DOI:
10.1039/C5TX00292C
(Paper)
Toxicol. Res., 2016,
5, 97-106
Evaluation of zinc-doped magnetite nanoparticle toxicity in the liver and kidney of mice after sub-chronic intragastric administration
Received
18th August 2015
, Accepted 2nd November 2015
First published on 18th November 2015
Abstract
Super-paramagnetic iron oxide nanoparticles (SPIONs) have been approved for clinical use due to their salient super-paramagnetic properties and low toxicity. Zn2+ doped SPIONs possess significantly higher magnetic susceptibility than that of conventional SPIONs. Here we evaluated the potential toxicity of Zn2+ doped Fe3O4 nanoparticles (Zn0.4Fe2.6O4 NPs) in the liver and kidney of mice after repeated intragastric administration for 30 days. Zn0.4Fe2.6O4 NPs did not cause significant changes in their body weights and the coefficients of the liver and kidney, but increased the levels of Fe and Zn in the two organs. Zn0.4Fe2.6O4 NP induced slight oxidative stress in the liver and kidney, which could be successfully counteracted by their intrinsic antioxidant systems and had no observable hazardous effects on the histopathology, ultrastructure and functions of the two organs. These results demonstrated that high-performance magnetic Zn0.4Fe2.6O4 NPs did not produce apparent toxicity in the liver and kidney of mice even after sub-chronic intragastric administration. In addition, Zn2+ doping not only markedly enhanced magnetic susceptibility of Zn0.4Fe2.6O4 NPs but also significantly increased the stability of Zn0.4Fe2.6O4 NPs in biological conditions, making them appropriate for use in magnetic resonance imaging and drug delivery by the oral route.
1. Introduction
Super-paramagnetic iron oxide nanoparticles (SPIONs) offer many applications in biomedicine such as bioimaging, targeted drug delivery, biosensors, anticancer hyperthermia therapy, tissue repair, and cell sorting mainly owing to their good chemical stability and magnetic responsiveness.1–4 SPIONs are the only magnetic nanomaterials approved for clinical use by the US Food and Drug Administration and European Medicines Agency, which take advantage of their salient super-paramagnetic properties.5,6 Anticancer drugs can be transported through the vascular system and specifically targeted to the tumor site using SPIONs as drug delivery systems with the aid of a magnetic field, reducing the damage to healthy cells.
Metal ion doped iron oxide nanoparticles, such as CoFe2O4, NiFe2O4, MnFe2O4, exhibit strong magnetic properties and have enhanced magnetic resonance imaging (MRI) contrast effects that are significantly superior to that of conventional SPIONs.7 Nevertheless, using these metal ion doped iron oxide nanoparticles in biomedical research will be hindered seriously as a result of the high-toxicity levels associated with the presence of these transition metals (Co, Ni, Mn).8–10 Jang et al.11 reported that Zn2+ doped iron oxide nanoparticles exhibit a high magnetization value, which significantly enhances their MRI contrast and hyperthermic effects. Their preliminary in vitro study showed that the Zn2+ doped SPIONs are nontoxic to healthy cells.11 Sufficient magnetic susceptibility is essential for effective utilization of magnetic force to ensure the transport of the drug carrier to the target site before release. Zn2+ doped SPIONs possess higher magnetic susceptibility than that of conventional SPIONs. Therefore, Zn2+ doped SPIONs as drug carriers and contrast imaging agents are significantly superior to that of conventional SPIONs. Recently, Zn2+ doped Fe3O4 nanoparticles (Zn0.4Fe2.6O4 NPs) have been used as a magnetic switch to control apoptosis signaling pathways in vivo by using a magnetic field.12
Applications of Zn2+ doped SPIONs in the field of diagnostics and therapy require a detailed understanding of their in vivo toxicity to ensure their safety. SPIONs are usually used for the delivery of diagnostic and therapeutic agents by the intravenous route. SPIONs are also suitable candidates for the oral delivery of therapeutic agents13,14 and MRI contrast agents for the diseases of the gastrointestinal tract organs.15 However, there are not many studies with respect to the toxicity of SPIONs following oral administration. Compared to the parenteral route of administration, oral administration can improve patient compliance and comfort. Anticancer drug delivery will inevitably involve sub-long-term or even long-term administration of nanoparticles especially by the oral route. Therefore, it is critical to assess the toxicity of Zn2+ doped SPIONs by oral administration. The liver and kidney are common target organs regardless of exposure routes and animal types.16 The present study was designed to determine Zn0.4Fe2.6O4 NP toxicity in the liver and kidney of mice by one month of repeated intragastric administration.
2. Materials and methods
2.1 Chemicals and characterization
FeSO4·(NH4)2SO4·6H2O, FeCl3·6H2O, NaOH, oleic acid, ethanol and pepsin were supplied by Sinopharm Chemical Reagent Co., Ltd (Shanghai, China). 2,3-Dimercaptosuccinic acid (DMSA) was purchased from Tokyo Chemical Industry Co., Ltd (Tokyo, Japan). The phase purity of the sample was examined by X-ray diffraction (XRD) using CuKa radiation (k = 1.5418 Å, 40 kV, 200 mA) (TTR-III, Rigaku Ltd, Japan). Transmission electron microscopy (TEM) analyses were carried out using a transmission electron microscope (JEM-2010, JEOL Ltd, Japan). Magnetic properties were determined using a SQUID magnetometer (SQUID-VSM, Quantum Design, USA). Elemental analysis was performed on an energy dispersive X-ray spectroscope (EDS) (JEM-2010, JEOL Ltd, Japan) and an inductively coupled plasma-atomic emission spectrometer (ICP-AES) (OPTIMA 7300DV, PerkinElmer, USA). The hydrodynamic diameters of Zn0.4Fe2.6O4 NPs in artificial intestinal fluid (50 mM KH2PO4, pH 6.8) and artificial blood solution (137 mM NaCl, 2.7 mM KCl, 8 mM Na2HPO4, 1.5 mM KH2PO4, pH 7.4) were determined by a dynamic light scattering (DLS) instrument (DynaPro-MS800, ATC, USA).
2.2 Synthesis of Zn0.4Fe2.6O4 NPs
A simple and low-cost method based on an oleic acid/alcohol/water system was modified to synthesize Zn0.4Fe2.6O4 nanoparticles.7 FeSO4·(NH4)2SO4·6H2O and ZnSO4 were dissolved in 20 mL of distilled water to obtain a 1.73 × 10−3 mol Fe2+ and 2.67 × 10−4 mol Zn2+ precursor. Then, 10 mL of oleic acid, 1 g of NaOH and 10 mL of ethanol were mixed by stirring at room temperature to get a uniform solution. Thereafter, the Fe2+ precursor was added to the mixed solution. After stirring for a few minutes, the precipitate turned brown. Then, the mixed reactants were transferred into a 50 mL autoclave, sealed, and heated at 230 °C for 15 h. The system was then allowed to cool to room temperature. The products were deposited at the bottom of the vessel. Cyclohexane was added to the solution to collect the nanoparticles. Upon addition of ethanol, the powder precipitated and was collected by centrifugation and washed with ethanol several times. Finally, the organic surfactants on the nanoparticle surface were double-exchanged with DMSA to make the nanoparticles completely dispersed in distilled water. For Fe3O4 NP synthesis, the same procedure was utilized in which the amount of reagent used was FeSO4·(NH4)2SO4·6H2O (0.002 mol Fe2+) instead of the mixture of FeSO4·(NH4)2SO4·6H2O and ZnSO4.
2.3 Treatment of animals
Mice (30 ± 2 g, 6–8 weeks old) were purchased from Shanghai SLAC Laboratory Animal Co., Ltd (Shanghai, China). Animals were housed under controlled conventional conditions (12 h light/dark cycle; temperature 22 ± 2 °C; relative humidity 50 ± 5%) with distilled water and sterilized food available ad libitum. All experiments were conducted in accordance with the guidelines of University of Science and Technology of China for the care and use of laboratory animals. All experimental protocols were approved by the Animal Ethical Committee of University of Science and Technology of China (USTCACUC1401019). After one week of acclimation, mice were randomly divided into two groups (10 male mice in each group): control group (0.9% saline) and experimental group (50 mg per kg BW of Zn0.4Fe2.6O4 NPs per day). Zn0.4Fe2.6O4 NPs were suspended in physiological saline and stirred for 5 min and then sonicated for 30 min at 90 W before the administration in mice. Chemicals were given to mice via intragastric administration at a dose of 0.1 ml per 10 g BW once a day for 30 consecutive days. Body weights of all mice were recorded every day and any clinical signs were carefully observed during the study period. After 30 days, the mice were anaesthetized by ether and sacrificed, and the blood samples were collected and the organs of liver and kidney were dissected out for further analysis. The coefficients of organs to body weight were calculated as the ratio of organs (wet weight, mg) to body weight (g).
2.4 Assays for serum biochemical parameters
Serum was harvested by centrifuging blood at 2500 rpm for 10 min. Liver function was evaluated with serum levels of alkaline phosphatase (ALP), alanine aminotransferase (ALT), total bilirubin levels (TBIL), lactate dehydrogenase (LDH) and aspartate aminotransferase (AST). Nephrotoxicity was determined with creatinine (CRE), blood urea nitrogen (BUN) and uric acid (UA). All biochemical parameter assays were performed with the commercial kits (Roche Diagnostics, Mannheim, Germany) by means of a biochemical autoanalyzer (Roche Modular DPP System, Germany).
2.5 Histopathological examination
Tissues of the liver and kidney were removed from the mice and fixed in 10% (v/v) formalin, embedded in paraffin blocks and sectioned at 5 μm. The sections were stained with hematoxylin and eosin and then examined using an optical microscope (IX-81, Olympus, Japan) at 40× magnification.
2.6 Observation of hepatocyte and nephrocyte ultrastructure
Tissues of the liver and kidney were removed from the mice and immediately fixed in 0.1 M phosphate buffer (pH 7.2) containing 3% glutaraldehyde and 2% paraformaldehyde overnight at 4 °C and then post-fixed for 2 h in 1% osmium tetroxide in the same buffer. The tissues were dehydrated gradually in ethanol (30%–100%) and embedded in Epon. Ultrathin sections (100 nm) were cut, contrasted with lead citrate and uranyl acetate, and then visualized with a Tecnai G2 F20 transmission electron microscope (FEI, Portland, USA).
2.7 Measurement of oxidative stress makers
The liver and kidney tissues were assayed for the oxidative biomarkers by the conventional methods as described previously.17 The levels of glutathione (GSH) and lipid peroxidation product malondialdehyde (MDA) and the activities of superoxide dismutase (SOD) and glutathione peroxidase (GSH-Px) were examined by commercial kits (Nanjing Jiancheng Bioengineering Institute, Nanjing, China), according to the manufacturer's instructions.
2.8 Analysis of contents of Fe and Zn
The blood (0.3 ml) and tissues of the liver and kidney (0.1–0.3 g) were pre-digested with ultrapure nitric acid overnight. The samples were then heated at 130 °C to remove the remaining nitric acid followed by addition of 0.5 mL of 70% perchloric acid. Then, the solutions were heated again until they were evaporated nearly to dryness. Finally, the samples were diluted to 3 mL with 2% (v/v) nitric acid. The Fe and Zn contents in the samples were determined by ICP-AES.
2.9 Assays for stability of nanoparticles
To evaluate the stability of nanoparticles in biological conditions, 5 mg Zn0.4Fe2.6O4 NPs or Fe3O4 NPs were incubated with 5 mL of artificial gastric fluid (0.32% pepsin, 0.2% NaCl, pH 1.5), artificial intestinal fluid (50 mM KH2PO4, 0.42 mM Trypsin, pH 6.8) or artificial blood solution (137 mM NaCl, 2.7 mM KCl, 8 mM Na2HPO4, 1.5 mM KH2PO4, 10 μM BSA, pH 7.4) at 37 °C with gentle shaking. After different incubation times, supernatants were collected by centrifugation at 15
000g for 25 min. The metal concentrations in supernatants were determined by ICP-AES.
2.10 Statistics analysis
Values were expressed as means ± SD. Statistical analyses were carried out by one-way analysis of variance test using SPSS 16.0 followed by Dunnett's test (SPSS Inc., Chicago, IL). A value of p < 0.05 was considered statistically significant for all tests.
3. Results
3.1 Synthesis and characterization of Zn0.4Fe2.6O4 NPs
The size and morphology of as-synthesized Zn0.4Fe2.6O4 NPs were examined by TEM. As illustrated in Fig. 1A and B, as-synthesized spherical Zn0.4Fe2.6O4 NPs were uniform in sizes and well-dispersed with an average particle diameter of 11.0 ± 0.5 nm. DLS measurements showed that the hydrodynamic diameters of Zn0.4Fe2.6O4 NPs in artificial intestinal fluid and artificial blood solution were 22.0 ± 0.6 nm and 20.5 ± 0.5 nm (mean ± SE, n = 3) (Fig. 1C), respectively, which were slightly larger than those determined by TEM imaging, suggesting that Zn0.4Fe2.6O4 NPs did not aggregate in artificial intestinal fluid or in artificial blood solution. X-ray diffraction analysis (Fig. 1D) indicated that the nanoparticles had high-quality crystallinity and the position and relative intensity of all diffraction peaks matched well with the standard powder diffraction data for bulk cubic spinel-structured magnetite (JCPDS file no. 75-0033). The Zn2+ doping level was estimated by EDS and ICP-AES. EDS analysis demonstrated that the atomic ratio of Fe to Zn was 2.58
:
0.42 (Fig. 1E), which was very close to the initial precursor ratio. ICP-AES analysis confirmed that the Fe to Zn mole ratio of the sample was 2.61
:
0.39, which nearly agreed with the EDS result. The magnetic saturation value of Zn0.4Fe2.6O4 NPs measured by a Quantum Design superconducting quantum interference device (SQUID) at 300 K was 75 emu g−1, which was higher than that of Fe3O4 nanoparticles (Fe3O4 NPs) (47 emu g−1) (Fig. 1F).
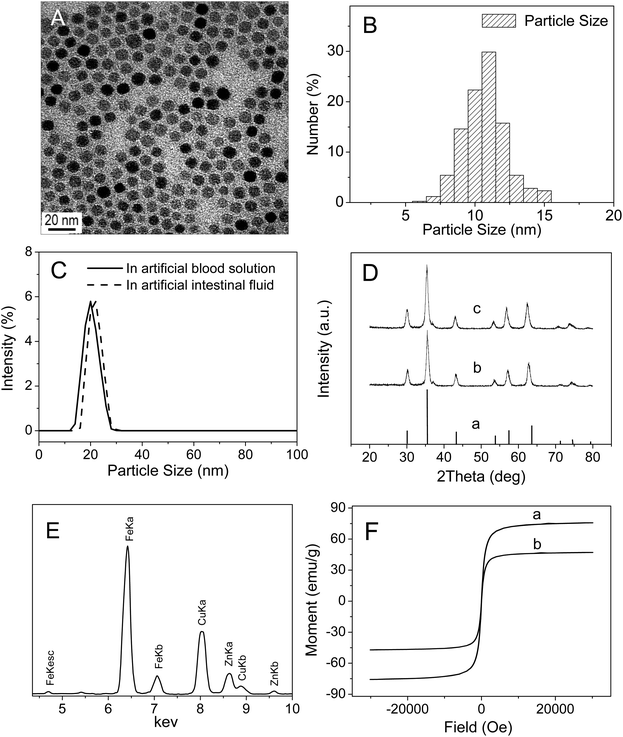 |
| Fig. 1 Characterization of as-prepared 11.0 ± 0.5 nm Zn0.4Fe2.6O4 NPs. (A) TEM image of Zn0.4Fe2.6O4 NPs; (B) particle size distribution histograms of Zn0.4Fe2.6O4 NPs; (C) particle size distributions of Zn0.4Fe2.6O4 NPs in artificial intestinal fluid and artificial blood solution measured by DLS; (D) XRD patterns of standard Fe3O4 (JCPDS 75-0033) (a), as-prepared Fe3O4 NPs (b) and Zn0.4Fe2.6O4 NPs (c); (E) energy dispersive X-ray spectroscopy of Zn0.4Fe2.6O4 NPs; (F) magnetization curves of the Zn0.4Fe2.6O4 NPs (a) and Fe3O4 NPs (b) at 300 K. | |
3.2 Body weight and coefficients of organs
During the 30 days study period, no animal showed any abnormal daily activity and symptoms, and no significant difference was observed in the body weights of mice between the control and Zn0.4Fe2.6O4 NP groups (Fig. 2A). After daily intragastric administration of Zn0.4Fe2.6O4 NPs for 30 days, the coefficients of the liver and kidney had no significant change compared to the control (Fig. 2B).
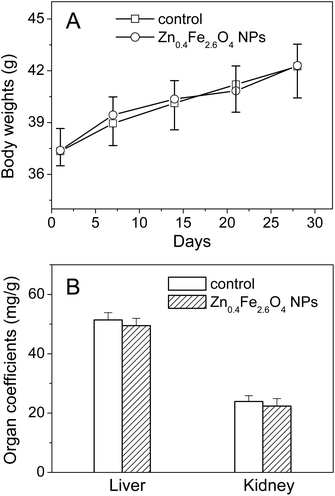 |
| Fig. 2 Body weight and coefficients of the liver and kidneys. Body weight increases of mice during the period of intragastric administration of Zn0.4Fe2.6O4 NPs (A) and coefficients of the liver and kidneys after intragastric administration of Zn0.4Fe2.6O4 NPs for 30 days (B). Physiological saline was used as control. Data are shown as means ± SD (n = 10). | |
3.3 Serum biochemical parameters
As shown in Table 1, all biochemical markers for hepatic function increased in Zn0.4Fe2.6O4 NP-administered mice compared to the control group, except for TBIL and ALP levels. By contrast, biochemical markers for kidney function, CRE, BUN and UA were almost unchanged compared with the control group.
Table 1 Biochemical parameters in the serum of mice
Index |
Control |
Zn0.4Fe2.6O4 NPs |
The mice were intragastrically administrated with Zn0.4Fe2.6O4 NPs for 30 days. Data presented as mean ± SD (n = 10). *p < 0.05 versus the control. Physiological saline was used as control. |
LDH (U L−1) |
590 ± 37 |
802 ± 53* |
TBIL (μmol L−1) |
1.80 ± 0.24 |
1.87 ± 0.31 |
ALT (U L−1) |
34.4 ± 4.0 |
52.0 ± 4.8* |
AST (U L−1) |
110 ± 7 |
165 ± 16* |
AST/ALT |
3.19 ± 0.32 |
3.17 ± 0.26 |
ALP (U L−1) |
61.7 ± 4.9 |
57.9 ± 3.9 |
BUN (mmol L−1) |
9.6 ± 1.9 |
10.0 ± 1.6 |
CRE (μmol L−1) |
66.1 ± 4.9 |
60.6 ± 3.4 |
UA (μmol L−1) |
142 ± 15 |
138 ± 18 |
3.4 Histopathological and ultrastructural analysis of tissues
Fig. 3 shows the histopathological photomicrographs of the liver and kidney tissues. No obvious abnormal pathological changes were found in the liver and kidney tissues of mice after 30 days of oral administration of Zn0.4Fe2.6O4 NPs, compared to the control group. Transmission electron microscopy sections at the cellular level show that both the liver and kidney cells had ultrastructures similar to the control group (Fig. 4).
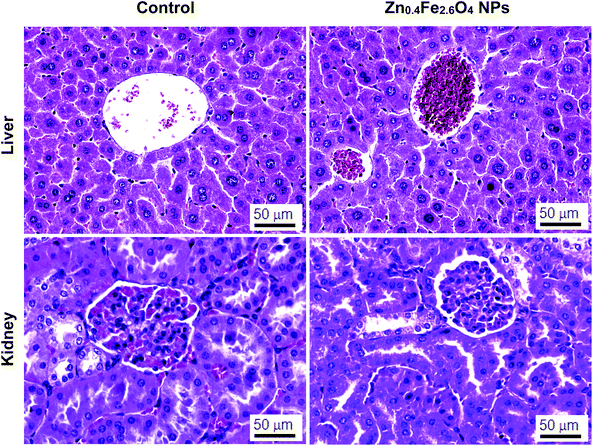 |
| Fig. 3 Histopathological evaluation of the liver and kidney. The mice were intragastrically administrated with Zn0.4Fe2.6O4 NPs for 30 days. Samples were stained with hematoxylin and eosin and observed at 40× magnification. No abnormal histopathological changes were observed for all sections. Physiological saline was used as control. | |
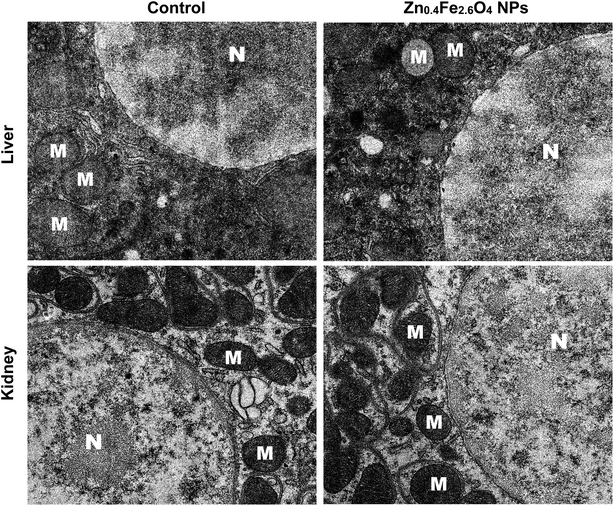 |
| Fig. 4 TEM images of the liver and kidney in mice. The mice were intragastrically administrated with Zn0.4Fe2.6O4 NPs for 30 days. No abnormal ultrastructural changes were observed for all tested cells. Note: N, nucleus; M, mitochondrion. Physiological saline was used as control. | |
3.5 Oxidative stress in liver and kidney
The biological responses were determined by assaying the endogenous antioxidative enzymes, GSH-Px and SOD in the liver and kidney. As shown in Fig. 5, the GSH-Px activity increased in the liver but decreased in the kidney (p < 0.05) in the Zn0.4Fe2.6O4 NP group compared to the control. The SOD activity in the Zn0.4Fe2.6O4 NP group was nearly the same as that of the control group in the liver but increased in the kidney compared to the control. GSH, a major non-enzymatic antioxidant, plays a vital role in cellular defense, which is a crucial determinant of tissue susceptibility to the toxic effects of reactive oxygen species (ROS), so it can be used as one of the earlier biomarkers to assess the effects of exposure to foreign harmful objects.18 MDA is the end product of lipid peroxidation and is also regarded as an index of cellular damage. Therefore, the effects of Zn0.4Fe2.6O4 NPs on the oxidative stress in the liver and kidney were further investigated by assaying their GSH and MDA levels. As shown in Fig. 5C and D, higher GSH and MDA levels were found in both the liver and kidney in the Zn0.4Fe2.6O4 NP group compared with the control group (p < 0.05).
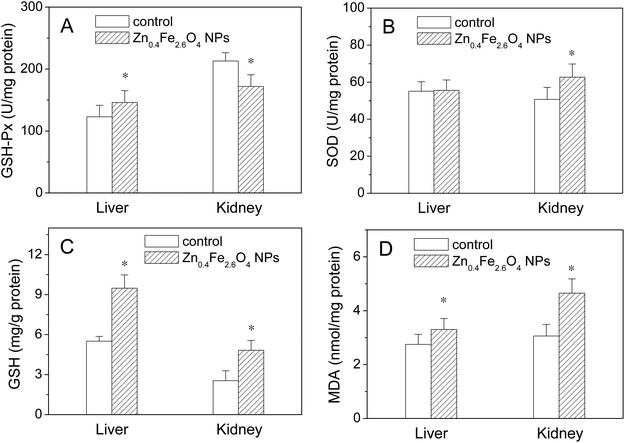 |
| Fig. 5 The activities of GSH-Px and SOD, and the levels of GSH and MDA in the liver and kidney. The mice were intragastrically administrated with Zn0.4Fe2.6O4 NPs for 30 days. Physiological saline was used as control. Results are presented as mean ± SD (n = 6). *p < 0.05 versus the control. | |
3.6 Contents of Fe and Zn
The contents of Fe and Zn in the liver, kidney and whole blood after intragastric administration with Zn0.4Fe2.6O4 NPs for 30 days were determined by ICP-AES. As shown in Fig. 6A and B, significant increases in Fe and Zn contents were found in the liver and kidney in the Zn0.4Fe2.6O4 NP group compared with the control (p < 0.05). As shown in Fig. 6C and D, a slight increase in the Zn content and a significant increase in the Fe content were observed in the blood compared with the control (p < 0.05).
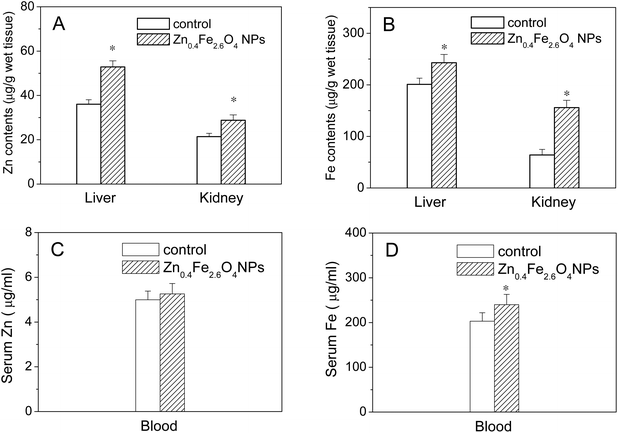 |
| Fig. 6 Systemic absorption of nanoparticles from the gastrointestinal tract. The contents of Zn and Fe in the liver, kidney and whole blood after 30 day-repeated intragastric administration with Zn0.4Fe2.6O4 NPs. Physiological saline was used as control. Data are shown as means ± SD (n = 6). *p < 0.05 versus the control. | |
3.7 Stability of nanoparticles in biological conditions
To analyze whether Zn2+ doping affects the stability of Fe3O4 NPs in biological conditions, dissolution experiments were carried out to measure the release of metal ions from nanoparticles into artificial gastric fluid, intestinal fluid or blood solution. As shown in Fig. 7A, most of the Fe3O4 NPs and Zn0.4Fe2.6O4 NPs remained insoluble in artificial gastric fluid after 4 h. 6.3% of Fe ions in Fe3O4 NPs, 3.8% of Fe ions and 3.9% Zn of ions in Zn0.4Fe2.6O4 NPs were released into the supernatant after 4 h, suggesting that the stability of Fe3O4 NPs was significantly enhanced by Zn2+ doping. As shown in Fig. 7B and C, Zn0.4Fe2.6O4 NPs were more stable in artificial intestinal fluid or artificial blood solution than in artificial gastric fluid. In addition, the stability of Fe3O4 NPs in artificial intestinal fluid or artificial blood solution was also significantly enhanced by Zn2+ doping. These results also revealed that both Fe and Zn ions were released from Zn0.4Fe2.6O4 NPs at a similar rate in all tested solutions.
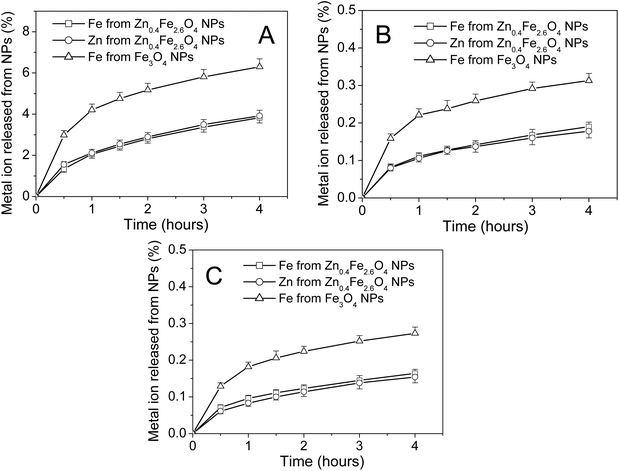 |
| Fig. 7 Metal ions released from Zn0.4Fe2.6O4 NPs in biological conditions. Percentage of dissolution of metal ions was measured using ICP-AES after incubation of Zn0.4Fe2.6O4 NPs or Fe3O4 NPs with the artificial gastric fluid (A), artificial intestinal fluid (B) and artificial blood solution (C) at different times. Data are shown as means ± SD (n = 3). | |
4. Discussion
SPIONs are often classified as biocompatible, showing no severe adverse effects in vivo.19 Jain et al.20 reported that iron oxide magnetic nanoparticles do not induce oxidative damage in the liver of rats after intravenous administration of a single dose of 10 mg Fe per kg body weight (BW). Singh et al.21 demonstrated that the accumulated Fe does not cause significant genotoxicity in rats treated orally with single doses of Fe2O3 nanoparticles (Fe2O3 NPs) (500, 1000, 2000 mg per kg BW). Simonsen et al.22 showed that no distinguishable side effects were observed after intravenous administration of a single dose of SPIONs (100 mg Fe per kg BW) to rats. In an ordinary biomedical application, the injected dosages of SPIONs are substantially lower than these attempts. All these results explained the safety of SPIONs in clinical applications.
However, previous studies also revealed that SPIONs may induce toxicity in various tissues. Feng et al.23 showed that SPIONs induce renal toxicity in rats following a single intravenous administration. Wu et al.24 reported that Fe3O4 NPs may cause oxidative damage in the brain striatum of rats after intranasal instillation of Fe3O4 NPs at a dose of 20 μg for 7 days. Although these studies suggest possible toxicities of SPIONs, this does not mean that SPIONs cannot be used for biomedical applications. SPIONs can be used in clinics for very short times at an appropriate dose. Employment of new SPIONs, such as Zn0.4Fe2.6O4 NPs, with high magnetic susceptibility and low toxicity is critical for their safety applications in clinics, as they can be used in vivo at a low dosage.
The results of the present study demonstrate that the Fe and Zn levels increase in the liver and kidney after sub-chronic oral exposure to Zn0.4Fe2.6O4 NPs. The increases of the Fe level in the liver and kidney are consistent with an investigation on tissue distribution of Fe in the mice receiving a single intragastric dose of Fe3O4 NPs (600 mg per kg BW),25 while the increases of the Zn level in the liver and kidney are consistent with an investigation on tissue distribution of Zn in rats following the administration of a single oral dose of ZnO nanoparticles (ZnO NPs) (50, 300 or 2000 mg per kg BW).16 Zn0.4Fe2.6O4 NPs are partially dissolved in gastric fluid and more than 96% of nanoparticles are not dissolved in gastric fluid after 4 h. The Zn0.4Fe2.6O4 NP gastric emptying time in mice is about 1 h (data not shown). It appears that Zn0.4Fe2.6O4 NPs are absorbed into the blood in ionic and/or particulate forms. The dissolved Fe and Zn ions will be absorbed through the small intestine,25 while the undissolved Zn0.4Fe2.6O4 NPs will be partly absorbed by the stomach26 and intestinal tract,27,28 and further distributed into various organs. The increases in Zn and Fe contents in the blood confirm that Zn0.4Fe2.6O4 NPs are absorbed into the blood in ionic and/or particulate forms.
The increases in the Fe and Zn levels in the liver and kidney did not cause marked oxidative stress in the two organs. It was reported that decreased levels of antioxidative enzymes are associated with increased oxygen free radical production.29 Sub-chronic oral administration of Zn0.4Fe2.6O4 NPs did not significantly affect the SOD activity but caused an increase in the GSH-Px activity in the liver, suggesting that Zn0.4Fe2.6O4 NPs did not cause marked oxidative stress in the liver. Sub-chronic oral administration of Zn0.4Fe2.6O4 NPs caused an increase in the SOD activity and a decrease in the GSH-Px activity in the kidney, suggesting that the antioxidant defense system in the kidney was disturbed by Zn0.4Fe2.6O4 NPs. The increases in the GSH-Px activity in the liver and the SOD activity in the kidney may be explained by compensatory response mechanism for the synthesis of more antioxidative enzymes to compensate for the oxidative stress. GSH-Px is involved in the conversion of the hydrogen peroxide generated, into harmless compounds. SOD protects organs against spontaneous oxygen toxicity and lipid peroxidation. The increases in the activities of the two antioxidant enzymes are usually induced by a slight oxidative stress. The above results indicate that Zn0.4Fe2.6O4 NPs induce a slight oxidative stress in the liver and kidney. GSH, a direct antioxidant, functions as a free radical scavenger for protecting the cell from oxidative damage.30 The quantity of GSH may increase through enhancement of its synthesis under slight oxidative stress. However, excess ROS production in response to xenobiotics can exhaust GSH and decrease its level in the organs under severe oxidative stress.31 The GSH levels in the liver and kidney significantly increased in the mice following sub-chronic oral administration of Zn0.4Fe2.6O4 NPs, further confirming that Zn0.4Fe2.6O4 NPs induce a slight oxidative stress in the two organs. The MDA content is an index of the level of oxidative stress in tissues. The increases in the MDA levels in the liver and kidney reveal that Zn0.4Fe2.6O4 NP-induced slight oxidative stress results in an increase in lipid peroxidation in the two organs.
The slight oxidative stress induced by Zn0.4Fe2.6O4 NPs did not affect the kidney function, as indicated by the fact that no obvious changes in the levels of CRE, BUN and UA were observed following oral administration of Zn0.4Fe2.6O4 NPs. Although the activities of AST, ALT and LDH increased after oral administration of Zn0.4Fe2.6O4 NPs, the three biochemical markers for hepatic function were all in the normal range. In addition, the ratio of AST/ALT, a sensitive index for hepatic damage, did not change significantly after oral administration of Zn0.4Fe2.6O4 NPs. Furthermore, no significant change was observed for the serum TBIL, which is used as a direct marker to evaluate liver function. All these results together indicated that although sub-chronic oral administration of Zn0.4Fe2.6O4 NPs caused slight increases in oxidative stress in the liver and kidney, this slight oxidative stress did not seem to be high enough to impair their functions and could be successfully counteracted by their intrinsic antioxidant systems. The slight oxidative stress did not affect tissue morphology and cell structure in the liver and kidney (Fig. 3 and 4). After sub-chronic oral administration of Zn0.4Fe2.6O4 NPs, no significant changes in the body weight and the coefficients of the liver and kidney also indicated that Zn0.4Fe2.6O4 NPs are biocompatible in mice.
Lin et al.32 reported that orally administered Fe3O4 NPs at a dose of 50 mg NPs per kg BW every other day for 4 weeks caused the infiltration of inflammatory and fatty degeneration in the liver of rats. Our results demonstrate that oral administration of Zn0.4Fe2.6O4 NPs at a dose of 50 mg kg−1 for 30 consecutive days does not induce obvious histopathological and ultrastructural abnormalities in the liver of mice, suggesting that doping Zn2+ into Fe3O4 NPs can attenuate Fe3O4 NPs toxicity in mice. It has been reported that high doses of Fe supplements inhibit Zn absorption in human, which causes Zn deficiency.33 The present data indicate that doping Fe3O4 NPs with Zn2+ increases Zn uptake in the liver and kidney and inhibits Fe-induced Zn deficiency in the two organs, which may play a role in attenuating Fe3O4 NPs toxicity in mice. Further investigation is necessary to clarify this issue.
ZnO NPs are well known to have high toxicity due to their ionization in biological fluids.34–38 The toxicity of ZnO NPs in rats and zebrafish can be alleviated by doping the ZnO NPs with Fe (10% Fe) as Fe doping changes the ZnO matrix to slow Zn2+ release in physiological saline.39 Our results show that Zn2+ doping of Fe3O4 NPs significantly decreases the level of Fe release in biological conditions. This enhancement of the stability of Zn0.4Fe2.6O4 NPs by Zn2+ doping makes them appropriate for use in magnetic resonance imaging and drug delivery by the oral route.
Seok et al.40 in their study with the 13-week chronic toxicity of ZnO NPs administered via the oral route at doses of 67.1, 134.2, 268.4 or 536.8 mg per kg per body reported that the no-observed-adverse effect level of ZnO NPs was found to be 268.4 mg per kg per day for rats. They also found that around 98% of the ZnO NP mass had dissolved in the acidic artificial gastric fluid (pH 1.7) after 24 h. The dose of Zn0.4Fe2.6O4 NPs used in the present study was 50 mg per kg, which was much lower than 268.4 mg per kg. In addition, only 3.9% Zn of ions in Zn0.4Fe2.6O4 NPs were released into acidic artificial gastric fluid (pH 1.5) after 4 h. Zn0.4Fe2.6O4 NPs were more stable in artificial intestinal fluid or artificial blood solution than in artificial gastric fluid. Based on these results, we deduce that the concentration of Zn2+ released from Zn0.4Fe2.6O4 NPs may not be high enough to elicit toxicity in mice.
The dosage of nanomaterials used in clinics is a critical matter for their safety applications. In fact, high amounts of SPIONs show a toxic effect. However, the lower amount of the same material is fully biocompatible.19 Zn2+ doped Fe3O4 NPs significantly enhance magnetic resonance imaging contrast. The nanoparticle probe dosage level can be lowered when using Zn0.4Fe2.6O4 NPs as probes, which is significant for the safety applications.
5. Conclusion
In summary, we have demonstrated that Zn0.4Fe2.6O4 NPs with a high saturation magnetization value have no apparent adverse effect on the liver and kidney of mice after one month of repeated intragastric administration. Zn0.4Fe2.6O4 NP-induced slight increase in oxidative stress in the liver and kidney can be successfully counteracted by their intrinsic antioxidant systems and have no obvious hazardous effects on their histopathology and functions. Zn2+ doping not only markedly enhances magnetic susceptibility of Zn0.4Fe2.6O4 NPs but also significantly increases the stability of Zn0.4Fe2.6O4 NPs in biological conditions, making them appropriate for use in magnetic resonance imaging and drug delivery by the oral route. These findings will be useful for future development of Zn2+ doped Fe3O4 NP based biomedical applications.
Declaration
The submission complies with the Helsinki Declaration with no information that has been published elsewhere. There are also no diagnostic testing and copyrighted material. The authors declare no potential conflicts of interest with respect to the authorship and/or publication of this article.
Disclosure
Shanshan Zhu and Xiaolong Xu contributed to the design of the whole study; Shanshan Zhu contributed to synthesis and characterization of Zn0.4Fe2.6O4 NPs; Shanshan Zhu, Rui Rong and Xue Wang contributed to the animal treatment, histopathological examination and measurements of biochemical parameters and oxidative stress makers; Bing Li, Xue Wang and Shanshan Zhu contributed to the biodistribution of Zn0.4Fe2.6O4 NPs in mice; Shanshan Zhu and Xiaolong Xu contributed to the proof-reading of the paper.
Acknowledgements
We would like to acknowledge the financial support from the National Natural Science Foundation of China (grant no. 21171157, 20871111 and 20571069).
References
- K. K. Jain, Med. Princ. Pract.: Int. J. Kuwait Univ., Health Sci. Cent., 2008, 17, 89–101 CAS.
- J. E. Rosen, L. Chan, D. B. Shieh and F. X. Gu, Nanomedicine, 2012, 8, 275–290 CrossRef CAS PubMed.
- A. K. Gupta, R. R. Naregalkar, V. D. Vaidya and M. Gupta, Nanomedicine, 2007, 2, 23–39 CrossRef CAS PubMed.
- H. Y. Wu, M. C. Chung, C. C. Wang, C. H. Huang, H. J. Liang and T. R. Jan, Part. Fibre Toxicol., 2013, 10 CAS.
- A. J. L. Villaraza, A. Bumb and M. W. Brechbiel, Chem. Rev., 2010, 110, 2921–2959 CrossRef CAS PubMed.
- S. Laurent, D. Forge, M. Port, A. Roch, C. Robic, L. V. Elst and R. N. Muller, Chem. Rev., 2008, 108, 2064–2110 CrossRef CAS PubMed.
- X. Liang, X. Wang, J. Zhuang, Y. Chen, D. Wang and Y. Li, Adv. Funct. Mater., 2006, 16, 1805–1813 CrossRef CAS.
- R. Colognato, A. Bonelli, D. Bonacchi, G. Baldi and L. Migliore, Nanotoxicology, 2007, 1, 301–308 CrossRef CAS.
- M. Bellusci, A. La Barbera, F. Padella, M. Mancuso, A. Pasquo, M. G. Grollino, G. Leter, E. Nardi, C. Cremisini, P. Giardullo and F. Pacchierotti, Int. J. Nanomed., 2014, 9, 1919–1929 Search PubMed.
-
R. Asmatulu, A. Garikapati, H. E. Misak, Z. Song, S. Y. Yang and P. Wooley, Proceedings of the ASME International Mechanical Engineering Congress and Exposition (IMECE 2010), 2012, vol. 10, pp. 911–918 Search PubMed.
- J. T. Jang, H. Nah, J. H. Lee, S. H. Moon, M. G. Kim and J. Cheon, Angew. Chem., Int. Ed., 2009, 48, 1234–1238 CrossRef CAS PubMed.
- M. H. Cho, E. J. Lee, M. Son, J. H. Lee, D. Yoo, J. W. Kim, S. W. Park, J. S. Shin and J. Cheon, Nat. Mater., 2012, 11, 1038–1043 CrossRef CAS PubMed.
- M. Arruebo, R. Fernández-Pacheco, M. R. Ibarra and J. Santamaría, Nano Today, 2007, 2, 22–32 CrossRef.
- H. M. Chen and R. Langer, Pharm. Res., 1997, 14, 537–540 CrossRef CAS.
- P. F. Hahn, D. D. Stark, J. M. Lewis, S. Saini, G. Elizondo, R. Weissleder, C. J. Fretz and J. T. Ferrucci, Radiology, 1990, 175, 695–700 CrossRef CAS PubMed.
- H. J. Paek, Y. J. Lee, H. E. Chung, N. H. Yoo, J. A. Lee, M. K. Kim, J. K. Lee, J. Jeong and S. J. Choi, Nanoscale, 2013, 5, 11416–11427 RSC.
- M. Guo, X. Xu, X. Yan, S. Wang, S. Gao and S. Zhu, J. Hazard. Mater., 2013, 260, 780–788 CrossRef CAS PubMed.
- M. E. Anderson and A. Meister, Proc. Natl. Acad. Sci. U. S. A., 1983, 80, 707–711 CrossRef CAS.
- M. Mahmoudi, H. Hofmann, B. Rothen-Rutishauser and A. Petri-Fink, Chem. Rev., 2012, 112, 2323–2338 CrossRef CAS PubMed.
- T. K. Jain, M. K. Reddy, M. A. Morales, D. L. Leslie-Pelecky and V. Labhasetwar, Mol. Pharmaceutics, 2008, 5, 316–327 CrossRef CAS PubMed.
- S. P. Singh, M. F. Rahman, U. S. Murty, M. Mahboob and P. Grover, Toxicol. Appl. Pharmacol., 2013, 266, 56–66 CrossRef CAS PubMed.
- C. Z. Simonsen, L. Ostergaard, P. Vestergaard-Poulsen, L. Rohl, A. Bjornerud and C. Gyldensted, Magn. Reson. Imaging, 1999, 9, 342–347 CrossRef CAS.
- J. Feng, H. Liu, K. K. Bhakoo, L. Lu and Z. Chen, Biomaterials, 2011, 32, 6558–6569 CrossRef CAS PubMed.
- J. Wu, T. Ding and J. Sun, Neurotoxicology, 2013, 34, 243–253 CrossRef CAS PubMed.
- J. Wang, Y. Chen, B. Chen, J. Ding, G. Xia, C. Gao, J. Cheng, N. Jin, Y. Zhou, X. Li, M. Tang and X. M. Wang, Int. J. Nanomed., 2010, 5, 861–866 CAS.
- C. M. Lee, H. J. Jeong, K. N. Yun, D. W. Kim, M. H. Sohn, J. K. Lee, J. Jeong and S. T. Lim, Int. J. Nanomed., 2012, 7, 3203–3209 CAS.
- N. Hussain, V. Jaitley and A. T. Florence, Adv. Drug Delivery Rev., 2001, 50, 107–142 CrossRef CAS PubMed.
- P. Jani, G. W. Halbert, J. Langridge and A. T. Florence, J. Pharm. Pharmacol., 1990, 42, 821–826 CrossRef CAS PubMed.
- B. Xu, Z. F. Xu, Y. Deng and J. H. Yang, Exp. Toxicol. Pathol., 2010, 62, 27–34 CrossRef CAS PubMed.
- P. V. Mohanan, C. S. Geetha, S. Syama and H. K. Varma, Colloids Surf., B, 2014, 116, 633–642 CrossRef CAS PubMed.
- X. S. Zhu, L. Zhu, Y. P. Lang and Y. S. Chen, Environ. Toxicol. Chem., 2008, 27, 1979–1985 CrossRef CAS PubMed.
- B. Lin, Z. Xi, Y. Zhang and H. Zhang, Weisheng Yanjiu, 2008, 37, 651–653 CAS.
- M. B. Zimmermann and F. M. Hilty, Nanoscale, 2011, 3, 2390–2398 RSC.
- I. De Angelis, F. Barone, A. Zijno, L. Bizzarri, M. T. Russo, R. Pozzi, F. Franchini, G. Giudetti, C. Uboldi, J. Ponti, F. Rossi and B. De Berardis, Nanotoxicology, 2013, 7, 1361–1372 CrossRef CAS PubMed.
- M. L. Fernandez-Cruz, T. Lammel, M. Connolly, E. Conde, A. I. Barrado, S. Derick, Y. Perez, M. Fernandez, C. Furger and J. M. Navas, Nanotoxicology, 2013, 7, 935–952 CrossRef CAS PubMed.
- T. Shaymurat, J. Gu, C. Xu, Z. Yang, Q. Zhao and Y. Liu, Nanotoxicology, 2012, 6, 241–248 CrossRef CAS PubMed.
- M. N. Croteau, A. D. Dybowska, S. N. Luoma and E. Valsami-Jones, Nanotoxicology, 2011, 5, 79–90 CrossRef CAS PubMed.
- A. Kermanizadeh, M. Lohr, M. Roursgaard, S. Messner, P. Gunness, J. M. Kelm, P. Moller, V. Stone and S. Loft, Part. Fibre Toxicol., 2014, 11 Search PubMed.
- T. Xia, Y. Zhao, T. Sager, S. George, S. Pokhrel, N. Li, D. Schoenfeld, H. Meng, S. Lin, X. Wang, M. Wang, Z. Ji, J. I. Zink, L. Madler, V. Castranova and A. E. Nel, ACS Nano, 2011, 5, 1223–1235 CrossRef CAS PubMed.
- S. H. Seok, W. S. Cho, J. S. Park, Y. Na, A. Jang, H. Kim, Y. Cho, T. Kim, J. R. You, S. Ko, B. C. Kang, J. K. Lee, J. Jeong and J. H. Che, J. Appl. Toxicol., 2013, 33, 1089–1096 CrossRef CAS PubMed.
|
This journal is © The Royal Society of Chemistry 2016 |
Click here to see how this site uses Cookies. View our privacy policy here.