Chem. Soc., 2011, 133, 2710–2714 CrossRef PubMed;
(b) D. Belmessieri, D. B. Cordes, A. M. Z. Slawin and A. D. Smith, Org. Lett., 2013, 15, 3472–3475 CrossRef CAS PubMed;
(c) D. G. Stark, L. C. Morrill, P.-P. Yeh, A. M. Z. Slawin, T. J. C. O'Riordan and A. D. Smith, Angew. Chem., Int. Ed., 2013, 52, 11642–11646 CrossRef CAS PubMed;
(d) S. R. Smith, C. Fallan, J. E. Taylor, R. McLennan, D. S. B. Daniels, L. C. Morrill, A. M. Z. Slawin and A. D. Smith, Chem.–Eur. J., 2015, 21, 10530–10536 CrossRef CAS PubMed.
-
(a) E. R. T. Robinson, C. Fallan, C. Simal, A. M. Z. Slawin and A. D. Smith, Chem. Sci., 2013, 4, 2193–2200 RSC; for other examples of related work using α,β-unsaturated acyl ammonium intermediates, see:
(b) E. Bappert, P. Müller and G. C. Fu, Chem. Commun., 2006, 2604–2606 RSC;
(c) S. Vellalath, K. N. Van and D. Romo, Angew. Chem., Int. Ed., 2013, 52, 13688–13693 CrossRef CAS PubMed;
(d) G. Liu, M. E. Shirley, K. N. Van, R. L. McFarlin and D. Romo, Nat. Chem., 2013, 5, 1049–1057 CrossRef CAS PubMed;
(e) S. Goudedranche, X. Bugaut, T. Constantieux, D. Bonne and J. Rodriguez, Chem.–Eur. J., 2014, 20, 410–415 CrossRef CAS PubMed;
(f) Y. Fukata, T. Omamura, K. Asano and S. Matsubara, Org. Lett., 2014, 16, 2184–2187 CrossRef CAS PubMed;
(g) Y. Fukata, K. Asano and S. Matsubara, J. Am. Chem. Soc., 2015, 137, 5320–5323 CrossRef CAS PubMed.
- For a recent review of related work using α,β-unsaturated acyl azolium intermediates, see: L. Candish, Y. Nakano and D. W. Lupton, Synthesis, 2014, 46, 1823–1835 CrossRef.
-
(a) B. R. Beno, K.-S. Yeung, M. D. Bartberger, L. D. Pennington and N. A. Meanwell, J. Med. Chem., 2015, 58, 4383–4438 CrossRef CAS PubMed;
(b) R. C. Reid, M.-K. Yau, R. Singh, J. Lim and D. P. Fairlie, J. Am. Chem. Soc., 2014, 136, 11914–11917 CrossRef CAS PubMed;
(c) F. T. Burling and B. M. Goldstein, J. Am. Chem. Soc., 1992, 114, 2313–2320 CrossRef CAS.
- For the initial postulate of 1,5-S⋯O interactions as a control element in isothiourea catalysis see
(a) V. B. Birman, X. Li and Z. Han, Org. Lett., 2007, 9, 37–40 CrossRef CAS PubMed. For other manuscripts of interest see
(b) M. E. Abbasov, B. M. Hudson, D. J. Tantillo and D. Romo, J. Am. Chem. Soc., 2014, 136, 4492–4495 CrossRef CAS PubMed;
(c) P. Liu, X. Yang, V. B. Birman and K. N. Houk, Org. Lett., 2012, 14, 3288–3291 CrossRef CAS PubMed. Romo and Tantillo have probed the nature of 1,5-S⋯O interactions of α,β-unsaturated acyl ammonium species with NBO and postulate this interaction is due to a number of orbital interactions. In particular, unfavorable nS ⇔ σ*C–H/σC–H interactions disfavor alternative conformations with an O–C–N–C dihedral angle of 180°. See ref. 13b.
- See leading publications in ref.
12 and 13 and the following for a selection of discussions:
(a) X. Zhang, Z. Gong, J. Li and T. Lu, J. Chem. Inf. Model., 2015, 55, 2138–2153 CrossRef CAS PubMed;
(b) J. G. Ángyán, Á. Kucsman, R. A. Poirier and I. G. Csizmadia, J. Mol. Struct.: THEOCHEM, 1985, 123, 189–201 CrossRef;
(c) J. S. Murray, P. Lane and P. Politzer, Int. J. Quantum Chem., 2008, 108, 2770–2781 CrossRef CAS;
(d) M. Iwaoka, S. Takemoto and S. Tomoda, J. Am. Chem. Soc., 2002, 124, 10613–10620 CrossRef CAS PubMed;
(e) K. A. Brameld, B. Kuhn, D. C. Reuter and M. Stahl, J. Chem. Inf. Model., 2008, 48, 1–24 CrossRef CAS PubMed.
- For selected reviews, see:
(a) A. G. Doyle and E. N. Jacobsen, Chem. Rev., 2007, 107, 5713–5743 CrossRef CAS PubMed;
(b) P. R. Schreiner, Chem. Soc. Rev., 2003, 32, 289–296 RSC.
- The absolute configurations of all products are based upon previous reports of annulations using α,β-unsaturated acyl ammonium intermediates (see ref. 10 and 11).
- Synthetic investigations showed no change in the % ee of lactone 16B over the course of the reaction, and no racemization of the isolated product under standard reaction conditions. QM computations reveal that this particular case is a unique exception to all other cases discussed in the manuscript. The enantioselectivity in this case does not simply derive from the 1,4-addition step. Computed enantioselectivity is 1.7 kcal mol−1 for both the lactam and lactone based on the computed 1,4-addition transition structures. This compares favorably for the experimental enantioselectivity of the lactam at 1.1 kcal mol−1, but compares poorly to the enantioselectivity of the lactone at 0.4 kcal mol−1. See ESI† for further details.
- The corresponding acid (E
:
Z ratio 89
:
11) was prepared following a literature procedure ( P. Tarrant and R. E. Taylor, J. Org. Chem., 1959, 24, 1888–1890 CrossRef CAS ). The anhydride was prepared from this mixture, presumably as a statistical ratio of stereoisomers, and used without purification (see ESI† for further information).
-
(a) Y. Zhao and D. G. Truhlar, Theor. Chem. Acc., 2008, 120, 215–241 CrossRef CAS;
(b) P. C. Hariharan and J. A. Pople, Theor. Chim. Acta, 1973, 28, 213–222 CrossRef CAS;
(c) W. J. Hehre, R. Ditchfield and J. A. Pople, J. Chem. Phys., 1972, 56, 2257–2261 CrossRef CAS;
(d) S. Miertuš, E. Scrocco and J. Tomasi, Chem. Phys., 1981, 55, 117–129 CrossRef.
- See ESI† for full authorship of Gaussian09:
M. J. Frisch, et al., Gaussian 09, Gaussian, Inc.: Wallingford, CT, 2009 Search PubMed.
- E. Gould, D. M. Walden, K. Kasten, R. C. Johnston, J. Wu, A. M. Z. Slawin, T. J. L. Mustard, B. Johnston, T. Davies, P. H.-Y. Cheong and A. D. Smith, Chem. Sci., 2014, 5, 3651–3658 RSC.
- M. Walker, A. J. A. Harvey, A. Sen and C. E. H. Dessent, J. Phys. Chem. A, 2013, 117, 12590–12600 CrossRef CAS PubMed.
- See ESI† for reaction coordinates, intermediate and transition structures, model systems, and energetics.
- S. Alverez, Dalton Trans., 2013, 42, 8617–8636 RSC.
- All geometries, energies, and thermal corrections obtained using MO6–2X/6-31+G(d,p)/PCM(THF)//MO6–2X/6-31G(d)/PCM(THF). Distances in Ångströms (Å); energies in kcal mol−1.
-
(a) D. M. Walden, O. M. Ogba, R. C. Johnston and P. H.-Y. Cheong, Acc. Chem. Res., 2016, 49, 1279–1291 CrossRef CAS PubMed;
(b) P. Maity, R. P. Pemberton, D. J. Tantillo and U. K. Tambar, J. Am. Chem. Soc., 2013, 135, 16380–16383 CrossRef CAS PubMed;
(c) O. Pattawong, T. J. L. Mustard, R. C. Johnston and P. H.-Y. Cheong, Angew. Chem., Int. Ed., 2013, 52, 1420–1423 CrossRef CAS PubMed;
(d) R. C. Johnston and P. H.-Y. Cheong, Org. Biomol. Chem., 2013, 11, 5057–5064 RSC;
(e) M. N. Paddon-Row, C. D. Anderson and K. N. Houk, J. Org. Chem., 2009, 74, 861–868 CrossRef CAS PubMed;
(f) E. J. Corey and J. J. Rohde, Tetrahedron Lett., 1997, 38, 37–40 CrossRef CAS.
-
(a) S. Wheeler, Acc. Chem. Res., 2013, 46, 1029–1038 CrossRef CAS PubMed;
(b) E. H. Krenske and K. N. Houk, Acc. Chem. Res., 2013, 46, 979–989 CrossRef CAS PubMed;
(c) M. O. Sinnokrot, E. F. Valeev and C. D. Sherrill, J. Am. Chem. Soc., 2002, 124, 10887–10893 CrossRef CAS PubMed.
-
C. Y. Legault, CYLview, 1.0b, Université de Sherbrooke, 2009 Search PubMed.
Footnote |
† Electronic supplementary information (ESI) available: 1H and 13C{1H} NMR spectra and HPLC traces of all novel compounds. Coordinates, thermal corrections, and energies of all computed structures. See DOI: 10.1039/c6sc00940a |
|
This journal is © The Royal Society of Chemistry 2016 |
Click here to see how this site uses Cookies. View our privacy policy here.