DOI:
10.1039/C6SC03012B
(Edge Article)
Chem. Sci., 2016,
7, 7040-7049
Synthesis of malhamensilipin A exploiting iterative epoxidation/chlorination: experimental and computational analysis of epoxide-derived chloronium ions†
Received
8th July 2016
, Accepted 2nd August 2016
First published on 2nd August 2016
Abstract
We report a 12-step catalytic enantioselective formal synthesis of malhamensilipin A (3) and diastereoisomeric analogues from (E)-2-undecenal. The convergent synthesis relied upon iterative epoxidation and phosphorus(V)-mediated deoxydichlorination reactions as well a titanium-mediated epoxide-opening to construct the C11–C16 stereohexad. The latter transformation occurred with very high levels of stereoretention regardless of the C13 configuration of the parent epoxide, implicating anchimeric assistance of either the γ- or δ-chlorine atoms, and the formation of chloretanium or chlorolanium ions, respectively. A computational analysis of the chloronium ion intermediates provided support for the involvement of chlorolanium ions, whereas the potential chloretanium ions were found to be less likely intermediates on the basis of their greater carbocationic character.
Introduction
The chlorosulfolipids (Scheme 1A) are a family of bioactive polychlorinated natural products1 isolated from algal sources and toxic mussels.2 Since the original reports in the late 1960s,3 the family has grown and many of the gross structures have been refined to include relative and absolute stereochemistry.4 The stereochemical challenges posed by these unique lipids have stimulated the interest of synthesis chemists around the world and the first total synthesis of a chlorosulfolipid was reported by the Carreira group in 2009.5 Following this work syntheses of mytilipin A (3),6 danicalipin A (1),7 malhamensilipin A (2)8 and mytilipin B (4)9 were reported (Scheme 1A).
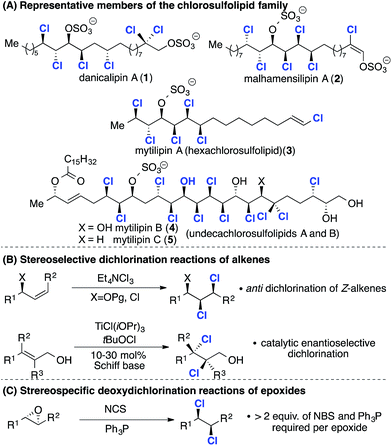 |
| Scheme 1 (A) Selected chlorosulfolipids. (B) and (C) Synthesis methods. | |
In terms of synthesis strategies two complementary dichlorination reactions have been applied. The first method (Scheme 1B, top), examined in detail by Vanderwal and co-workers, involves anti dichlorination of alkenes using tetraethylammonium trichloride.10 This dichlorination reaction is a feature of many of the groundbreaking syntheses reported by both the Vanderwal and Carreira groups.2 Very recently Burns and co-workers have developed catalytic enantioselective chlorination reactions of allylic alcohols and applied them in synthesis of (deschloro)mytilipin A and danicalipin A (2) (Scheme 1B, bottom).11 This work, along with other methods developed by the Snyder,12 Nicolaou and13 Denmark14 groups opens up new approaches to polyhalogenated target molecules. The second approach (Scheme 1C) involves deoxydichlorination reactions of epoxides, reported by Tanaka and Yoshimitsu in 2009.15 While this reaction has found application in the synthesis of mytilipin A (4) and danicalipin A (2)6a,7b it generates stoichiometric quantities of organic waste.
We reasoned that a catalytic deoxydichlorination reaction would provide a powerful alternative to alkene dichlorination and in 2011 we reported a catalytic reaction that generates CO2 and CO as the sole by-products (Scheme 2A).16 The integration of this reaction into an iterative epoxidation/chlorination sequence (Scheme 2B) provides a general and systematic approach to the chlorine- and hydroxyl-containing acyclic stereochemical arrays present within the chlorosulfolipids (Scheme 2B). Significantly, any stereochemical permutation can be accessed using this approach by control of epoxide configuration.
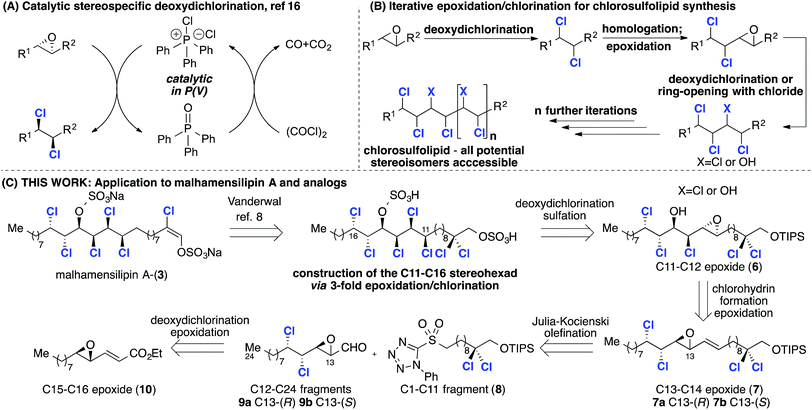 |
| Scheme 2 (A) Catalytic deoxydichlorination of epoxides (B) general synthesis strategy for chlorosulfolipids (C) application to malhamensilipin A. | |
Herein we demonstrate the power of the iterative epoxidation/chlorination strategy (Scheme 2B) with a catalytic enantioselective formal synthesis of malhamensilipin A4f (2) and stereoisomeric analogues. We also illustrate the applicability of Carreira's NMR database17 for the assignment of polychlorinated stereotetrads and stereohexads. Finally, we analyse ring-opening reactions of γ,δ-dichlorovinyl epoxides and report computational studies that give new insights into the structure of the chloronium ion intermediates which have been proposed to intervene in these reactions.
Synthesis plan
Our retrosynthetic analysis of malhamensilipin A (Scheme 2C) is based upon three iterations of the epoxidation/chlorination sequence for the construction of the C11–C16 stereohexad and involves epoxides 10, 7 and 6 as intermediates. While the stereochemical outcome of the two deoxydichlorination processes was secure, the ring opening of epoxide 7 could occur with either retention or inversion of configuration at C13 (ref. 5) and therefore both C13 diastereoisomers, 7a and 7b were targeted. Disconnection of the C11–C12 E-olefin was predicated upon the modified Julia–Kocienski olefination and led to sulfone 8 and aldehydes 9a and 9b. The aldehydes, in either diastereoisomeric series, were projected to arise from enoate 10, itself derived from E-2-undecenal.
Results and discussion
Fragment synthesis
We began with the synthesis of the more complex C12–C24 fragment (Scheme 3). Thus, catalytic enantioselective nucleophilic epoxidation of enal 11,18,19 provided the corresponding epoxide 12 in excellent yield and 97
:
3 enantiomeric ratio. The conversion of the epoxide into enoate 10 was effected through a Horner–Wadsworth–Emmons reaction, which provided the enoate with a d.r. in excess of 20
:
1. Phosphorus(V)-mediated catalytic deoxydichlorination16 of this substrate afforded dichloride 13 with the required C15–C16 syn stereochemistry in 51% isolated yield. By-product 14 accounted for the remainder of the mass-balance and is most likely derived from a competing elimination from the chloroalkoxyphosphonium salt intermediate A.20
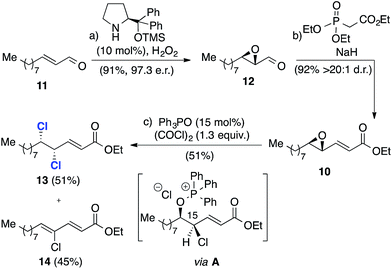 |
| Scheme 3 Towards the C12–C24 fragment. Reagents and conditions: (a) 10 mol% catalyst, H2O2, CHCl3, r.t., 91%, 97 : 3 e.r.; (b) NaH, THF −78 °C to r.t., 92%, >20 : 1 d.r.; (c) 15 mol% Ph3PO, 1.3 equiv. (COCl)2, C6H6, 80 °C, 51%. | |
With intermediate 13 in hand, the two diastereoisomeric C12–C24 aldehydes 9a and 9b were prepared (Scheme 4A). A sequence consisting of diastereoselective Upjohn dihydroxylation (81%, 10
:
1 d.r.), cyclization and reduction (15 → 16 → 9a, Scheme 4) afforded epoxide 9a.21 The stereochemical outcome of the dihydroxylation was confirmed by an X-ray crystal structure of 15 and is in agreement with results previously obtained by Carreira5 (notwithstanding the stereochemical difference at C10) and Vanderwal, who introduced the C12 and C13 hydroxyl groups after dichlorination.8 Inspection of the crystal structure of 15 indicated a gauche conformation with respect to the C15 and C16 chlorine atoms and that the C14 hydroxyl and C15 chlorine are oriented anti with respect to each other. Analysis of the relevant vicinal coupling constants (Scheme 4) revealed that the molecule adopts the same conformation in solution. The diastereoisomeric epoxide 9b was prepared via reduction of 13 to afford allylic alcohol 17 followed by sharpless epoxidation and oxidation with Dess–Martin periodinane.
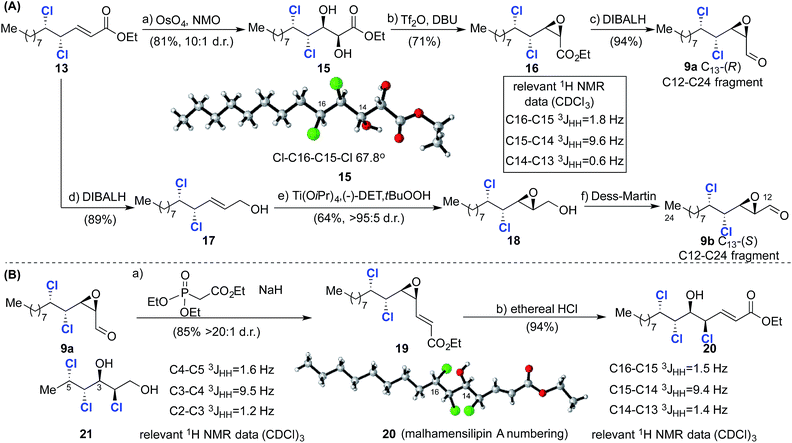 |
| Scheme 4 (A) Completion of the diastereoisomeric C12–C24 fragments 9a and 9b reagents and conditions: (a) 5 mol% OsO4, NMO, 2 : 1 acetone/H2O, r.t., 16 h, 81%, >10 : 1 d.r.; (b) Tf2O, DABCO, CH2Cl2, −78 °C to r.t., 16 h, 71%; (c) DIBALH, toluene, −78 °C, 1 h, 94%; (d) DIBALH, toluene, 0 °C, 15 min, 89%; (e) (−)-DET, Ti(Oi-Pr)4, t-BuOOH, CH2Cl2, −20 °C, 24 h, 64%; (f) Dess–Martin periodinane, CH2Cl2, r.t., 3 h. (B) Opening of the C13–C14 epoxide with inversion of configuration at C13 in a model system reagents and conditions: (a) NaH, THF, −78 °C to r.t., 2 h, 85%, >20 : 1 E/Z; (b) HCl·Et2O, 0 °C to r.t., 16 h, 94%. | |
Having established reliable and selective synthesis routes to the required C12–C24 aldehydes, we investigated the critical nucleophilic ring opening of the C13–C14 epoxide. Similar ring opening reactions had been observed to take place with retention5,7a and inversion of configuration at C13.8 In the first instance we investigated chlorohydrin formation on model compound 19, prepared from aldehyde 9avia a Horner–Wadsworth–Emmons reaction (85%, >20
:
1 d.r., Scheme 4B).
Treatment of 19 with ethereal HCl afforded chlorohydrin 20 as a single diastereoisomer, the stereochemistry of which was assigned via X-ray crystallography and 1H NMR analysis (Scheme 4B). These data confirm inversion of configuration at C13 and hence the formation of the desired syn/anti/syn stereotetrad. The stereochemistry of 20 could also be assigned using model compounds in Carreira's NMR database.17a
Thus, comparison of 20 with reference compound 2117a revealed very closely matching 3JHH coupling constants, highlighting the utility of the database approach and giving us confidence in its application to more complex fragments in the absence of X-ray crystallographic data. Following this encouraging result we focused on the synthesis of the less complex C1–C11 fragment (Scheme 5). To this end, PCC-mediated oxidation of 22 afforded aldehyde 23, which was subjected to pyrrolidine-catalyzed α,α-dichlorination using NCS.8 The highly sensitive aldehyde so obtained was immediately reduced to the corresponding alcohol to afford 24 in 93% isolated yield over the two steps. Installation of the required phenyltetrazolesulfonyl group was then accomplished in two straightforward steps after protection of the alcohol to afford compound 8.
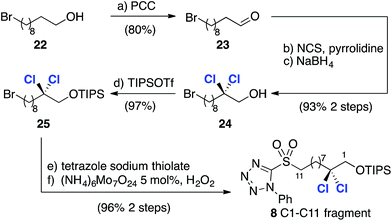 |
| Scheme 5 Synthesis of the C1–C11 fragment 8. Reagents and conditions: (a) PCC, CH2Cl2, r.t., 16 h, 80%; (b) NCS, 20 mol% pyrrolidine, 1,2-dichloroethane, 60 °C, 3 h; (c) NaBH4, EtOH, 0 °C to r.t., 1 h, 93% over two steps; (d) TIPSOTf, 2,6-lutidine, CH2Cl2, r.t., 2 h, 97%; (e) sodium 1-phenyl-1H-tetrazole-5-thiolate, DMF, r.t., 4 h, quantitative; (f) 5 mol% ammonium heptamolybdate, H2O2, EtOH, 0 °C to r.t., 16 h, 96%. | |
Fragment coupling and chlorohydrin formation
Our synthesis plan (Scheme 2C) is based on stereospecific chlorination reactions of epoxides to construct the C11–C16 stereohexad. Based upon this analysis, an E-selective olefination reaction was required for the fragment coupling and we selected the Julia–Kocienski reaction for this purpose.22 With the requisite fragments available in sufficient quantities, we examined the key olefination reaction using aldehyde 9a (Scheme 6). The olefination reaction was examined in detail23 beginning with the original conditions reported by Kocienski before investigating conditions reported by Jacobsen (LiHMDS, DMF/HMPA)24 and, finally, Popisil (KHMDS, 18-C-6).25 The optimized coupling process, from which alkene 7a was obtained in 86% yield and 4
:
1 d.r., is depicted in Scheme 6. Since 7a was inseparable from its Z-isomer, the key epoxide opening and introduction of the C13 chlorine substituent was investigated using the crude product. After some experimentation with alternative reagents,23 namely, ethereal HCl and thionyl chloride, we discovered that chlorotitanium isopropoxide in toluene was a very effective reagent for the conversion of γ-δ-chlorovinyl epoxides into chlorohydrins and product 26 was obtained in good yield and excellent stereoselectivity (Scheme 6). While this reagent has been used for ring opening reactions of hydroxyepoxides;17a it has not previously been applied to γ-δ-chlorovinyl epoxides and the high selectivity obtained is noteworthy. To assign the configuration at C13 we again compared the 3JHH coupling constants of the key C13–C16 region with two relevant (enantiomeric) fragments from Carreira's database (Scheme 6).17a We began by comparing the major chlorohydrin product 26 with model compound 21, which has the relative stereochemistry required for the natural product and corresponds to epoxide opening with inversion at C13. A significant discrepancy between the C13–C14 and C2–C3 3JHH values was observed. However, further comparison with model compound 27 revealed very closely matching coupling constants. Therefore, the stereochemistry was assigned as depicted in structure 26, which is the result of epoxide opening with retention of stereochemistry at C13. As noted above this outcome has been observed before, but contrasts with the result obtained with model compound 19 (Scheme 4B), pointing to a potential electronic effect (vide infra).
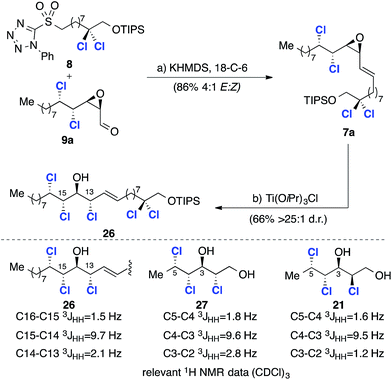 |
| Scheme 6 Fragment coupling and installation of C13 chlorine with retention of configuration reagents and conditions: (a) KHMDS, 18-crown-6, THF, −78 °C to r.t., 30 s, 86%, 4 : 1 E : Z; (b) TiCl(OiPr)3, C6H6, −20 °C, 66%, >25 : 1 d.r. | |
In order to confirm our stereochemical assignments and investigate the final epoxidation and deoxydichlorination sequence, compound 26 was taken forward (Scheme 7). Thus electrophilic epoxidation of 26 with mCPBA afforded a separable 1
:
1 mixture of epoxides 28 and 29 (Scheme 7). Since it was not possible to assign the configuration at C11 or C12 the stereochemical assignment was carried out retrospectively after successful deoxydichlorination of both epoxides (Scheme 7).
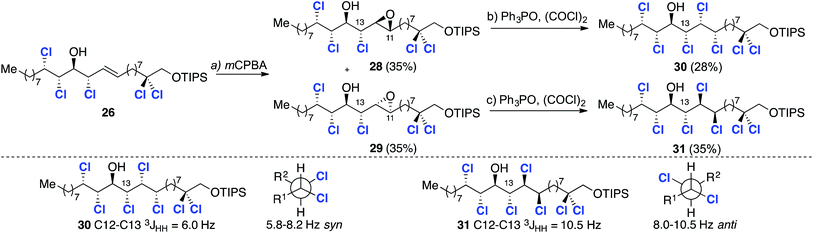 |
| Scheme 7 Final epoxidation/deoxydichlorination and synthesis of diastereoisomeric analogs reagents and conditions: (a) mCPBA, CH2Cl2, 0 °C to r.t., 70% combined yield, 1 : 1 29 and 30; (b) 1.5 equiv. Ph3PO, 1.5 equiv. (COCl)2, toluene, 90 °C, 3 h, 27%; (c) 1.5 equiv. Ph3PO, 1.5 equiv. (COCl)2, toluene, 90 °C, 3 h, 35%. | |
This transformation occurred in 28% and 36% isolated yield for epoxides 28 and 29 respectively. In each case by-products arising from elimination were also observed. Given that the deoxydichlorination reaction takes place with inversion of configuration at both stereogenic centers of the epoxide, the C11–C12 relative stereochemistry in both compounds was assumed to be syn. To determine the relative configuration between the chlorines at C12 and C13 the 3JHH coupling constants were examined (Scheme 7). Based upon Carreira's previous study,17a a value of 6.0 Hz is indicative of a syn relationship between C13 and C12 and, therefore, compound 30 was assigned as having a syn,anti,anti,syn,syn stereohexad. The higher value of 10.5 Hz is characteristic of an anti relationship between the C13 and C12 chlorine substituents and hence a syn,anti,anti,anti,syn stereohexad, as depicted in structure 31. While not providing the correct stereochemistry required for malhamensilipin A, these studies established the viability of our synthesis plan based upon a threefold epxoditation/chlorination approach. We next sought to correct the stereochemistry at C13 in order to complete a synthesis of malhamensilipin A.
Completion of the synthesis
Given that the C13 epoxide opening had been observed to take place with stereoretention using TiCl(OiPr)3, we progressed the alternative C12–C24 fragment 9b with inverted C13 stereochemistry (Scheme 8). To this end, coupling with the C1–C11 sulfone 8 under the previously identified conditions afforded intermediate 7b with improved selectivity (7
:
1 d.r.), but in lower yield – a consequence of the relative instability of aldehyde 9b compared to 9a. The critical epoxide opening reaction was then investigated (Scheme 8). We assumed that in this stereochemical series the epoxide opening would again take place with retention of configuration at C13 when using TiCl(OiPr)3 and this was confirmed by comparison of 32 with model compounds 21 and 2717a (Scheme 8). Subsequent epoxidation of 32 with mCPBA (Scheme 9) gave two separable epoxides 6a and 6b in a 2
:
1 ratio. Again, stereochemical assignments of these key intermediates were performed retrospectively after the introduction of the final two chlorines. First, we subjected the minor diastereoisomer to deoxydichlorination and obtained the corresponding heptachlorinated compound 33 in good yield (Scheme 9).
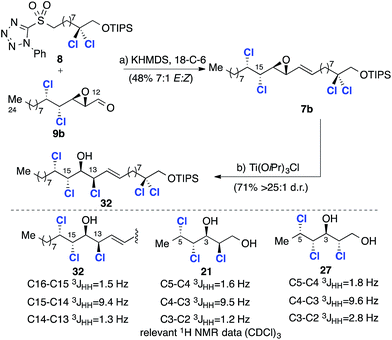 |
| Scheme 8 Synthesis of the carbon skeleton with inverted configuration at C13 reagents and conditions: (a) KHMDS, 18-crown-6, THF, −78 °C, 30 s, then 10b, −78 °C to r.t., 16 h, 48% from 19, 7 : 1 E/Z; (b) TiCl(Oi-Pr)3, toluene, −20 °C, 2 h, 71%, >25 : 1 d.r. | |
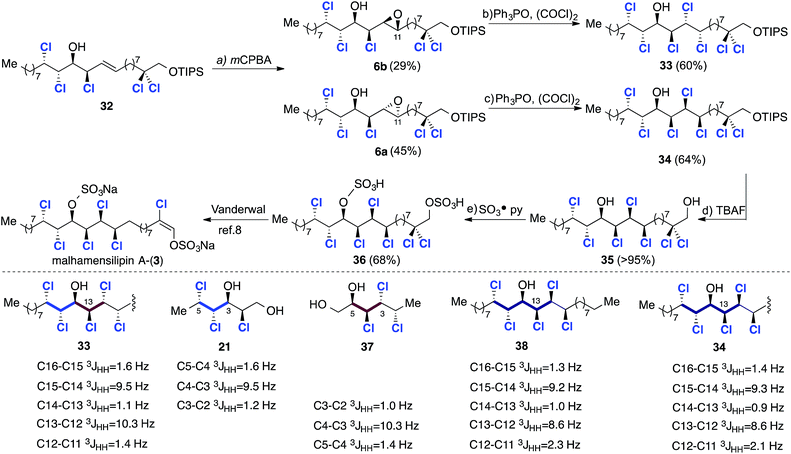 |
| Scheme 9 Completion of a formal synthesis of malhamensilipin A reagents and conditions: (a) mCPBA, CH2Cl2, 0 °C to r.t., 24 h, 74%, 2 : 1 7a : 7b; (b) 1.5 eq. Ph3PO, 1.5 eq. (COCl)2, toluene, 90 °C, 3 h, 64%; (c) 1.5 eq. Ph3PO, 1.5 eq. (COCl)2, toluene, 90 °C, 3 h 60% (d) TBAF, AcOH, THF, r.t., 16 h, quantitative; (e) SO3-pyridine, DMF, r.t., 1 h, 68%. | |
This final deoxydichlorination process is noteworthy for two reasons: (a) the reaction is chemoselective – the C14 hydroxyl group does not undergo deoxychlorination; and (b) the introduction of chlorine at C12 occurs despite the presence of the flanking chlorines at C13 and C11. These observations demonstrate that a combination of steric and electronic effects prevents activation of the C14 hydroxyl group by the electrophilic chlorophosphonium salt.
The configuration of 33 was deduced by comparison with models 21 and 37,17a which contain matching stereotriads (highlighted in blue and red).
The close match of the relevant vicinal coupling constants are congruous with the non-natural syn/anti/syn/anti/syn stereochemistry depicted in structure 33. With this analysis in mind, deoxydichlorination of the major epoxide 6a was undertaken with the expectation that the correct syn/anti/syn/syn/syn stereochemistry would result. The heptachlorinated material 34 was obtained in good yield and compared very favourably with model compound 38, which contains an identical stereohexad.4f Further spectroscopic comparison of 34 with Vanderwal's TBDMS protected analogue8 confirmed the desired stereochemistry.
In order to complete a formal total synthesis of malhamensilipin A the silyl ether protecting group was removed in excellent yield using TBAF to afford diol 35, which was subsequently converted into diacid 36 using sulfur trioxide pyridine complex. Compound 36 had previously been prepared by Vanderwal and was the penultimate intermediate in his total synthesis of the natural product.8 All spectroscopic data for 36 were in excellent agreement with those previously reported, thereby completing a 12-step formal synthesis of malhamensilipin A (3).
Stereoretentive ring opening reactions of γ- and δ-dichlorovinylepoxides: the intermediacy of chloronium ion intermediates
The synthesis described in the previous sections, along with several other chlorosulfolipid syntheses, relied upon nucleophilic opening of γ,δ-dichlorovinylepoxide intermediates for the introduction of the chlorohydrin motif. The stereochemical outcome of such reactions has been shown to occur with both retention (e.g.7a → 26, Scheme 6 and 7b → 32, Scheme 8) and inversion (e.g.19 → 20, Scheme 4B). Stereoretentive reactions were first observed and documented by Carreira during the synthesis of mytilipin A5 and have subsequently been observed by Vanderwal.7a Carreira has proposed that retention of configuration occurs as a result of a double inversion process in which anchimeric assistance from either the γ- or δ-chlorine substituent affords a chloronium ion intermediate, which itself undergoes subsequent nucleophilic substitution.5 Carreira's analysis can be transferred to the ring opening reactions that were discussed in the previous section of this Article (Scheme 10).
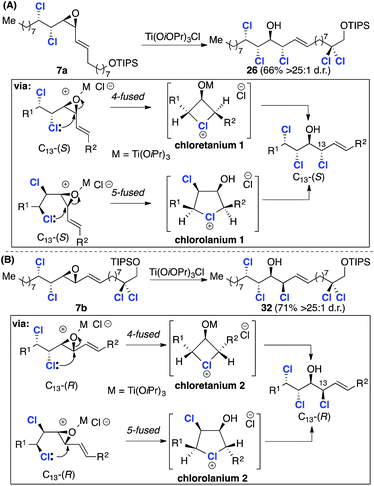 |
| Scheme 10 Intervention of chloronium ions in epoxide opening reactions of 7a and 7b. | |
In each case there are two potential chloronium ion intermediates arising from attack of either the γ- or δ-chlorine substituent on C13 in either a 5 or 4-fused26 (endo) sense, respectively. Two further possible chloronium ions arising from attack at C12 in either a 4- or 3-spiro (exo) cyclisation modes have not been considered as the products arising from these modes of attack were not isolated, presumably as a result of the alkene activating C13 with respect to nucleophilic attack.27 The observed retention with both7a and 7b coupled with numerous elegant studies on chloronium species, including cyclic structures,28,29 makes this a compelling explanation. More recent work by Carreira30 on the ring opening reactions of a collection of γ- and δ-chloro vinyl epoxides has provided further results that are consistent with the intervention of four-(chloretanium) and five-(chlorolanium) membered ring ions. In comparing the early work of Peterson and Olah28,29 with recent studies we noted that all of the substrates that have been investigated in the context of chlorosulfolipid synthesis are vinyl epoxides. Given that the alkene substituent would be expected to influence the structure of any derived chloronium ion intermediates we carried out a theoretical study to investigate the structures and energies of the unsaturated cyclic chloronium ions depicted in Scheme 10.31
We began our computational investigation with a comparison of the simple dipropyl substituted chloronium ion 39 with its unsaturated analogy 40. Equilibrium geometries for these two species were optimized using a variety of methods at the wavefunction and density functional level of theory (Table 1), with implicit solvation by an SMD description of toluene.32
Table 1 Comparison of alkyl and allyl substituted chloronium ions (BS1 = def2-TZVPP; BS2 = def2-QZVPP)

|
Entry |
Level of theory |
r
C–Cl
39 (Å) |
r
C–allyl
40 (Å) |
r
C–alkyl
40 (Å) |
Δr (Å) |
ΔE (kcal mol−1) |
1 |
B3YLP/BS1 |
1.89 |
2.00 |
1.88 |
0.12 |
11.2 |
2 |
B3LYP-D3/BS1 |
1.89 |
1.98 |
1.88 |
0.10 |
14.8 |
3 |
ωB97XD/BS1 |
1.85 |
1.90 |
1.85 |
0.05 |
14.9 |
4 |
M06-2X/BS1 |
1.85 |
1.89 |
1.85 |
0.04 |
16.8 |
5 |
M11/BS1 |
— |
1.89 |
1.85 |
0.04 |
16.2 |
6 |
M11-L/BS1 |
1.83 |
2.54 |
1.80 |
0.74 |
8.1 |
7 |
MP2/BS1 |
1.84 |
1.87 |
1.84 |
0.03 |
— |
8 |
B2-PLYP-D3/BS1 |
1.87 |
1.93 |
1.86 |
0.07 |
— |
9 |
B3LYP/BS2 |
1.89 |
2.01 |
1.88 |
0.13 |
— |
10 |
B3LYP-D3/BS2 |
1.89 |
1.99 |
1.88 |
0.11 |
— |
11 |
ωB97XD/BS2 |
1.86 |
1.90 |
1.85 |
0.05 |
— |
12 |
M06-2X/BS2 |
1.86 |
1.89 |
1.85 |
0.04 |
— |
13 |
M11/BS2 |
— |
1.90 |
1.85 |
0.05 |
— |
14 |
M11-L/BS2 |
1.84 |
1.94 |
1.83 |
0.11 |
— |
A recent study by Stoyanov and Reed, in which X-ray structures of dimethyl and diethyl chloronium salts were obtained,33 allowed us to compare computed and experimentally determined C–Cl bond lengths. The experimental C–Cl length in the diethylchloronium ion is 1.840 Å. A survey of different methods and basis sets (BS1 = def2-TZVPP, BS2 = def2-QZVPP) found that B3LYP, B3LYP-D3, B2PLYP overestimate this distance. However, ωB97XD and the Minnesota functionals provide a good description of the chloronium structure. The MP2/BS1 and M11-L/BS2 derived bond lengths of 1.84 Å were in excellent agreement with experiment. Smaller basis sets, such as 6-31+G(d) gave routinely worse results (see ESI†). We next examined the unsymmetrical allyl/propyl chloronium ion using the same theoretical models (Table 1). This model compound allows for a comparison of C–Cl bond lengths, and, therefore, to establish the extent of chloronium versus carbocationic character in these structures. All of the theoretical methods tested show that the allylic group interacts more weakly than the alkyl group, based on a longer C–Cl bond distance. The computed variation between alkyl and allyl distances ranges from 0.04–0.13 Å. The M11-L/def2-TZVPP (entry 6) optimized structure is an outlier, consistent with a carbocation (see Fig. 1A) with a r(C–allyl) distance 2.54 Å and bond lengths of 1.35 Å and 1.39 Å in the allylic fragment. However, all other methods generated a chloronium like structure. At all levels the calculated dissociation energy of 40 into allyl cation and propylchloride fragments (ΔE, Table 1) is positive by at least 8 kcal mol−1, signifying a stabilizing interaction between the chlorine and the allylic carbon atom even in the structures with longer (C–allyl) distances.
We returned to the ring opening reactions of epoxides 7a and 7b (Scheme 10). Taking epoxide 7a first, we optimized the SMD-M06-2X/def-TZVPP geometries for truncated analogues of the two putative chloronium ion intermediates, chloretanium 1 and chlorolanium 1 (Fig. 1A) derived from anchimeric assistance of either the γ- or δ-chlorine substituents, respectively. Inspection of the geometry of chloretanium 1 reveals: (a) a long Cl-allyl distance of 2.69 Å; (b) similar bond lengths of 1.39 Å and 1.36 Å within the allylic fragment; and (c) planar allylic carbon atoms, as quantified by the extent of pyramidalization. These data are consistent with a distorted chloretanium ion with significant carbocationic character. In contrast, chlorolanium 1 has a substantially shorter carbon–chlorine bond length (1.90 Å) and substantial pyramidalization of the allylic carbon, consistent with a cyclic chloronium ion. The same pattern was seen with chloretanium 2 and chlorolanium 2, which arise from the diastereoisomeric epoxide 7b. The relative energy of the carbocation-like chloretanium structures was, in each case, significantly higher by more than 8 kcal mol−1 compared to the corresponding chlorolanium ion (Fig. 1).
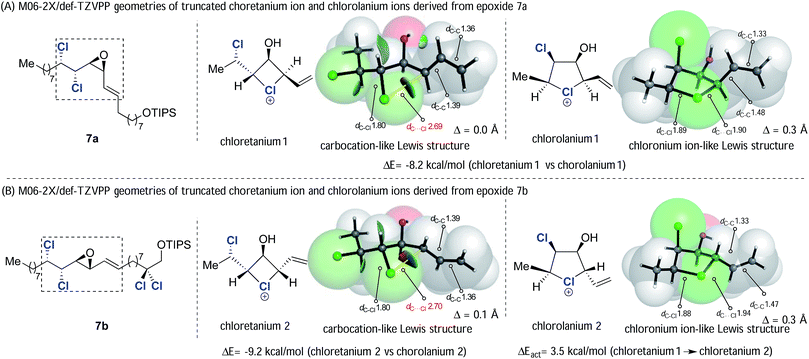 |
| Fig. 1 SMD-M06-2X/def2-TZVPP optimized structures of model chloronium ions. Bond distances and pyramidalization (Δ) in Å, with NCI isosurface shown for the chloretanium structures. | |
While chloretanium intermediates were found to be less stable, with greater carbocationic character than the chlorolanium species, there is still an interaction between Cl and the allylic carbon. The C–Cl distance in these unsymmetrical intermediates (2.69–2.70 Å) is considerably shorter than the sum of van der Waals radii of 3.45 Å (Bondi) or 3.52 Å (Pauling). The presence of a through-space, weakly attractive C–Cl interaction in the chloretanium is also apparent from a NBO-computed Wiberg bond order34 of 0.06, and the Non-Covalent Interaction (NCI) isosurface shown in Fig. 1.35 This interaction is much weaker than the corresponding bond order obtained for the chlorolanium species (0.89–0.92), and a fairly small barrier of 3.5 kcal mol−1 to rotate about the C13–C14 bond was found for chloretanium 1. It is fair to summarise that the four and five-membered chloronium intermediates lie along a continuum from free carbocation to chloronium-like Lewis structures, rather than an abrupt shift in the nature of bonding.
With these computational data in mind, we return to the stereoretentive titanium-mediated epoxide ring opening reactions depicted in Scheme 10. We suggest that these reactions take place through a pair of diastereoisomeric chlorolanium-like intermediates (chlorolanium 1 and chlorolanium 2, Scheme 10) rather than the higher energy distorted chloretanium ions. Based upon the computed geometries of the truncated analogues the chlorolanium-like intermediates are likely to have unequal carbon–chlorine bond distances and exhibit a degree of carbocationic character at C13. We note that the epoxide opening of model compound 19 (Scheme 4B), which contained an electron withdrawing ester group took place with inversion of stereochemistry. This result corroborates the development of partial cationic character at C13 during reactions that take place with retention of configuration. Finally, it should be noted that high levels of stereoretention have been observed in systems in which the formation of a chlorolanium ion is not possible e.g. δ-chloro vinyl epoxides.30 In such cases it is likely that distorted chloretanium ions are involved and that the chlorine substituent blocks one face of the allylic subunit leading to high levels of stereoretention.
Conclusion
In summary, we have accomplished a 12-step convergent, enantioselective formal synthesis of malhamensilipin A from commercial (E)-2-undecenal. The synthesis strategy was based upon an iterative epoxidation/deoxydichlorination sequence, which demonstrates the power of phosphorus(V)-mediated deoxydichlorination reactions of epoxides for the stereospecific and chemoselective construction of chlorine-containing stereochemical arrays. In addition to the formal synthesis, we have prepared three further diastereoisomeric heptachlorinated analogues and demonstrated the utility of Carreira's NMR database for the stereochemical assignment of complex polychlorinated entities. Our computational analysis has provided new insight into the stereoretentive ring-opening reactions of γ,δ-dichloro vinyl epoxides. Specifically, we have corroborated the intervention of chloronium ion intermediates both experimentally and computationally. Furthermore, the intermediate chloretanium ions have been shown to be highly distorted with pronounced carbocationic character, making them less stable than the chlorolaniums. Despite this, computations do support the existence of a weakly attractive C–Cl interaction in the four-membered intermediate. The structures of both cyclic intermediates exist along a continuum of Lewis structures from free carbocation to chloronium, such that anchimeric assistance can occur in both cases.
Acknowledgements
RMD acknowledges financial support from the School of Chemistry, University of Nottingham (Studentship to JS) and the support of analytical services within the School of Chemistry. RSP acknowledges the use of the EPSRC UK National Service for Computational Chemistry Software (CHEM773).
Notes and references
- For a comprehensive review on halogen-containing natural products, see:
(a) G. W. Gribble, Prog. Chem. Org. Nat. Prod., 2010, 91, 1–613 CAS. For other recent elegant studies on halogenated natural products, see
(b) S. A. Snyder, A. P. Brucks, D. S. Treitler and I. Moga, J. Am. Chem. Soc., 2012, 134, 17714 CrossRef CAS PubMed;
(c) D. C. Braddock, A. X. Gao, A. J. P. White and M. Whyte, Chem. Commun., 2014, 50, 13725–13728 RSC;
(d) D. C. Braddock and D. T. Sbircea, Chem. Commun., 2014, 50, 12691–12693 RSC;
(e) H. M. Sheldrake, C. Jamieson, S. I. Pasca and J. W. Burton, Org. Biomol. Chem., 2009, 7, 238–234 RSC.
- For reviews the chlorosulfolipid family of natural products, see:
(a) D. K. Bedke and C. D. Vanderwal, Nat. Prod. Rep., 2011, 28, 15–25 RSC;
(b) C. Nilewski and E. M. Carreira, Eur. J. Org. Chem., 2012, 1685–1698 CrossRef CAS;
(c) T. Umezawa and F. Matsuda, Tetrahedron Lett., 2014, 55, 3003–3012 CrossRef CAS;
(d) W.-J. Chung and C. D. Vanderwal, Acc. Chem. Res., 2014, 47, 718–728 CrossRef CAS PubMed.
-
(a) J. Elovson and P. R. Vagelos, Proc. Natl. Acad. Sci. U. S. A., 1969, 62, 957–963 CrossRef CAS PubMed;
(b) T. H. Haines, M. Pousada, B. Stern and G. L. Mayers, Biochem. J., 1969, 113, 565–566 CrossRef CAS PubMed;
(c) T. H. Haines, M. Pousada, B. Stern and G. L. Mayers, Biochem. J., 1969, 113, 565–566 CrossRef CAS PubMed;
(d) J. Elovson and P. R. Vagelos, Biochemistry, 1970, 9, 3110–3126 CrossRef CAS PubMed;
(e) J. Elovson, Biochemistry, 1974, 13, 2105–2109 CrossRef CAS PubMed;
(f) J. Elovson, Biochemistry, 1974, 13, 3483–3487 CrossRef CAS PubMed;
(g) C. L. Mooney, E. M. Mahoney, M. Pousada and T. H. Haines, Biochemistry, 1972, 11, 4839–4844 CrossRef CAS PubMed;
(h) C. L. Mooney and T. H. Haines, Biochemistry, 1973, 12, 4469–4472 CrossRef CAS PubMed;
(i) G. Thomas and E. I. Mercer, Phytochemistry, 1974, 12, 4469–4472 Search PubMed.
-
(a) P. Ciminiello, E. Fattorusso, M. Forino, M. Di Rosa, A. Ianaro and R. Poletti, J. Org. Chem., 2001, 66, 578–582 CrossRef CAS PubMed;
(b) P. Ciminiello, C. Dell’Aversano, E. Fattorusso, M. Forino, S. Magno, M. Di Rosa, A. Ianaro and R. Poletti, J. Am. Chem. Soc., 2002, 124, 13114–13120 CrossRef CAS PubMed;
(c) P. Ciminiello, C. Dell'Aversano, E. Fattorusso, M. Forino, S. Magno, P. Di Meglio, A. Ianaro and R. Poletti, Tetrahedron, 2004, 60, 7093–7098 CrossRef CAS;
(d) T. Kawahara, Y. Kumaki, T. Kamada, T. Ishii and T. Okino, J. Org. Chem., 2009, 74, 6016–6024 CrossRef CAS PubMed;
(e) J. L. Chen, P. J. Proteau, M. A. Roberts and W. H. Gerwick, J. Nat. Prod., 1994, 57, 524–527 CrossRef CAS PubMed;
(f) A. R. Pereira, T. Byrum, M. Shibuya, C. D. Vanderwal and W. H. Gerwick, J. Nat. Prod., 2010, 73, 279–283 CrossRef CAS PubMed;
(g) C.-H. Chao, H.-C. Huang, G. H. Wang, Z.-H. Wen, W.-H. Wang, I.-M. Chen and J.-H. Sheu, Chem. Pharm. Bull., 2010, 58, 944–946 CrossRef CAS PubMed.
- C. Nilewski, R. W. Geisser and E. M. Carreira, Nature, 2009, 457, 573–577 CrossRef CAS PubMed.
-
(a) T. Yoshimitsu, N. Fukumoto, R. Nakatani, N. Kojima and T. Tanaka, J. Org. Chem., 2010, 75, 5425–5437 CrossRef CAS PubMed;
(b) W.-J. Chung, J. S. Carlson, D. K. Bedke and C. D. Vanderwal, Angew. Chem., Int. Ed., 2013, 52, 10052–10055 CrossRef CAS PubMed;
(c) W.-J. Chung, J. S. Carlson and C. D. Vanderwal, J. Org. Chem., 2014, 79, 2226–2241 CrossRef CAS PubMed.
-
(a) D. K. Bedke, G. M. Shibuya, A. Pereira, W. H. Gerwick, T. H. Haines and C. D. Vanderwal, J. Am. Chem. Soc., 2009, 131, 7570–7572 CrossRef CAS PubMed;
(b) T. Yoshimitsu, R. Nakatani, A. Kobayashi and T. Tanaka, Org. Lett., 2011, 13, 908–911 CrossRef CAS PubMed;
(c) T. Umezawa, M. Shibata, K. Kaneko, T. Okino and F. Matsuda, Org. Lett., 2011, 13, 904–907 CrossRef CAS PubMed;
(d) S. Fischer, N. Huwyler, S. Wolfrum and E. M. Carreira, Angew. Chem., Int. Ed., 2016, 55, 2555 CrossRef CAS PubMed;
(e) A. R. White, B. M. Duggan, S.-C. Tsai and C. D. Vanderwal, Org. Lett., 2016, 18, 1124 CrossRef CAS PubMed.
- D. K. Bedke, G. M. Shibuya, A. R. Pereira, W. H. Gerwick and C. D. Vanderwal, J. Am. Chem. Soc., 2010, 132, 2542–2543 CrossRef CAS PubMed.
- C. Nilewski, N. R. Deprez, T. C. Fessard, D. B. Li, R. W. Geisser and E. M. Carreira, Angew. Chem., Int. Ed., 2011, 50, 7940–7943 CrossRef CAS PubMed.
-
(a) G. M. Shibuya, J. S. Kanady and C. D. Vanderwal, J. Am. Chem. Soc., 2008, 130, 12514–12518 CrossRef CAS PubMed;
(b) J. S. Kanady and J. D. Nguyen, J. Org. Chem., 2009, 74, 2175–2178 CrossRef CAS PubMed.
-
(a) M. L. Landry, D. X. Hu, G. M. McKenna and N. Z. Burns, J. Am. Chem. Soc., 2016, 138, 5150 CrossRef CAS PubMed; for further work, see:
(b) D. X. Hu, G. M. Shibuya and N. Z. Burns, J. Am. Chem. Soc., 2013, 135, 12960–12963 CrossRef CAS PubMed;
(c) D. X. Hu, F. J. Seidl, C. Bucher and N. Z. Burns, J. Am. Chem. Soc., 2015, 137, 3795–3798 CrossRef CAS PubMed;
(d) C. Bucher, R. M. Deans and N. Z. Burns, J. Am. Chem. Soc., 2015, 137, 12784–12787 CrossRef CAS PubMed.
- S. A. Snyder, Z.-Y. Tang and R. Gupta, J. Am. Chem. Soc., 2009, 131, 5744–5745 CrossRef CAS PubMed.
- K. C. Nicolaou, N.-L. Simmons, Y. Ying, P. M. Heretsch and J. S. Chen, J. Am. Chem. Soc., 2011, 133, 8134–8137 CrossRef CAS PubMed.
- A. J. Cresswell, S. T.-C. Eey and S. E. Denmark, Nat. Chem., 2015, 7, 146–152 CrossRef CAS PubMed.
-
(a) T. Yoshimitsu, N. Fukumoto and T. Tanaka, J. Org. Chem., 2009, 74, 696–702 CrossRef CAS PubMed; for the original reaction, see
(b) N. S. Isaacs and D. Kirkpatrick, Tetrahedron Lett., 1972, 13, 3869–3870 CrossRef.
-
(a) R. M. Denton, X. Tang and A. Przeslak, Org. Lett., 2010, 12, 4678–4681 CrossRef CAS PubMed;
(b)
S. P. Marsden in, P. J. Dunn; K. K. Hii; M. J. Krische and M. T. Williams, Sustainable Catalysis: Challenges and Practices for the Pharmaceutical and Fine Chemical Industries, Wiley, New York, 1st edn, 2013, p. 440 Search PubMed.
-
(a) C. Nilewski, R. W. Geisser, M.-O. Ebert and E. M. Carreira, J. Am. Chem. Soc., 2009, 131, 15866–15876 CrossRef CAS PubMed; Kishi has designed and developed a universal NMR database for the assignment of polyketides, for proof-of-concept see:
(b) J. Lee, Y. Kobayashi, K. Tezuka and Y. Kishi, Org. Lett., 1999, 1, 2181 CrossRef CAS PubMed.
- G. L. Zhao, I. Ibrahem, H. Sunden and A. Cordova, Adv. Synth. Catal., 2007, 349, 1210–1224 CrossRef CAS.
- M. Marigo, J. Franzen, T. B. Poulsen, W. Zhuang and K. A. Jorgensen, J. Am. Chem. Soc., 2005, 127, 6964–6965 CrossRef CAS PubMed.
- The competing elimination has been observed in related reactions by us (ref. 16) as well as Tanaka and Yoshimitsu (ref. 15a).
- Epoxide 9a had been previously described by Vanderwal and co-workers during the first total synthesis of malhamensilipin A, see ref. 8.
- P. R. Blakemore, W. J. Cole, P. J. Kocienski and A. Morley, Synlett, 1998, 26–28 CrossRef CAS.
- Full details can be found in the ESI.†.
- P. Liu and E. N. Jacobsen, J. Am. Chem. Soc., 2001, 123, 10772–10773 CrossRef CAS PubMed.
- J. Pospisil, Tetrahedron Lett., 2011, 52, 2348–2352 CrossRef CAS.
- The designation of “fused” and “spiro” mode cyclizations in place of endo and exo has been used to describe ring-opening reactions of cyclopropanes and, more recently, to describe epoxide opening reactions, see I. Vilotijevic and T. F. Jamison, Science, 2007, 317, 1189–1192 CrossRef CAS PubMed . This terminology avoids confusion since the carbon–oxygen bond breaks outside the ring in both the endo and exo ring opening reactions.
- For endo-selective ring opening reactions of vinyl epoxides, see
(a) K. C. Nicolaou, C. V. C. Prasad, P. K. Somers and C.-K. Hwang, J. Am. Chem. Soc., 1989, 111, 5330–5334 CrossRef CAS; for a review recent on vinyl epoxides in organic synthesis, see
(b) J. He, J. Ling and P. Chiu, Chem. Rev., 2014, 114, 8037–8128 CrossRef CAS PubMed.
-
(a) G. A. Olah, J. M. Bollinger and J. Brinich, J. Am. Chem. Soc., 1968, 90, 2587–2594 CrossRef CAS;
(b) G. A. Olah and P. E. Peterson, J. Am. Chem. Soc., 1968, 90, 4675–4678 CrossRef CAS;
(c) G. A. Olah, P. W. Westerman, E. G. Melby and Y. K. Mo, J. Am. Chem. Soc., 1974, 96, 3565–3573 CrossRef CAS;
(d) G. A. Olah, G. K. Prakash and G. Rasul, Proc. Natl. Acad. Sci. U. S. A., 2013, 110, 8427 CrossRef CAS PubMed;
(e)
G. A. Olah, K. K. Laali, Q. Wang and G. K. S. Prakash, Onium Ions, Wiley, New York, 1998, ch. 6 Search PubMed.
-
(a) P. E. Peterson, R. J. Bopp, M. C. Dhansukh, E. L. Curran, D. Dillard and R. J. Kamat, J. Am. Chem. Soc., 1967, 89, 5902–5911 CrossRef CAS;
(b) P. E. Peterson, J. M. Indelicato and R. R. Bonazza, Tetrahedron Lett., 1971, 12, 13–16 CrossRef.
- A. Shemet, D. Sarlah and E. M. Carreira, Org. Lett., 2015, 17, 1878–1881 CrossRef CAS PubMed.
-
M. J. Frisch, et al., Quantum chemical calculations were performed with Gaussian09: Gaussian 09, Revision D.01, Gaussian, Inc., Wallingford CT, 2009 Search PubMed, Full computational details
and their supporting references are given in the ESI†.
- A. V. Marenich, C. J. Cramer and D. G. Truhlar, J. Phys. Chem. B, 2009, 113, 6378–6396 CrossRef CAS PubMed.
- E. S. Stoyanov, I. V. Stoyanova, F. S. Tham and C. A. Reed, J. Am. Chem. Soc., 2010, 132, 4062–4063 CrossRef CAS PubMed.
-
E. D. Glendening, J. K. Badenhoop, A. E. Reed, J. E. Carpenter, J. A. Bohmann, C. M. Morales, C. R. Landis and F. Weinhold, NBO 6.0., Theoretical Chemistry Institute, University of Wisconsin, Madison, 201 Search PubMed.
-
(a) J. Contreras-Garcia, E. R. Johnson, S. Keinan, R. Chaudret, J.-P. Piquemal, D. Beratan and W. Yang, J. Chem. Theory Comput., 2011, 7, 625 CrossRef CAS PubMed;
(b) E. R. Johnson, S. Keinan, P. Mori-Sanchez, J. Contreras-Garcia, A. Cohen and W. Yang, J. Am. Chem. Soc., 2010, 132, 6498 CrossRef CAS PubMed.
Footnote |
† Electronic supplementary information (ESI) available. CCDC 1470484 and 1470483. For ESI and crystallographic data in CIF or other electronic format see DOI: 10.1039/c6sc03012b |
|
This journal is © The Royal Society of Chemistry 2016 |
Click here to see how this site uses Cookies. View our privacy policy here.