rBMSC and bacterial responses to isoelastic carbon fiber-reinforced poly(ether-ether-ketone) modified by zirconium implantation
Received
28th August 2015
, Accepted 17th November 2015
First published on 18th November 2015
Abstract
PEEK-based biomaterials have great potential applications as hard tissue substitutes in bone tissue engineering. However, inherent bio-inert properties limited their clinical use. In order to improve the bioactivity, in this work, zirconium ions were implanted into the carbon fiber-reinforced PEEK (CFR-PEEK) using plasma immersion ion implantation (PIII) technology. Surface morphologies and chemical compositions of Zr-PIII treated samples were analyzed by scanning electron microscopy (SEM), X-ray photoelectron spectroscopy (XPS), respectively. The results indicated that nanostructures and ZrO2 nanoparticles were formed on the surface of CFR-PEEK after Zr-PIII. Mechanical tests revealed that nanohardness, elastic modulus, and elastic resistance increased after implantation, especially for the elastic modulus with a maximum value of about 14 GPa, which is much close to that of human natural bone. In vitro cellular experiments showed that Zr-PIII treated samples enhanced the initial adhesion of rBMSCs, spreading and proliferation significantly. Moreover, the heightened ALP activity, collagen secretion, and extracellular matrix mineralization suggested that Zr-PIII treatment could greatly lead to an up-regulated osteogenic differentiation of rBMSCs on CFR-PEEK. In addition, antibacterial properties were also investigated and the results showed that Zr-PIII treated CFR-PEEK with nanostructures exhibited obvious antibacterial activity against S. aureus but no effect on E. coli.
1. Introduction
Poly(ether-ether-ketone) (PEEK) has attracted more and more attention as an alternative material in hard tissue engineering because of its excellent mechanical properties, chemical stability, as well as good biocompatibility.1–4 Compared with metallic implants such as titanium and its alloys, PEEK has a lower elastic modulus which can be tailored close to that of human bone, resulting in alleviated bone absorption and bone loss, which was usually known as stress shielding due to the mismatch in the modulus between implants and host bone.2 Moreover, PEEK has excellent corrosion resistance and non-degradation in the harsh environment of the human body, and there is no toxic substance release as a result of its chemical stability.5,6 Additionally, PEEK belongs to radiolucent materials and its compatibility with modern imaging technologies such as computer tomography (CT) and magnetic resonance imaging (MRI) provides favorable advantages for medical diagnosis.
However, PEEK is a non-bioactive material and its bio-inert surface is unfavorable for cell adhesion and growth.7–9 Moreover, PEEK has inferior ability to induce new bone formation in vivo so that PEEK implants can hardly bind or interlock with host bone directly and firmly.10–12 Long term stability of PEEK implants in the human body is still a challenging problem that impedes their clinical use extensively.
Many physical and chemical methods have been applied to treat PEEK materials for improving their bioactivity.8 Among these methods, plasma immersion ion implantation (PIII) is a fast and versatile surface modification technology which can modulate surface physical and chemical properties of biomaterials without damaging the bulk material.13 During the PIII process, high kinetic energy ions bombard the surface of PEEK and complex physical and chemical reactions, such as surface activation and crosslinking, chain degradation, surface ablation and etching, will occur.14–16 As a result, nanostructures and functional groups can be formed on the surfaces and this will lead to a favorable effect on cell responses to PEEK. It was reported that ravined nanostructures and hydroxyl groups could be introduced on the surface of PEEK after water PIII treatment and osteoblast responses, such as adhesion, spreading, proliferation, and osteogenic differentiation, were up-regulated in vitro.17 Moreover, the CFR-PEEK and PEEK with nanostructures were respectively fabricated by titanium and tantalum implantation and enhanced rat bone mesenchymal stem cell (rBMSC) responses in vitro and tissue reaction in vivo were also reported.18–20
Zirconia (ZrO2) ceramics possesses good biocompatibility, and have been widely used in dental, orthopedic, and bone repair.21 Simulated body fluid (SBF) immersion studies indicated that nanostructured ZrO2 and ZrO2 films/coatings had good bioactivity due to their excellent bone-like apatite-induced ability.22–25 It was reported that ZrO2 did not present any signs of toxic, immune, carcinogenic and oncogenic activities in vitro.26,27 Moreover, nanostructured ZrO2 and ZrO2 films/coatings could enhance bone marrow mesenchymal stem cell (BMMSC) and osteoblast responses such as adhesion, proliferation and mineralization, thus indicating that ZrO2 had good bioactivity and cytocompatibility.24,25,28–31 And besides, the amorphous ZrO2 films produced by cathodic arc deposition could enhance ALP activity and the expression of osteogenic marker genes, such as ALP, collagen I and osteocalcin.32,33 In addition, in vivo studies indicated that ZrO2 had a high affinity for bone tissue and ZrO2 modified implants exhibited excellent osseous integration and direct bone contact could be formed between the bone-implant interface.34–36 These results indicated that ZrO2 had good osteointegration. Finally, it was reported that ZrO2 nanoparticles had antibacterial properties against Gram negative and positive microorganisms.37,38
Our previous study indicated that nanopores could be formed on the CFR-PEEK surface after zirconium plasma immersion ion implantation (Zr-PIII) at a high implantation voltage (30 kV).19 However, the surface modification of CFR-PEEK by Zr-PIII at a low implantation voltage was still rarely reported. In this work, Zr-PIII was conducted to introduce bioactive ZrO2 on the CFR-PEEK surface. The physical, mechanical, and chemical properties were investigated and corresponding effects on rBMSC responses and antibacterial activity were also assessed in vitro.
2. Experimental
2.1. Sample preparation
Raw carbon fiber-reinforced poly(ether-ether-ketone) (CFR-PEEK) samples with dimension ∅ 120 mm × 1 mm were machined from biomedical grade CFR-PEEK rods with 30 vol% carbon fibers (PEEK450-30CA, Junhua High Performance specialty Engineering Plastics (Peek) Products Co. Ltd, Jiangsu, China) and polished on one side to a mirror finish and then ultrasonically purged in acetone, ethanol, and ultra-pure water, respectively, to remove contaminants on the surface. After drying in an oven at 60 °C, the samples were treated by Zr-plasma immersion ion implantation (Zr-PIII). The pressure of the vacuum chamber was 5 × 10−3 Pa. The important processing parameters in Zr-PIII were listed in Table 1. The specimens implanted for 1 h and 2 h were marked as Zr-1 and Zr-2, respectively. And the samples without Zr ion implantation were used as a control which was designated as Zr-0.
Table 1 Processing parameters in Zr plasma immersion ion implantation (PIII)
|
Zr-1 |
Zr-2 |
Cathode source |
99.99% pure zirconium |
99.99% pure zirconium |
Voltage pulse duration (μs) |
500 |
500 |
Cathodic arc pulse duration (μs) |
500 |
500 |
Ion implantation voltage (kV) |
−15 |
−15 |
Pressure (Pa) |
5 × 10−3 |
5 × 10−3 |
Pulsing frequency (Hz) |
10 |
10 |
Ion implantation time (h) |
1 |
2 |
2.2. Surface characterization
Field-emission scanning electron microscopy (FE-SEM, HITACHI, S-4800, Japan) was used to examine the morphologies of samples before and after Zr-PIII at different magnification. Surface chemistry and XPS depth profiles of Zirconium of various specimens were determined by X-ray photoelectron spectroscopy (XPS, Physical Electronic PHI 5802) using monochromatic Al Kα radiation (350 W) as an X-ray source in the City University of Hong Kong.
2.3. Surface mechanical performance test
Nanohardness, elastic modulus, and load-displacement curves were measured by nanoindentation (Nano Indenter G200, Agilent Technologies, USA). The indentation depth of nanohardness and elastic modulus measurements was from 10 nm to 100 nm. The maximal load of Load-displacement curve measurement was 1.6 mN. Eight indents were made to improve the statistics.
2.4. Zirconium ion release
Zr-PIII treated specimens were immersed in 5 ml phosphate buffer saline (PBS, pH = 7.4) for 7, 14, 21, and 28 days at 37 °C. At each period, the solution was collected and the amount of zirconium ions release was analyzed by inductively-coupled plasma atomic emission spectroscopy (ICP-AES, JY 2000-2, France).
2.5.
In vitro biological performance evaluation
2.5.1. Cell culture.
Rat bone mesenchymal stem cells (rBMSCs) (purchased from Cells Resource Center, Shanghai Institutes of Biological Science, Shanghai, China) were used to evaluate in vitro biological performance of various samples. The cells were seeded in a culture dish containing 8 ml of the α-minimum essential medium (a-MEM) (Minimum Essential Medium alpha-Medium, Gbico, Invtrogen, Inc) supplemented with 10% fetal bovine serum (FBS) (Hyclone, USA) and 1% antimicrobial of penicillin and streptomycin (Antibiotic-Antimycotic, Hyclone, USA). The culture dishes were then placed in an incubator containing 5% CO2 at 37 °C. α-MEM was refreshed every 3 days during cell culturing and cells of passages 3–5 were used in the experiments.
2.5.2. Cell adhesion and spreading.
Samples were sterilized by 75% alcohol for 2 h and then transferred into a 24-well plate. 1 ml rBMSC suspension with a density of 5 × 104 cells per ml was added into each well. After 1, 4, 24 hour incubation, samples were transferred into a new 24-well plate and rinsed two times by phosphate buffer saline (PBS) (pH = 7.4) and then samples were kept at 4 °C after adding 0.5 ml 2.5% glutaraldehyde solution into each well to fix cells on samples. After 4 hour fixation, samples were dehydrated by 30 vol%, 50 vol%, 75 vol%, 90 vol%, 95 vol%, 100 vol% alcohol solutions, and mixed solutions of alcohol and the hexamethyl disilazane. Finally, cell morphologies were observed by scanning electron microscopy (SEM, S-3400, HITACHI, Japan) after dehydrated samples being coated with platinum.
2.5.3. Cell proliferation.
rBMSCs were seeded on samples with a density of 1 × 104 cells per ml and incubated at 37 °C in a 5% CO2 incubator for 1, 4, and 7 days. At each time point, samples were transferred into a new 24-well plate and rinsed twice by PBS. Subsequently, 0.5 ml fresh medium with 5% alamarBlue™ was added into each well. After 4 hour incubation at 37 °C in a 5% CO2 incubator, 100 μl medium from each well was added into a 96-well plate and the absorbance was examined at wavelengths of 570 nm and 600 nm by using an enzyme-labeling instrument (BIO-TEK, Synergy 2). The amount of reduced alamarBlue™ was determined by the following formula:
where, A is the absorbance of the test wells, A0 is the absorbance of negative control wells (with media plus alamarBlue™ but no cells), λ1 = 570 nm and λ2 = 600 nm.
2.5.4. Alkaline phosphatase activity assay.
rBMSCs were seeded on various samples with initial densities of 1 × 104 cells per ml (7 days) and 0.5 × 104 cells per ml (14 days). After 7 and 14 day culture, cells on sample surfaces were decomposed and p-nitrophenyl phosphate (pNPP) (Sigma, USA) was added to the resulting lysate, then the mixed solutions were incubated at 37 °C for 30 min. The ALP activity was quantified by measuring the absorbance at a wavelength of 405 nm. With respect to the total amount of protein, it was determined by using the BCA protein assay and normalized with a series of bovine serum albumin (BSA, Sigma) standards by measuring the absorbance at 570 nm. The ALP levels were determined by the ratio of ALP activity and the total amount of protein.
2.5.5. Collagen secretion.
After 7 and 14 day culture of rBMSCs seeded on samples at densities of 1 × 104 cells per ml (7 days) and 0.5 × 104 cells per ml (14 days) in an incubator, collagen in cells was stained by 0.5 ml mixed solutions of 0.1% Sirius Red solution (Sigma) in saturated picric acid at 4 °C for 18 h. Subsequently, samples were rinsed with 0.1 M acetic acid repeatedly. Afterwards, stained cells were observed and images were taken using a fluorescence microscope (Olympus, GX71, Japan). In terms of quantitative analysis, the stained cells on samples were dissolved by an elution (0.2 M NaOH
:
methanol = 1
:
1) and the absorbance at 570 nm of the resulting fluid was measured.
2.5.6. Extracellular matrix mineralization.
The alizarin red staining method was used to determine extracellular matrix (ECM) mineralization of various samples. rBMSCs were seeded on samples with densities of 1 × 104 cells per ml (7 days) and 0.5 × 104 cells per ml (14 days). At each time point, samples were transferred into a new plate and rinsed three times with PBS. Afterwards, cells on samples were fixed by 75 vol% alcohol solutions for 1 hour. Subsequently, 40 mM Alizarin Red was used to stain cell on samples for 10 minutes at room temperature. Finally, the resulting samples were washed by ultra-pure water repeatedly and images of cells were taken using a fluorescence microscope. With respect to quantitative analysis, 0.5 ml 10 mM sodium phosphate solution containing 10% cetylpyridinium chloride was used to dissolve the stain on samples. The absorbance of 100 μl resulting solution at 600 nm was measured to evaluate the extracellular matrix mineralization level.
2.6.
In vitro antibacterial evaluation
Antibacterial performance of samples before and after Zr-PIII was examined by a bacterial counting method using Escherichia coli (E. coli, ATCC 25922) and Staphylococcus aureus (S. aureus, ATCC 25923). Briefly, various samples were rinsed 3 times in 75 vol% alcohol. Afterwards, a 60 μl bacterial suspension with a bacterial density of 107 cfu ml−1 was dropped on the surface of each sample. After 24 h incubation at 37 °C, samples with the bacterial suspension were transferred into a sterilized centrifugal tube in which 4 ml physiological saline was pre-added and then tubes were agitated vigorously to dissociate the bacteria from samples. The resulting suspension was diluted 10, 100, and 1000 times by physiological saline. After that, 100 μl of the various diluted suspension was dropped on agar culture plates (the tryptic soy broth (TSB) or agar plates for S. aureus and Luria-bertani (LB) or agar plates for E. coli) and a sterilized scraper was used to make the suspension homogenously distributed on the plates. Finally, all plates were kept in a 37 °C incubator and the colonies ware counted according to the National Standard of China GB/T4789.2 protocol.
For the SEM observation, a 60 μl bacterial suspension with a bacterial density of 107 cfu ml−1 was seeded on the surface of samples. After 24 h incubation at 37 °C, samples were rinsed 2 times with physiological saline and the bacteria were fixed with 2.5% glutaraldehyde solution for 24 h. The samples were dehydrated by 30 vol%, 50 vol%, 75 vol%, 90 vol%, 95 vol%, 100 vol% alcohol solutions, and mixed solutions of alcohol and the hexamethyl disilazane. Bacterial morphologies were observed using a scanning electron microscope (SEM, S-3400, HITACHI, Japan) after the dehydrated samples being sputter coated with platinum.
2.7. Statistical analysis
All the data were expressed as means ± standard deviations and the statistical analysis was done by using a GraphPad Prism statistical software package. Statistically significant differences (P) between the various groups were measured using two-way analysis of variance.
3. Results
3.1. Surface characterization
Surface morphologies of CFR-PEEK samples before and after Zr-PIII treatment were shown in Fig. 1. The polished CFR-PEEK surface was flat (Fig. 1a) and no significant topographical changes could be observed on CFR-PEEK surfaces after Zr-PIII treatment for 1 h (Fig. 1b). However, for 2 h Zr-PIII treatment, island-like nanostructures were formed (Fig. 1c) with many smaller nanoparticles evenly distributed in the gap of these island-like nanostructures.
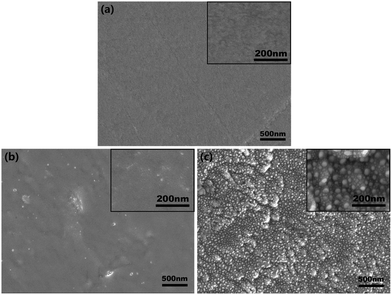 |
| Fig. 1 SEM morphologies of CFR-PEEK samples: (a) Zr-0, (b) Zr-1 and (c) Zr-2. | |
Chemical states of sample surfaces were determined by XPS, which were shown in Fig. 2. In comparison with the XPS survey spectrum of Zr-0 (Fig. 2a), characteristic peaks of Zr 3d5/2 and Zr3p3/2 were detected for both Zr-1 and Zr-2 (Fig. 2b and c). In the high-resolution spectra of Zr 3d, the peaks at 182.10 eV and 184.47 eV corresponded to the typical binding energy Zr 3d5/2 and Zr 3d3/2 states of ZrO2 in Zr-1, respectively (Fig. 2d). Similarly, peaks at positions 181.92 eV and 184.30 eV corresponded to characteristic peaks of Zr 3d5/2 and Zr 3d3/2 states in ZrO2 were also found from the Zr-2 surface. These indicated that ZrO2 had been formed on the Zr-PIII treated samples surface after exposing to an ambient atmosphere. XPS depth profile results (Fig. 2f) indicated that the thickness of the modified layer of Zr-1 and Zr-2 was about 100 nm and 200 nm, respectively. The amount of Zr in the modified layer increased with the implantation time and the amount of zirconium in the outer layer of Zr-2 was twice higher than that of Zr-1. According to the thickness of the modified layer and the implantation time, the coating rate of Zr-PIII was about 100 nm h−1.
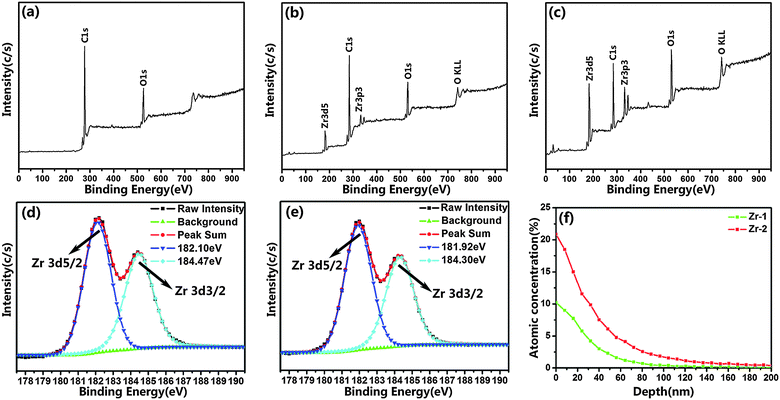 |
| Fig. 2 XPS full spectra of Zr-0 (a), Zr-1 (b) and Zr-2 (c); Zr 3d high resolution spectra of Zr-1 (d) and Zr-2 (e); XPS depth profiles of Zr (f). | |
Nanohardness, elastic modulus, and load-displacement curves were measured by nanoindentation and the results were displayed in Fig. 3. The nanohardness and the elastic modulus of CFR-PEEK increased after Zr-PIII. At the same indentation depth, the nanohardness and the elastic modulus were in the order of Zr-0 < Zr-1 < Zr-2 and Zr-2 not only showed the highest nanohardness with a maximum value of about 4.5 GPa, which was more than twice than that of Zr-0, but also exhibited a maximal elastic modulus of about 14 GPa which was much close to that of human cortical bone (18 GPa). With an increase in the indentation depth, the nanohardness and the elastic modulus of Zr-0, Zr-1 and Zr-2 decreased and became comparable at an indentation depth of 100 nm. With respect to the load-displacement curves, the continuous load-displacement curves (Fig. 3c) illustrated that no abrupt cracking occurred in the modified layers in the loading and unloading stages, meaning the firm attachment of the modified layers on the CFT-PEEK substrate. At the same load, the indentation depths of Zr-0, Zr-1, Zr-2 were in the order of Zr-0 > Zr-1> Zr-2, suggesting that elastic resistance was enhanced after Zr-PIII and Zr-2 had superior elastic resistance.
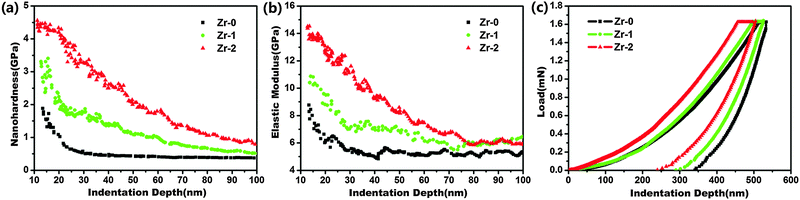 |
| Fig. 3 Nanohardness (a), elastic modulus (b) and load-displacement (c) curves of CFR-PEEK before and after Zr-PIII. | |
3.2. Responses of rBMSCs
Fig. 4a showed the morphologies of rBMSCs cultured on various sample surfaces for 1, 4, and 24 h. After 1 h, cells seeded on Zr-0 samples exhibited a spherical shape with little filose pseudopodium. However rBMSCs seeded on Zr-1 and Zr-2 surfaces adhere and spread very well on the sample surface. After cultured for 4 and 24 h, cells completely covered the various samples surface without obvious difference.
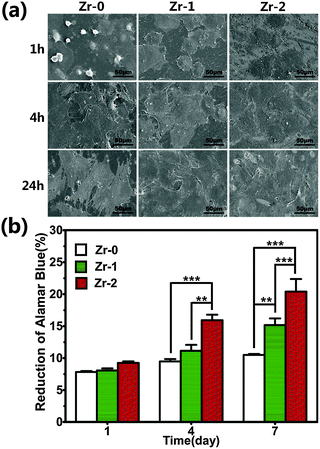 |
| Fig. 4 (a) Adhesion and spreading of rBMSCs cultured on various samples after 1, 4, and 24 h; (b) reduction percentages of alamarBlue™ of rBMSCs cultured on various samples after 1, 4, and 7 days. **P < 0.005, ***P < 0.001. | |
AlamarBlue assay was performed to investigate rBMSC proliferation on the samples after 1, 4, and 7 days (Fig. 4b). rBMSCs cultured on all samples displayed comparable proliferation at day 1. The proliferation of rBMSCs cultured on Zr-1 was higher than that on Zr-0 at day 7. This indicated that ZrO2 formed on sample surfaces exhibited excellent biocompatibility. Additionally, rBMSCs cultured on Zr-2 exhibited significantly higher proliferation at day 4 and day 7 than that on both Zr-0 and Zr-1. This might be ascribed to the nanostructures formed on Zr-2, which were thought to be beneficial to cell growth.
To evaluate the effect on the osteogenic differentiation of rBMSCs, ALP activity was measured after cells cultured on these samples for 7 and 14 days. As shown in Fig. 5, ALP activity of rBMSCs cultured on Zr-2 for 7 days was higher than that on the control (Zr-0) and rBMSCs cultured on Zr-0 and Zr-1 displayed comparable ALP activity at day 7. After culturing for 14 days, the ALP activity level of rBMSCs seeded on both Zr-1 and Zr-2 was significantly higher than that on Zr-0, indicating that Zr-PIII can improve initial osteogenic differentiation of rBMSCs seeded on CFR-PEEK.
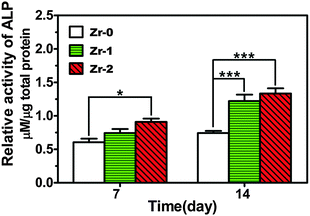 |
| Fig. 5 ALP activity of rBMSCs cultured on various samples for 7 and 14 days. *P < 0.05, ***P < 0.001. | |
Collagen secretion of rBMSCs cultured on various samples and the corresponding quantitative analysis were displayed in Fig. 6. Collagen secretion of rBMSCs cultured for 7 and 14 days on Zr-PIII treated samples was significantly enhanced compared to that on the control. And rBMSCs seeded on Zr-1 and Zr-2 exhibited a comparable collagen secretion level at day 7 and day 14.
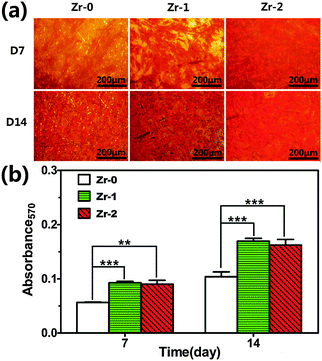 |
| Fig. 6 (a) Collagen secretion of rBMSCs cultured on various samples for 7 and 14 days; (b) quantitative results of Collagen secretion staining. **P < 0.01, ***P < 0.001. | |
The staining images of extracellular matrix (ECM) mineralization of rBMSCs cultured on various samples for 7 and 14 days and corresponding quantitative results were depicted in Fig. 7. The results showed that ECM mineralization levels of rBMSCs cultured on both Zr-1 and Zr-2 were greatly up-regulated compared to that on Zr-0 at both day 7 and day 14. rBMSCs seeded on Zr-1 and Zr-2 exhibited comparable ECM mineralization levels throughout the observation period. Moreover, ECM mineralization of rBMSCs seeded on CFR-PEEK was nearly unchanged at day 7 and day 14, but ECM mineralization of rBMSCs seeded on Zr-1 and Zr-2 at day 14 was much higher than that at day 7.
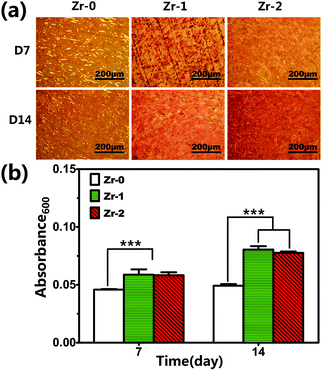 |
| Fig. 7 (a) Matrix mineralization of rBMSCs cultured on various samples for 7 and 14 days; (b) quantitative results of matrix mineralization staining. ***P < 0.001. | |
3.3. Antibacterial activity
S. aureus and E. coli were used to examine antibacterial activity of the samples and these results were shown in Fig. 8. The amounts of E. coli adhered on various sample surfaces were nearly the same, meaning that Zr-PIII treated CFR-PEEK has no antibacterial activity against E. coli. However, Zr-2 exhibited obvious antibacterial activity against S. aureus and the quantity of S. aureus on Zr-2 was greatly reduced, the corresponding antibacterial rate was about 62.7%. It was believed that nano-ZrO2 particles formed on Zr-2 were the main reason for the bacteriostasis of Zr-2 against S. aureus.
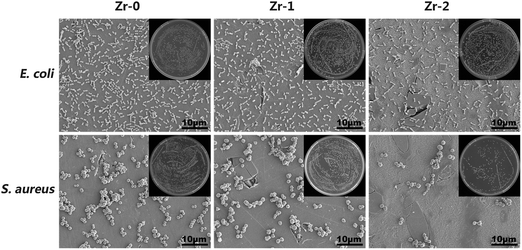 |
| Fig. 8 SEM morphologies of bacteria on various samples. The insets show re-cultivated bacterial colonies on agar: S. aureus and E. coli colonies are previously dissociated from Zr-0, Zr-1, and Zr-2. The concentration of S. aureus and E. coli bacteria seeded on the samples is 107 cfu ml−1. | |
4. Discussion
In general, PIII is recognized as an effective and controllable technology for the surface modification of biomaterials. By regulating pulse voltage, frequency, and implantation time, surface properties such as topography and chemical compositions of biomaterials could be modulated. Thus, Zr-PIII was employed to improve the bioactivity of CFR-PEEK in this work. After Zr-PIII treatment for 2 h, island-like nanostructures with nanoparticle distribution were formed, but in a shorter time treatment like 1 h, no topographical difference was observed on the CFR-PEEK surface compared with the surface of primitive CFR-PEEK (Fig. 1a–c). In our previous study, a growth model of nanopores on the CFR-PEEK surface during Ti-PIII at a high implantation voltage (30 kV) was proposed, in which the effects of thermal ablation and dielectric breakdown were viewed as the driving force of the nanostructure growth.19 To certify our hypothesis, Zr-PIII was also conducted and the similar nanopores were formed on the CFR-PEEK surface during Zr-PIII at a high implantation voltage (30 kV) (data were not published). But in this work, a lower implantation voltage (15 kV) was adopted and island-like nanostructures with nanoparticle distribution rather than nanopore were formed on the surface of CFR-PEEK after Zr-PIII. This indicated that dielectric breakdown did not occur during Zr-PIII (15 kV). It was likely that the strength of electronic field inside the plate capacitor did not rise to the level that dielectric breakdown would occur on the CFR-PEEK surface during Zr-PIII at a lower implantation voltage (15 kV). So thermal ablation was believed to be the driving force of the formation of the nanostructures on the CFR-PEEK surface in this work. During Zr-PIII (15 kV), zirconium ions with high energy could be generated and they accelerated toward the CFR-PEEK substrate by loading a negative bias. When ions penetrated into the polymeric substrates, their kinetic energy could be converted into thermal energy via atomic scale heating (ASH) and the accumulation of heat also occurred.19 In the early stage, it was reported that a dense film would like to be formed on the substrate at low temperature.20 Therefore, no topographical change was observed on the surface of Zr-1 compared with primitive CFR-PEEK. By extending the implantation time, the heat energy was increased to a threshold value and thermal ablation of the polymeric substrate occurred, thus leading to the formation of nanostructures on the surface of CFR-PEEK. As Zr-PIII proceeds, nanoparticles gradually formed due to heat accumulation. These may explain why island-like nanostructures with nanoparticle distribution were formed on the surface of Zr-2.
Zr-PIII could also alter the surface chemical composition of CFR-PEEK. XPS results indicated that the coating rate of Zr-PIII was about 100 nm h−1 and zirconia was formed on the treated sample surface after Zr-PIII. XPS depth profiles indicated that the thickness of the modified layer depended on the implantation time. The thickness of the modified layer of Zr-1 and Zr-2 was about 100 nm and 200 nm, respectively. The amount of Zr increased with the implantation time and the amount of zirconium in the outer layer of Zr-2 was twice higher than that of Zr-1 (Fig. 2). Ion release tests showed that Zr ion release was not detected by ICP-AES after immersion in PBS for 28 days and it might be due to the excellent corrosion resistance of ZrO2. Moreover, nanoindentation tests indicated that the nanohardness, elastic modulus, and elastic resistance were improved after Zr-PIII (Fig. 3a–c). In the recovery of the surgery, it is of great importance for the match of implant stiffness and native bone. In fact, the mismatch of the elastic modulus between implants and native bone could lead to osseous absorption and atrophy, known as stress shielding. In this study, isoelastic CFR-PEEK with an elastic stiffness comparable to bone was produced by Zr-PIII. The maximal elastic modulus was about 14 GPa (Fig. 3b), which was much close to that of human cortical bone (18 GPa2). Unlike conventional coating technologies, high energy zirconium ions in PIII can penetrate into the matrix of CFR-PEEK without creating a striking boundary between the substrate and modified layers, meaning a minimized detachment of the modified layers. The continuous load-displacement curves (Fig. 3c) illustrated that firm attachment of the modified layers on the CFT-PEEK substrate with no abrupt cracking occurred in the modified layers upon increasing the load.
Numerous research studies have reported that nanostructured ZrO2 and ZrO2 films or coatings had excellent biocompatibility and bioactivity as well as elevation of cell responses.32,33,39 It was believed that abundant hydroxyl groups could be formed on the surface of ZrO2 in water solutions through the hydration and hydroxylation of the surface oxide ions.40–43 These hydroxyl groups formed could improve the wettability of the material surface and enhance the adhesion and spreading of cells on the surface of the materials.44,45 Thus, enhanced cell adhesion and spreading of rBMSCs were observed on the CFR-PEEK surface after Zr-PIII (Fig. 4a). Furthermore, the subsequent proliferation of rBMSCs was significantly up-regulated, which was in accord with the results of our previous studies.25,30 Interestingly, rBMSCs seeded on Zr-2 exhibited much better proliferation activity compared to that on Zr-1. It was likely that nanostructures formed on the Zr-2 surface were favorable for cell proliferation, which illustrated that micro- and nanostructures could enhance bioactivity of biomaterials and their corresponding cellular responses.46–48
It is known that osteogenic differentiation of cells around the interface between bone and implants plays a crucial role in the bone-regeneration process. But limited direct bone contact to PEEK was observed through histological analysis, the majority of the interaction of the tissue with the PEEK were soft/connective tissue encapsulation.49 In this study, enhanced ALP activity, collagen secretion, and ECM mineralization of rBMSCs cultured on CFR-PEEK after Zr-PIII were observed. ALP activity and collagen secretion are two key markers of osteogenic differentiation of rBMSCs in the early stage. Higher levels of ALP activity and collagen secretion illustrated more rapid and earlier differentiation of rBMSCs cultured on Zr-PIII modified CFR-PEEK. These results were in accord with other studies reported that ZrO2 films could enhance ALP activity and collagen expression of MG-63 cells.32,33 ECM mineralization is a later marker of osteogenic differentiation of rBMSCs. The results showed that, the ECM mineralization of rBMSCs seeded on Zr-PIII modified CFR-PEEK was significantly enhanced compared to that of rBMSCs seeded on primitive CFR-PEEK. These results indicated that Zr-incorporation could enhance osteogenic differentiation of rBMSCs seeded on CFR-PEEK both in the early and late stage.
Antimicrobial properties are of great importance to avoid implant-related infections. In this study, antibacterial activity was evaluated by a bacterial counting method using both E. coli and S. aureus. The results displayed that Zr-PIII could not improve antibacterial activity of CFR-PEEK against E. coli. But Zr-2 exhibited obvious antibacterial activity against S. aureus, while Zr-1 showed weak bacterial inhibitory activity (Fig. 8). Although the exact antibacterial mechanism is still unknown, some mechanisms, such as the formation of reactive oxygen species (ROS) like superoxide anions O2−
50,51 and the alkaline effect,51 have been proposed to explain the antibacterial activity of metallic oxide nanoparticles. In this work, ZrO2 nanoparticles were formed on the Zr-2 surface, which may lead to the formation of ROS and inhibited the adhesion of S. aureus. It was documented that active sites on the surface of nanoparticles could reduce dissolved oxygen in the solution through single-electron reduction and resulted in the generation of O2− which was stable in a basic environment.52 On the other hand, according to the alkaline effect, the formation of hydroxyl groups around the surface of ZrO2 might result in a higher local pH value on the sample surface, which could inhibit the adhesion of bacteria. It was believed that these two effects lead to the distinctive antibacterial activity of Zr-2 against S. aureus. Compared with S. aureus, the amount of E. coli adhered on Zr-PIII treated sample surfaces was not changed compared with that of E. coli adhered on CFR-PEEK. This might result from the stronger resistance of Gram-negative bacteria to ZrO2 nanoparticles because of the more complex cell membrane both structurally and chemically,51,53,54 of which the packed lipopolysaccharide (LPS) molecules in the outer membrane provided an effective barrier against the antibacterial agent.
5. Conclusions
Bioactive ZrO2 was successfully incorporated into the CFR-PEEK surface by plasma immersion ion implantation technology. The results indicated that nanostructures could be formed on the surface of CFR-PEEK. The mechanical text showed that Zr-PIII treatment could significantly improve the nanohardness, elastic resistance, and elastic modulus. In vitro studies suggested that initial adhesion and spreading, proliferation, ALP activity, collagen secretion and ECM mineralization of rBMSCs were also greatly enhanced after Zr-PIII treatment. Antibacterial examination illustrated that Zr-PIII treated CFR-PEEK with nanostructures exhibited obvious antibacterial activity against S. aureus. This evidence indicated that Zr-PIII treated CFR-PEEK has great potential to be used as an orthopedic implant in hard tissue engineering.
Acknowledgements
Financial support from the National Basic Research Program of China (973 Program, 2012CB933600), National Science Fund for Distinguished Young Scholars (51525207), Shanghai Committee of Science and Technology, China (14JC1493100 and 14XD1403900), National Natural Science Foundation of China (81271704) is acknowledged.
References
- J. M. Toth, M. Wang, B. T. Estes, J. L. Scifert, H. B. Seim and A. S. Turner, Biomaterials, 2006, 27, 324–334 CrossRef CAS PubMed.
- S. M. Kurtz and J. N. Devine, Biomaterials, 2007, 28, 4845–4869 CrossRef CAS PubMed.
-
S. Green, in PEEK Biomaterials Handbook, ed. S. M. Kurtz, William Andrew Publishing, Oxford, 2012, pp. 23–48 Search PubMed.
-
J. M. Toth, in PEEK Biomaterials Handbook, ed. S. M. Kurtz, William Andrew Publishing, Oxford, 2012, pp. 81–92 Search PubMed.
- T. Zhai, L. Z. Di and D. A. Yang, ACS Appl. Mater. Interfaces, 2013, 5, 12499–12509 CAS.
- Y. Zhao, H. M. Wong, W. H. Wang, P. H. Li, Z. S. Xu, E. Y. W. Chong, C. H. Yan, K. W. K. Yeung and P. K. Chu, Biomaterials, 2013, 34, 9264–9277 CrossRef CAS PubMed.
- H. K. Tsou, P. Y. Hsieh, C. J. Chung, C. H. Tang, T. W. Shyr and J. L. He, Surf. Coat. Technol., 2009, 204, 1121–1125 CrossRef CAS.
- R. Ma and T. T. Tang, Int. J. Mol. Sci., 2014, 15, 5426–5445 CrossRef PubMed.
- H. Wang, M. Xu, W. Zhang, D. T. K. Kwok, J. Jiang, Z. Wu and P. K. Chu, Biomaterials, 2010, 31, 8181–8187 CrossRef CAS PubMed.
- C. von Wilmowsky, E. Vairaktaris, D. Pohle, T. Rechtenwald, R. Lutz, H. Munstedt, G. Koller, M. Schmidt, F. W. Neukam, K. A. Schlegel and E. Nkenke, J. Biomed. Mater. Res., Part A, 2008, 87A, 896–902 CrossRef CAS PubMed.
- B. D. Hahn, D. S. Park, J. J. Choi, J. Ryu, W. H. Yoon, J. H. Choi, J. W. Kim, C. W. Ahn, H. E. Kim, B. H. Yoon and I. K. Jung, Appl. Surf. Sci., 2013, 283, 6–11 CrossRef CAS.
- J. H. Lee, H. L. Jang, K. M. Lee, H. R. Baek, K. Jin, K. S. Hong, J. H. Noh and H. K. Lee, Acta Biomater., 2013, 9, 6177–6187 CrossRef CAS PubMed.
- P. K. Chu, J. Y. Chen, L. P. Wang and N. Huang, Mater. Sci. Eng., R, 2002, 36, 143–206 CrossRef.
- K. Fricke, S. Reuter, D. Schroder, V. Schulz-von der Gathen, K. D. Weltmann and T. von Woedtke, IEEE Trans. Plasma Sci., 2012, 40, 2900–2911 CrossRef CAS.
- K. Narushima, S. Tasaka and N. Inagaki, Jpn. J. Appl. Phys., Part 1, 2002, 41, 6506–6516 CrossRef CAS.
- C. Jama, O. Dessaux, P. Goudmand, L. Gengembre and J. Grimblot, Surf. Interface Anal., 1992, 18, 751–756 CrossRef CAS.
- H. Y. Wang, T. Lu, F. H. Meng, H. Q. Zhu and X. Y. Liu, Colloids Surf., B, 2014, 117, 89–97 CrossRef CAS PubMed.
- J. H. Lee, H. W. Jung, I. K. Kang and H. B. Lee, Biomaterials, 1994, 15, 705–711 CrossRef CAS PubMed.
- T. Lu, X. Y. Liu, S. Qian, H. L. Cao, Y. Q. Qiao, Y. F. Mei, P. K. Chu and C. X. Ding, Biomaterials, 2014, 35, 5731–5740 CrossRef CAS PubMed.
- T. Lu, J. Wen, S. Qian, H. L. Cao, C. Q. Ning, X. X. Pan, X. Q. Jiang, X. Y. Liu and P. K. Chu, Biomaterials, 2015, 51, 173–183 CrossRef CAS PubMed.
- A. Afzal, Mater. Express, 2014, 4, 1–12 CrossRef CAS.
- Y. Y. Yan and Y. Han, Surf. Coat. Technol., 2007, 201, 5692–5695 CrossRef CAS.
- D. Sarkar, S. K. Swain, S. Adhikari, B. S. Reddy and H. S. Maiti, Mater. Sci. Eng., C, 2013, 33, 3413–3417 CrossRef CAS PubMed.
- X. Liu, A. Huang, C. Ding and P. K. Chu, Biomaterials, 2006, 27, 3904–3911 CrossRef CAS PubMed.
- G. Wang, X. Liu, H. Zreiqat and C. Ding, Colloids Surf., B, 2011, 86, 267–274 CrossRef CAS PubMed.
- V. Covacci, N. Bruzzese, G. Maccauro, C. Andreassi, G. A. Ricci, C. Piconi, E. Marmo, W. Burger and A. Cittadini, Biomaterials, 1999, 20, 371–376 CrossRef CAS PubMed.
- F. Carinci, F. Pezzetti, S. Volinia, F. Francioso, D. Arcelli, E. Farina and A. Piattelli, Biomaterials, 2004, 25, 215–228 CrossRef CAS PubMed.
- Y. Josset, Z. Oum'Hamed, A. Zarrinpour, M. Lorenzato, J. J. Adnet and D. Laurent-Maquin, J. Biomed. Mater. Res., 1999, 47, 481–493 CrossRef CAS PubMed.
- L. Saldana, A. Mendez-Vilas, L. Jiang, M. Multigner, J. L. Gonzalez-Carrasco, M. T. Perez-Prado, M. L. Gonzalez-Martin, L. Munuera and N. Vilaboa, Biomaterials, 2007, 28, 4343–4354 CrossRef CAS PubMed.
- G. C. Wang, F. H. Meng, C. X. Ding, P. K. Chu and X. Y. Liu, Acta Biomater., 2010, 6, 990–1000 CrossRef CAS PubMed.
- C. J. Frandsen, K. S. Brammer, K. Noh, L. S. Connelly, S. Oh, L. H. Chen and S. J. Jin, Mater. Sci. Eng., C, 2011, 31, 1716–1722 CrossRef CAS.
- S. L. Zhang, J. Y. Sun, Y. Xu, S. Qian, B. Wang, F. Liu and X. Y. Liu, Bio-Med. Mater. Eng., 2013, 23, 373–385 CAS.
- S. L. Zhang, J. Y. Sun, Y. Xu, S. Qian, B. Wang, F. Liu and X. Y. Liu, Biointerphases, 2012, 7, 10 CrossRef PubMed.
- Y. Akagawa, Y. Ichikawa, H. Nikai and H. Tsuru, J. Prosthet. Dent., 1993, 69, 599–604 CrossRef CAS PubMed.
- S. Schultze-Mosgau, H. Schliephake, M. Radespiel-Troger and F. W. Neukam, Oral Surg. Oral Med. Oral Pathol. Oral Radiol. Endod., 2000, 89, 91–98 CrossRef CAS.
- M. Gahlert, S. Rohling, M. Wieland, C. M. Sprecher, H. Kniha and S. Milz, Clin. Oral Implants Res., 2009, 20, 1247–1253 CrossRef CAS PubMed.
- S. L. Jangra, K. Stalin, N. Dilbaghi, S. Kumar, J. Tawale, S. P. Singh and R. Pasricha, J. Nanosci. Nanotechnol., 2012, 12, 7105–7112 CrossRef CAS PubMed.
- M. Gouda, J. Ind. Text., 2012, 41, 222–240 CrossRef CAS.
- Y. Zhao, M. I. Jamesh, W. K. Li, G. S. Wu, C. X. Wang, Y. F. Zheng, K. W. K. Yeung and P. K. Chu, Acta Biomater., 2014, 10, 544–556 CrossRef CAS PubMed.
- H. Tamura, K. Mita, A. Tanaka and M. Ito, J. Colloid Interface Sci., 2001, 243, 202–207 CrossRef CAS.
- K. T. Jung and A. T. Bell, J. Mol. Catal. A: Chem., 2000, 163, 27–42 CrossRef CAS.
- A. Ignatchenko, D. G. Nealon, R. Dushane and K. Humphries, J. Mol. Catal. A: Chem., 2006, 256, 57–74 CrossRef CAS.
- T. Yamaguchi, Y. Nakano and K. Tanabe, Bull. Chem. Soc. Jpn., 1978, 51, 2482–2487 CrossRef CAS.
- J. H. Lee, J. W. Park and H. B. Lee, Biomaterials, 1991, 12, 443–448 CrossRef CAS PubMed.
- H. Seyednejad, T. Vermonden, N. E. Fedorovich, R. van Eijk, M. J. van Steenbergen, W. J. A. Dhert, C. F. van Nostrum and W. E. Hennink, Biomacromolecules, 2009, 10, 3048–3054 CrossRef CAS PubMed.
- K. C. Popat, R. H. Daniels, R. S. Dubrow, V. Hardev and T. A. Desai, J. Orthop. Res., 2006, 24, 619–627 CrossRef CAS PubMed.
- X. J. Wang, Y. C. Li, J. G. Lin, Y. Yamada, P. D. Hodgson and C. E. Wen, Acta Biomater., 2008, 4, 1530–1535 CrossRef CAS PubMed.
- G. B. Wei and P. X. Ma, Adv. Funct. Mater., 2008, 18, 3568–3582 CrossRef CAS PubMed.
-
A. H. C. Poulsson and R. G. Richards, in PEEK Biomaterials Handbook, ed. S. M. Kurtz, William Andrew Publishing, Oxford, 2012, pp. 145–161 Search PubMed.
- H. Lei, D. Q. Li, Y. J. Lin, D. G. Evans and D. Xue, Chin. Sci. Bull., 2005, 50, 514–519 CrossRef.
- Z. X. Tang and B. F. Lv, Braz. J. Chem. Eng., 2014, 31, 591–601 CrossRef.
- L. Huang, D. Q. Li, Y. J. Lin, M. Wei, D. G. Evans and X. Duan, J. Inorg. Biochem., 2005, 99, 986–993 CrossRef CAS PubMed.
- M. J. Hajipour, K. M. Fromm, A. A. Ashkarran, D. J. de Aberasturi, I. R. de Larramendi, T. Rojo, V. Serpooshan, W. J. Parak and M. Mahmoudi, Trends Biotechnol., 2012, 30, 499–511 CrossRef CAS PubMed.
- K. Y. Yoon, J. H. Byeon, J. H. Park and J. Hwang, Sci. Total Environ., 2007, 373, 572–575 CrossRef CAS PubMed.
|
This journal is © The Royal Society of Chemistry 2016 |
Click here to see how this site uses Cookies. View our privacy policy here.