Influence of chemical speciation and biofilm composition on mercury accumulation by freshwater biofilms†
Received
8th September 2016
, Accepted 17th November 2016
First published on 18th November 2016
Abstract
Mercury (Hg) is a pollutant of high concern for aquatic systems due to the biomagnification of its methylated form along the food chain. However, in contrast to other metals, gaining knowledge of its bioavailable forms for aquatic microorganisms remains challenging, making Hg risk assessment difficult. Ubiquitous and sessile freshwater biofilms are well known to accumulate and to transform Hg present in their ambient environment. The present study thus aims to evaluate whether non-extractable (proxy of intracellular) Hg accumulated by biofilms could be a good indicator of Hg bioavailability for microorganisms in freshwater. To that end, the link between Hg concentration and speciation, as well as biofilm composition (percentage of abiotic, biotic, chlorophyll and phycocyanin-fractions and abundance of dsrA, gcs, merA and hgcA bacterial genes) and biofilm Hg accumulation was examined. The studied biofilms were grown on artificial substrata in four reservoirs along the Olt River (Romania), which was contaminated by Hg coming from chlor-alkali plant effluents. The 0.45 μm-filterable Hg concentrations in ambient waters were measured and inorganic IHg speciation was modelled. Biofilms were analyzed for their non-extractable IHg and methylmercury (MeHg) contents as well as for their composition. The non-extractable IHg content was related, but not significantly, to the concentration of total IHg (r2 = 0.88, p = 0.061) whereas a significant correlation was found with the predicted IHg concentration that is not bound to dissolved organic matter (r2 = 0.95, p = 0.027), despite its extremely low concentrations (10−25 M), showing a limitation of the thermodynamic Hg modelling to predict Hg bioavailability. The studied biofilms were different in biomass and composition and a principal component analysis showed that the non-extractable IHg content correlated with the abundance of the merA and hgcA genes, while MeHg accumulation was only linked with the abundance of the rRNA 16S gene. The present study suggests that non-extractable IHg concentrations in biofilms are a useful proxy of IHg bioavailable forms in waters whereas the hgcA and merA genes are good biomarkers of both biofilm IHg exposure and bioavailability.
Environmental impact
Mercury (Hg) is a trace element of high toxicity for aquatic organisms, especially due to its methylated form that biomagnifies along the food chain. Biofilms, which are communities of microorganisms, are well known to accumulate and transform their ambient Hg. Here, we evaluate if biofilms could be used as bioindicators of Hg bioavailability through the analyses of their Hg accumulation. A clear relationship was found between the concentration and speciation of inorganic Hg (IHg) in ambient waters and the IHg concentration in biofilms grown in Hg contaminated reservoirs. That accumulation was related to the biofilm composition, notably with the presence of methylating and resistant bacteria. Biofilms could be potential bioindicators of Hg exposure in contaminated systems.
|
1. Introduction
Mercury (Hg) is classified as one of the most dangerous and toxic metals for aquatic organisms.1 Although present at very low concentration in natural waters (∼pM) and mostly in inorganic form (IHg), its methylated form (MeHg) is of high concern due to its biomagnification along the food chain.2 Elevated MeHg contents can thus be measured in higher organisms such as fish, which could induce negative effects in their consumers, including humans.3 Primary producers are the first entry point of Hg into autotroph-based trophic webs and as such will partly determine the degree to which MeHg will magnify along the food chains.4–6 In lotic and shallow waters, one important community of primary producers (mostly composed of microalgae) lives in biofilms, which colonize hard substrata (plants, rocks, etc.), and inhabit in symbiosis with heterotrophic microorganisms (bacteria and fungi).
Several field studies have reported IHg and MeHg accumulation by biofilms collected in either unperturbed or Hg contaminated sites.7–9 For example, very high Hg contents were measured in biofilms collected along a Slovenian River polluted by past Hg mining activities, with IHg content varying from 4.81 × 10−9 to 2.21 × 10−7 mol g−1 dry weight (gdw) and MeHg content varying from 5.43 × 10−11 to 1.93 × 10−9 mol gdw−1.10 An increase of Hg concentration in ambient water and sediment has been reported to usually lead to an increase of Hg content in biofilms but with some exceptions,7,11,12 which could be explained by chemical and biological factors. First, in natural freshwater, Hg is bound to a variety of particles, colloids, macromolecules and small ligands such as hydroxide, chloride or sulfide forming various complexes.13 Laboratory studies demonstrated that not all Hg forms are bioavailable for microorganisms. Small neutral lipophilic complexes (i.e. HgCl02 and MeHgCl0) were shown to be taken up by algae via passive diffusion14,15 although the involvement of active transport has also been demonstrated.16 In bacteria, and possibly in natural biofilms,17 the facilitated transport of Hg has been evidenced to be enhanced in the presence of specific low molecular weight thiols,18–20 whereas dissolved Hg and nanoparticulate HgS were found to induce more methylation than microscale HgS in a model Hg-methylating bacterium.21,22 The physico-chemical composition of ambient waters might thus modulate biofilm Hg accumulation. Furthermore, Hg accumulation by biofilms might also depend on their composition. A negative relationship was observed between the autotrophic index (ratio between the ash free dry weight and chlorophyll a content) of epiphytic communities collected in a large fluvial lake and their Hg content,23 demonstrating the importance of the algal fraction in biofilm Hg accumulation. Finally, microorganisms inhabiting biofilms could transform accumulated Hg, which will affect its relation with ambient Hg concentrations. Indeed, sulfate-reducing bacteria (SRB)24,25 and iron-reducing bacteria (IRB)26 as well as methanogens27 were shown to methylate IHg, which might lead to the increase of MeHg concentrations in biofilms whereas, in contrast, demethylation is also known to occur within biofilms, reducing accumulated MeHg and increasing IHg concentrations in biofilms.27 Biofilms exposed to contaminated Hg water were characterized by a high abundance of the merA gene, which encodes for the enzyme involved in Hg resistance through IHg reduction28 whereas microalgae are also known to reduce accumulated IHg as a detoxification mechanism.29 Therefore the presence of bacteria and algae, which are able to reduce IHg, could decrease accumulated IHg concentrations within biofilms.
Besides Hg, biofilms are known to be good accumulators of metals such as Cu, Zn, Cd and Pb,30,31 whose accumulation has been demonstrated to be very rapid (within minutes of exposure) and to be linked with their ambient speciation, notably their free ion as well as labile species concentrations.32,33 Furthermore biofilms possess several interesting characteristics (ubiquity, sessility, ease of collection and large abundance), which make them useful bioindicators of metal bioavailability for microorganisms in natural waters. The present study thus aims to bring insights into the possible use of Hg accumulation in biofilms as an indicator of Hg bioavailability for microorganisms and to evaluate how the biofilm composition relates to Hg accumulation. The knowledge of Hg bioavailability (e.g. “the extent of absorption of a substance by a living organism”34) for microorganisms is crucial to accurately assess Hg impacts towards aquatic organisms. To that end, we explored the relationships between ambient Hg concentration, Hg accumulated by biofilms and the biofilm composition of in situ grown biofilms in the Olt River (Romania), which is known to be contaminated by Hg-rich effluents coming from a chlor-alkali production plant.35 Waters collected in four specific reservoirs (one reference and three downstream of the Hg point source) were examined for their physico-chemical parameters as well as for dissolved Hg concentrations. Biofilms were grown for three weeks on artificial substrata deployed in the studied reservoirs and analyzed for their non-extractable (proxy of intracellular) IHg and MeHg contents as well as for their composition. Their abiotic and biotic fractions were quantified using microscopy whereas the abundance of five bacterial genes related to Hg transformation was examined using quantitative polymerase chain reaction (qPCR). Correlations were drawn between dissolved Hg concentration and modelled speciation, Hg bioaccumulation and biofilm composition to infer conclusions on their significance for the prediction of Hg accumulation by biofilms.
2. Materials and methods
2.1 Sampling sites
The field campaign took place in September 2013 in the Olt River (Romania), which is situated in the South Carpathian region and is the longest and principal tributary of the Danube River in Romania. Its natural morphology was strongly modified with the construction of 19 dams for flood management and hydropower along its 615 km length. Its watershed is under high anthropogenic pressure, notably in the region of Rm Valcea, which possesses several industrial companies, including a large chlor-alkali plant.36 The Hg released by the platform reaches the Olt River through the Govora River into the Babeni Reservoir (a surface area of 8.5 km2 and maximum depth of 16 m) (Fig. 1). The three downstream reservoirs of the Babeni Reservoir are the Ionesti (a surface area of 4.6 km2 and maximum depth of 12 m), the Zavideni (a surface area of 10.9 km2 and maximum depth of 14 m) and the Dragasani (a surface area of 12.2 km2 and maximum depth of 16 m) Reservoirs. Previous experiments evidenced the contamination of the Babeni Reservoir by Hg.35–37 In 2009, the Govora River was found to bring 88 ng L−1 Hg into the Babeni Reservoir water, in which the dissolved Hg concentration decreased by the dilution effect from 8.8 ng L−1 in the upstream part of the reservoir to 2.9 ng L−1 in the downstream part, close to the dam.35 In contrast, the MeHg concentration increased from 0.15 ng L−1 to 0.42 ng L−1 toward the dam due to in situ methylation.35 The propagation of Hg contamination downstream the chemical platform was also recorded in the sediment compartment, with a 26- and a 6-fold increase of Hg concentration in the Babeni and Ionesti Reservoir sediment, respectively, compared to the natural background measured in the Valcea Reservoir (a surface area of 2.9 km2 and maximum depth of 16.5 m) located upstream of the chlor-alkali platform and used as a reference site.
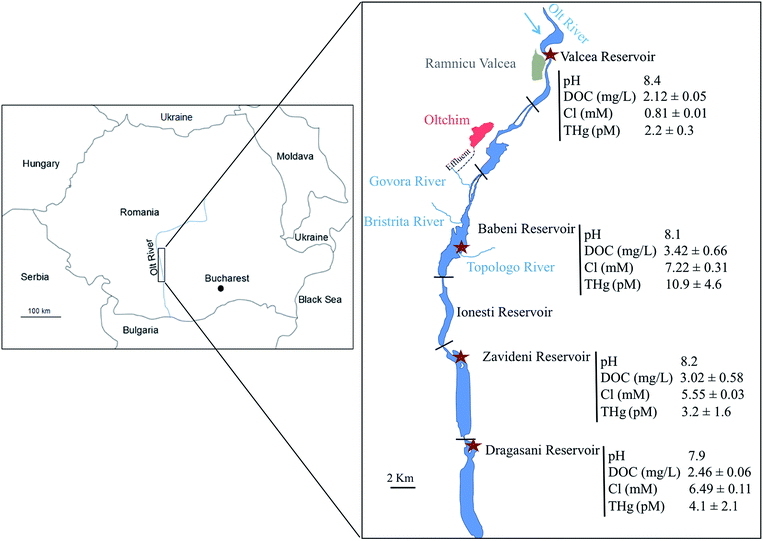 |
| Fig. 1 Map showing a part of the Olt River (Romania) with the four sampling sites (brown stars) and their related physico-chemical characteristics (pH, concentrations of dissolved organic carbon (DOC) and chloride (Cl); mean ± s.d., n = 3), including the concentrations of dissolved Hg (THg), measured in September 2013. The chlor-alkali plant (Oltchim) is presented in red, with its effluent directly connected to the Govora River. | |
2.2 Biofilm colonization
Artificial substrata for biofilm colonization were placed for three weeks in the upstream (Valcea, used as the control site) and downstream (Babeni, Zavideni and Dragasani) reservoirs of the chlor-alkali plant, in order to obtain a gradient of Hg concentrations in ambient water (Fig. 1). Setups were fixed at about 1 m water depth and at less than 10 m from the reservoir shore to obtain similar conditions of exposure (current flow, light penetration, etc.) at each sampling site. The setups were made of two plastic arms, which held a total of 24 microscopy glass slides (7.6 × 2.6 cm), pre-pyrolyzed for 2 hours at 475 °C. The use of artificial substrata made of glass had several advantages in our study, i.e. the pyrolysis treatment allowed us to remove Hg and possible microbes, to directly process our microscopic analyses without damaging or losing biofilms and to limit inorganic particles and dead microorganisms in the biofilms. After the colonization period, microscopic slides were retrieved and processed back in the laboratory for the determination of Hg accumulation and composition.
2.3 Water analysis
At each sampling site, pH was measured using a multi-parameter probe YSI 600 R (Yellow Springs, OH) and water was sampled at 1 m depth using a grabber equipped with a Teflon bottle facing upstream for anion and cation analysis. To that end, 10 mL of natural waters were collected in 10 mL polypropylene (PP) tubes after filtration on 0.45 μm Sterivex filters (poly(ether)sulfone, Millipore, Schaffhausen, Switzerland) and measured by ion chromatography (Dionex ICS-3000, Thermo Fisher Scientific Inc., Waltham, MA). Samples were also collected for the determination of dissolved organic concentrations (DOC) in 550 °C-burned glass bottles after filtration on pre-pyrolyzed 0.7 μm GF/F filters (GE Healthcare Life Sciences, Glattbrugg, Switzerland) and acidified with hydrochloric acid (HCl, analytical grade, Merck, Zug, Switzerland) at 2 M. DOC measurements were performed with a Shimadzu TOC-5000A (Kyoto, Japan). All samples were kept at 4 °C in the dark until analysis.
2.4 Hg analysis in ambient water and its modelled speciation
The preparation of the water sampling material for Hg analysis is given in the ESI, Section S1.† Sampled water was analyzed for total dissolved Hg [THg]. 125 mL of water were filtered on 0.45 μm Sterivex filters, acidified to 1% (v/v final concentration) with HCl (Ultrex, J.T. Baker®, VWR, Nyon, Switzerland) and stored in Hg-cleaned Teflon bottles in the dark and at 4 °C prior to analyses. [THg] was measured after bromine monochloride oxidation and stannous chloride reduction steps by cold vapour atomic fluorescence spectrometry (CV-AFS) using a Tekran (Model 2500) mercury detector38 following the USEPA method 1631.39 All water samples were measured in triplicate and 1.7 ± 0.3 pM [THg] was measured in the field blanks. The analytical detection limit was 1.8 pM, calculated as three times the standard deviation (SD) of 10 blank runs. The certified reference material ORMS-5 (National Research Council Canada) was used for quality control and had a mean recovery of 102 ± 26% (n = 10). The analytical stability was tested by analysing a standard every 7 samples (99 ± 16%).
The speciation of the dissolved IHg was modelled by assuming that 100% of [THg] was in IHg form, neglecting elemental [Hg0] and [MeHg], which was previously measured to vary between 2 and 12% of [THg] in the Babeni Reservoir.35 The calculation was performed with the Windermere Humic Aqueous Model (WHAM) VII allowing to run simulations with natural organic matter.40 Its default database was updated for OH−, Cl−, SO42− and CO32− binding constants41 (see ESI, Table S1†) whereas the default formation constants for the complexation of IHg to humic and fulvic acids were kept. A ratio between DOC and DOM of 2 was taken and DOM was assumed to be composed of 90% of fulvic acids and 10% of humic acids.42
2.5 Mercury accumulation by biofilms
The total accumulated Hg content {THg} which represents the sum of Hg adsorbed onto living organisms and particles, bound to exopolymeric substances in the biofilm matrix and intracellularly accumulated by microorganisms, was determined in collected biofilms rinsed with the Valcea Reservoir water. To assess the intracellular (hereafter called non-extractable) Hg content, {THg}n-ext, biofilms were rinsed with 100 mM cysteine (>97%, Sigma-Aldrich, Busch, Switzerland) for 10 min (ESI, Fig. S1†).43 The slides were then scraped and collected in pre-cleaned PP tubes to be freeze-dried. The content of accumulated Hg with and without the rinsing step was determined with an Advanced Hg Analyser AMA 254 (Altec s.r.l., Czech Republic). The detection limit of Hg measurements was 0.05 ng (3 SD of 10 blanks). The accuracy of the AMA measurements was verified using two reference materials (TORT2 and MESS3, National Research Council, Canada) and measurements were accepted only when their excepted concentrations were in the range of the certified materials. Accumulated {MeHg}n-ext was further extracted in cysteine-rinsed biofilms following the method of Liu et al.44 Briefly, a mixture of 2 mL of 1 M copper sulfate, 5 mL of 5 M HNO3 and 10 mL of dichloromethane was added to ∼ 5 mg freeze-dried biofilms. The samples were then shaken for 1 hour and subsequently centrifuged for 25 min at 3000 rpm. The solvent layer was removed and the samples were back-extracted into water at 50 °C under N2 bubbling for 30 min. Finally, the samples were stored at 4 °C in the dark for 12 hours and then analyzed by PT-GC-Pyr-AFS (MERX automated methylmercury systems, Brooks Rand, Seattle, USA) after the addition of acetate buffer and ethylation with sodium tetraethylborate (NaBEt4) following the USEPA method 1630.45 The analytical detection limit was 4.4 nM (3 SD of 10 blanks). The ERMCC580 estuarine sediment (75.5 ± 3.7 ng g−1) was used as a certified material and was recovered at 66.9 ± 0.2% (n = 3). No correction was further applied to Hg accumulation data. All samples were analyzed in triplicate. The non-extractable IHg content {IHg}n-ext was calculated by subtracting the measured {MeHg}n-ext from {THg}n-ext.
2.6 Biofilm composition
Biofilms were stained and fixed according to the studied fraction following the procedure detailed elsewhere (ESI, Fig. S2†).46 Briefly, slides for the analysis of the surface coverage, abiotic fractions (proxy of inorganic particles), chlorophyll- (proxy for microalgae) and phycocyanin- (proxy for cyanobacteria) fractions were only fixed with 100 μL of 3.7% formaldehyde (Sigma-Aldrich, Buchs, Switzerland) and 50 μL of Mowiol47 for microscopy analysis. Slides for the biotic fraction (proxy for biomass) analysis were stained with 10 μg mL−1 of 6-diamidino-2-phenylindodihydrochloride (DAPI, Sigma-Aldrich, Buchs, Switzerland) before the fixation step. All slides were then observed with a fluorescence microscope (Olympus BX61, OLYMPUS, Volketswil, Switzerland) equipped with a digital camera (XC30, OLYMPUS, Volketswil, Switzerland) and the CELLSENS software (Cellsens dimension, OLYMPUS, Volketswil, Switzerland). The percentage of the non-covered slide area (NCA) and abiotic fractions were determined using the DAPI channel. The abiotic fraction was determined by quantifying elements that emit turquoise fluorescence in the DAPI channel. The percentage of the covered area (CA) was then calculated based on eqn (1):
The biotic fraction (in blue) was also observed with the DAPI channel whereas chlorophyll- (in red) and phycocyanin- (in orange) containing fractions were examined with the DAPI and TRITC channels, respectively. Other fractions, which represent non-phototrophic organisms such as bacteria and fungi, were calculated by subtracting the chlorophyll- and phycocyanin-containing fractions from the biotic fraction. Microscopic images were analyzed with the software “Image J”.48 Measurements of the hue, saturation and brightness values were used to distinguish and quantify between the different fractions.
The abundance of specific functional genes present in biofilms was examined by qPCR. DNA was extracted from biofilms (∼10 mg) using a Power Biofilm DNA kit (Mo-Bio, Canada) following the instructions of the providers. The quality of the extracted DNA was measured (see ESI, Section S2,† for details of the DNA extraction and qPCR procedure) and the abundance of the studied genes was analysed using an Eco cycler (Illumina, San Diego, CA) and KAPA SYBR® FAST qPCR Universal kit (Kapabiosystems, Wilmington, MA). The qPCR procedures are further detailed in the ESI, Section S2.† Primers for qPCR were described previously49 to target sulfate-reducing bacteria (dsrA), Geobacteraceae (gcs), and Hg resistant (merA) and Hg-methylation (hgcA) related genes (ESI, Table S2†).
2.7 Statistical analysis
One-way ANOVA was used to compare the average values of accumulated Hg in biofilms, of biotic and abiotic fractions, of the relative copy number of rRNA 16S, merA, hgcA, dsrA and gcs genes between reservoirs. When the null hypothesis was rejected at α = 0.05, the Tukey's Honestly Significant Difference (HSD) test was applied. Principal component analysis (PCA) was performed to identify correlations between the biofilm composition and the percentage of Hg content in biofilms using the software R.50
3. Results
3.1 Hg concentrations and modelled speciation in the studied reservoirs
Dissolved THg concentration measured in the reference reservoir (Valcea) was low with a value of 2.2 ± 0.3 pM (Fig. 1). In contrast, the Govora River, which received the direct release from the chlor-alkali platform and flows in the Babeni Reservoir, was highly contaminated with a [THg] of 384 ± 183 pM. Consequently, about 5-fold increase of the [THg] in the Babeni Reservoir (10.8 ± 4.6 pM) was found as compared with the Valcea reference reservoir. [THg] decreased in the reservoirs downstream of Babeni, with measured values of 3.2 ± 1.6 pM and 4.1 ± 2.1 pM in the Zavideni and Dragasani Reservoirs, respectively. Nonetheless, [THg] in the Dragasani Reservoir was still significantly higher than that measured in the reference reservoir (P < 0.05).
Besides Hg contamination, the release of the chlor-alkali platform also modified the water quality characteristics of its downstream reservoirs (Fig. 1). The water of the reference reservoir (Valcea) was characterized by an average pH of 8.4, a DOC concentration of 2.12 ± 0.05 mg L−1 and a Cl concentration of 0.81 ± 0.01 mM. In contrast, the water from the Babeni Reservoir contained 9 times more Cl, a larger quantity of DOC (3.42 ± 0.66 mg L−1) and a pH of 8.1. The downstream reservoirs, Zavideni and Dragasani, also had lower pHs than the Valcea Reservoir (8.2 and 7.9, respectively). The concentration of DOC remained high in the Zavideni Reservoir (3.02 ± 0.58 mg L−1) but returned to similar concentrations to that of the control reservoir in the Dragasani Reservoir (2.46 ± 0.57 mg L−1). Chloride concentrations were still about 7 times and 8 times higher in the Zavideni and Dragasani Reservoirs than that in the Valcea Reservoir, respectively.
The speciation modelling revealed that Hg speciation changed with the increase of [THg] along the Olt River (ESI, Fig. S3†). Based on our assumptions, dissolved IHg was predicted to be mainly present in Hg-DOM forms (>99.9%) with Hg-fulvic and Hg-humic complexes representing about 85% and 15% of the complexes, respectively, in all the reservoirs (ESI, Fig. S3a†). However, the speciation of the non-DOM-bound bound fraction was strongly influenced by the variation of Cl concentrations in the sampling sites. In the Govora River, IHg speciation was dominated by chloro-complexes (HgCl42− and HgCl3−) rather than by the hydroxo-complexes. Predominant IHg species were predicted to be Hg(OH)2 in the Valcea Reservoir representing 95% of the non-DOM-bound complexes, whereas in the downstream reservoirs, HgCl2 and HgOHCl also contributed to the main complexes with a proportion of 17% and 36%, respectively (ESI, Fig. S3b†).
3.2 Hg accumulation by biofilms
Biofilms collected in the Valcea Reservoir accumulated 269 ± 6 pmol gdw−1 {THg}, which increased by about 5 times in biofilms from the Babeni Reservoir, to return to similar concentrations in the Zavideni Reservoir and to increase back by about 3 times in the Dragasani Reservoir (Fig. 2). {IHg}n-ext was 167 ± 3 pmol gdw−1 in the reference reservoir and increased by 6-fold in biofilms collected in the Babeni Reservoir (Fig. 2), with an increase in the proportion of {IHg}n-ext to {THg} from 62% in Valcea to 75% in Babeni. Biofilms grown in the Zavideni Reservoir contained a similar quantity of {IHg}n-ext to those grown in the Valcea Reservoir whereas those collected in the Dragasani Reservoir had higher {IHg}n-ext. A value of 1.18 ± 0.09 pmol gdw−1 {MeHg}n-ext was obtained in Valcea biofilms, which was similar to the value found in Zavideni biofilms. A two-fold increase was observed in biofilms collected in the Babeni Reservoir, which was comparable with concentrations measured in Dragasani biofilms. The proportion of {MeHg}n-ext to {THg} varied between 0.17% (Zavideni) and 0.71% (Dragasani).
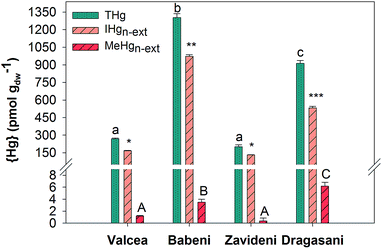 |
| Fig. 2 Total THg, non-extractable IHg {IHg}n-ext and MeHg {MeHg}n-ext content (pmol gdw−1) in biofilms grown for 3 weeks in the Olt River Reservoirs. Significant differences between the reservoirs are indicated with letters and asterisks (p < 0.05). | |
3.3 Biofilm composition
Biofilm biomass and composition changed according to the studied sites (Fig. 3). The slide surface coverage was used as a measure of biofilm biomass and was comparable in the Valcea, Zavideni and Dragasani Reservoirs, but was about twice lower in the Babeni Reservoir (Fig. 3a). Analyses of the biotic fractions further evidenced that biofilm microorganisms in the Babeni Reservoir represented only 44 ± 10% of the covered area (Fig. 3b). For comparison, in the non-impacted reference site, the biotic fraction represented 90 ± 31% of the covered area. Biofilms grown in the Babeni Reservoir had a 4-fold larger abiotic fraction than those from the other reservoirs. Chlorophyll-containing microorganisms were 4 times more abundant in biofilms grown in the Babeni and Dragasani Reservoirs than in the Valcea and Zavideni Reservoirs (Fig. 3c). Phycocyanin-containing fractions were higher in biofilms from the sites downstream the industrial platform than in the Valcea Reservoir (0.77 ± 0.3%). In the Hg-impacted reservoirs, their proportion decreased in the order Babeni > Dragasani > Zavideni. Other fractions, containing bacteria, fungi and unidentified group of organisms, were about three-fold higher in biofilms from the Valcea and Zavideni than in the Babeni and Dragasani Reservoirs.
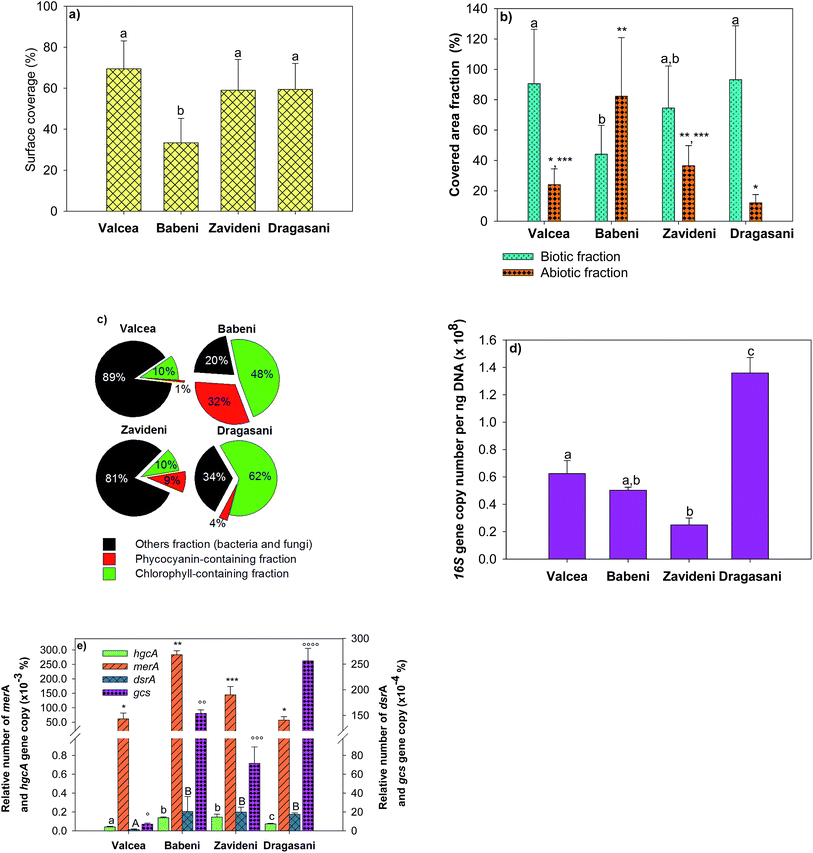 |
| Fig. 3 Composition of biofilms collected in the four studied reservoirs on the Olt River during the field campaign in 2013 with (a) the surface coverage of the substrata, (b) the percentage of biotic and abiotic fractions normalized to total substrata surface coverage, (c) chlorophyll, phycocyanin and other (bacteria, fungi and other unidentified organisms) containing fractions expressed as a percentage of the biotic fraction; (d) rRNA 16S gene copy number per ng of DNA and (e) relative dsrA, gcs, hgcA and merA gene copy numbers per rRNA 16S gene copy number. Asterisks and letters indicate a significant difference between reservoirs (p < 0.05). | |
Bacteria were further investigated using quantification of specific functional genes. The 16S rRNA gene copy number, representing the whole bacteria population, decreased downstream the Valcea reservoir to increase back in the Dragasani reservoir (Fig. 3d). The presence of sulfate-reducing bacteria and iron-reducing Geobacteraceae known to be potentially involved in Hg methylation was examined using the dsrA gene and the gcs gene, respectively. The proportion of both genes normalized by the abundance of the 16S rRNA gene was higher in biofilms from the Babeni, Zavideni, and Dragasani Reservoirs than in the Valcea Reservoir (Fig. 3e). The abundance of the Hg methylation related gene hgcA was also observed to increase in biofilms collected downstream the source of Hg pollution. Finally, the relative copy number of the Hg resistance merA gene was also higher in biofilms collected in the polluted reservoirs than in biofilms grown in the Valcea Reservoir.
3.4 Relationships between Hg accumulation and modelled Hg speciation
Relationships were drawn between {IHg}n-ext, and the predicted concentrations of [IHg] and [IHg]n-DOM (here defined as the fraction that is not complexed by DOM) to get insight into which Hg fraction better predicts Hg accumulation in biofilms. A positive correlation was found between [IHg] and {IHg}n-ext (r2 = 0.88, p = 0.061) (Fig. 4a) whereas a significant correlation was obtained between [IHg]n-DOM and {IHg}n-ext (r2 = 0.946, p = 0.027) (Fig. 4b).
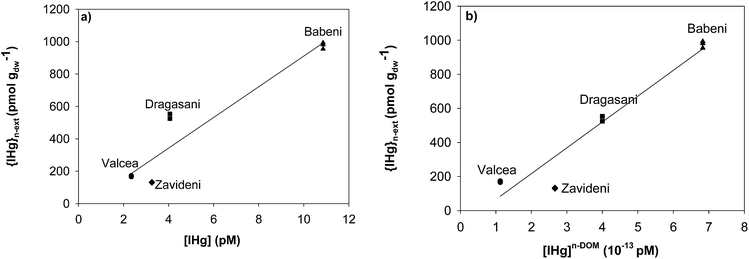 |
| Fig. 4 Non-extractable IHg {IHg}n-ext in biofilms (pmol gdw−1) as a function of (a) [IHg] and (b) [IHg]n-DOM (not bound to DOM) in ambient waters. | |
3.5 Relationship between Hg accumulation and biofilm composition
To evaluate the link between the biofilm composition and IHg and MeHg accumulation, a PCA was performed using the {IHg}n-ext and {MeHg}n-ext expressed as a percentage of the total Hg content (P-IHgn-ext, and P-MeHgn-ext) and the studied biofilm components (Fig. 5). The first axis explained 58.04% of the variability and the second 33.79% of the variability among the data. P-IHgn-ext was found to positively correlate with the relative abundance of merA and hgcA genes in the biofilms and to be negatively related to the biotic fraction. P-MeHgn-ext was only positively associated with the abundance of the rRNA 16S gene (Fig. 5).
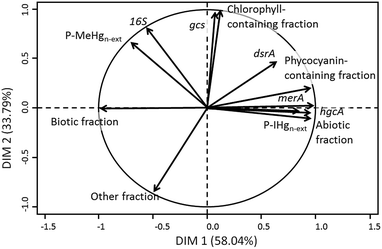 |
| Fig. 5 Principal component analysis of the percentage of non-extractable IHg (P-IHgn-ext), MeHg (P-MeHgn-ext) in biofilms and the biofilm composition i.e. biotic fraction, abiotic fraction, phycocyanin- containing fraction, chlorophyll-containing fraction, other fractions, rRNA 16S gene copy number (16S), relative gcs gene copy number (gcs), relative dsrA gene copy number (dsrA), relative hgcA gene copy number (hgcA) and relative merA gene copy number (merA). | |
4. Discussion
The [THg] measured in the reference reservoir of Valcea in the Olt River was comparable with those previously found during field campaigns performed between 2007 and 200935 and confirmed the observed degradation of the water quality in its downstream reservoirs. The release of the Oltchim Company is a well-known source of Hg pollution in the Rm Valcea region,36 which increased Hg concentration in the water column as far as several tens of kilometers down to the fourth reservoir. A recent study further observed that wide dispersion along the contaminated reservoirs with elevated Hg concentrations in sediments and seston.49 Chlor-alkali industries are an important source of Hg contamination for aquatic systems.51 The level of Hg pollution measured close to these industries could be as high as 1.4 μg L−1 (7 nM)52 for total Hg concentrations and 3 ng L−1 (15 pM)53 for dissolved Hg concentrations, which is comparable with the concentrations found during our field campaign (Fig. 1). The Oltchim Company has been using Hg-based electrodes since 1968 and has partly switched to Hg-free technologies in 198436 to reduce its negative impacts on the surrounding environment. A previous study has shown the decrease of Hg input in the Babeni Reservoir since that shift in the electrode material.36 A 2-fold decrease was further observed in the present study, which could be due to the reported slowdown of the chlor-alkali platform activities.54
In 2013, the increase of [THg] downstream the chlor-alkali plant led to a significant increase of accumulated Hg in biofilms, which was mostly found to be intracellular (more than 60%), demonstrating that a large part of THg present in the studied reservoirs was bioavailable for microorganisms. Assuming that all THg was in IHg form, a positive correlation was found between [IHg] and {IHg}n-ext, however not significant. Besides the possible flaw in our assumption, one additional explanation for that lack of significance might come from the change in the dissolved IHg speciation (i.e. bioavailable forms) in the ambient waters. Indeed, besides Hg, the release of Cl by chlor-alkali platforms is another known pollutant, which comes from the use of sodium chloride and calcium chloride in the chlor-alkali process.52,55
That modification of the water chemistry is predicted to directly impact the dissolved Hg speciation, notably the part of Hg that is not bound to DOM (ESI, Fig. S3b†). The predominance of hydroxo-Hg complexes (Hg(OH)2) in the water of the reference reservoir is calculated to decrease in favour of the chloro-Hg complexes (HgOHCl and HgCl2) in the impacted waters. Among the tested regression analysis performed between {IHg}n-ext and the concentrations of the different IHg complexes in the ambient biofilm water (data not shown), a weak correlation was found between {IHg}n-ext and [HgCl02] (r2 = 0.67, data not shown), in agreement with evidence showing that the early assumption of the passive diffusion of the small Hg neutral complexes as the main Hg uptake mechanism in microorganisms is partly inaccurate.16,20 The distinction between Hg bound to DOM and not bound to DOM significantly improved the relationship between [IHg] and {IHg}n-ext, suggesting that Hg forms that are not bound to DOM might drive {IHg}n-ext in our studied biofilms. DOM has already been reported to reduce Hg accumulation by model bacteria56 and microalgae57 through its complexation and thus decrease of bioavailability. Nevertheless, DOM present in the reservoir waters is predicted to highly control dissolved IHg speciation with more than 99.9% of IHg predicted to be bound to DOM, leading to extremely low values of [IHg]n-DOM (around 10−25 M). The accumulation of IHg by biofilms cannot thus be limited to the only non-DOM-bound Hg fraction present in the water. The limitation of the thermodynamic modelling of dissolved Hg (<0.45 μm) has recently been pointed out with its failure to explain the increase of Hg methylation in anaerobic bacteria in the presence of nano-HgS.22,58 In that case, kinetically labile Hg species rather than modelled Hg forms at equilibrium were suggested to be better predictors of Hg bioavailability for bacteria. Moreover, that study highlighted the need to distinguish between the truly dissolved (<10 kDa) and colloidal (fraction between 10 kDa and 0.45 μm) fractions to explore microbial Hg methylation. Refinement in the modelling would also be of great interest taking into account the quality of the DOM, which might influence the affinity of Hg for DOM.21,59
Besides speciation, we also tested if the biofilm composition and functionality might be related to the amount of Hg accumulated by biofilms. Indeed, {IHg}n-ext and {MeHg}n-ext are both the resultants of IHg and MeHg accumulated from their ambient environment and of their transformations occurring within biofilms. The studied biofilm biomass and composition were strongly modified according to their living reservoirs. For example, the biomass of biofilms grown in the most contaminated reservoir was observed to be twice lower than that of biofilms grown in the reference reservoir, which might suggest a direct effect of the water quality degradation towards the biofilms (Fig. 3a). To clearly state that this impact is due to Hg contamination remains however challenging, due to the numerous parameters that could affect biofilm biomass such as for example, the presence of herbivores or other potential contaminants that are produced by the chemical platform (chlorinated organic products, pesticides, etc.).60
One of the first transformations that could modify accumulated IHg and MeHg concentrations is their (de)methylation occurring in anoxic microzones of the biofilms, which are known to be present in both anoxic and oxic environments,61,62 and where methylating microorganisms can thrive. Both dsrA and gcs genes were found in higher quantity in the contaminated reservoirs as compared with the reference one, suggesting the presence of SRB and IRB as well as reduced microzones known to be favourable for Hg methylation. Indeed, the dsrA gene encodes for the dissimilatory sulfite reductase, a key enzyme involved in the anaerobic sulfate respiration of bacteria and thus allows the identification of SRB.63 The presence of Geobacteraceae (the genus of IRB able to methylate Hg) was assessed with the specific metabolic gcs gene encoding for the citrate synthase.64 SRB,7,25,65 geobacter,26,66 and methanogens27 have been evidenced to methylate Hg in periphyton and sediments. The higher abundance of genes related to SRB and Geobacteraceae in the Hg contaminated biofilms might suggest the enhancement of microenvironments favourable for MeHg production. However, no correlation has been obtained between both P-IHgn-ext and P-MeHgn-ext with both dsrA and gcs genes (Fig. 5). In contrast, the abundance of the hgcA gene found in methylating bacteria67 was observed to be positively correlated with P-IHgn-ext (Fig. 5). A recent study conducted in the sediments of the same reservoirs also evidenced the link between Hg bioavailability and the hgcA abundance49 whereas a positive correlation between the hgcA abundance and MeHg concentration was found in soils collected in an Hg mining area in China.68 The abundance of the hgcA gene in our studied biofilms thus allows us to make the hypothesis that methylation might partly modulate IHg and MeHg accumulation in the studied biofilms.
Another transformation that can affect accumulated IHg is its reduction into elemental Hg0. Hg resistant bacteria were found to be favoured in the impacted reservoirs as revealed by the large number of merA gene copies. The merA gene is involved in the bacterial Hg resistance by the reduction of accumulated IHg into the volatile Hg0.69 Its strong correlation with P-IHgn-ext (Fig. 5) may suggest that reduction of accumulated IHg occurred in our studied biofilms and that chronic exposure to Hg shifted biodiversity and functionality towards increasing Hg tolerance in the exposed biofilms. The merA was found to be widespread in Hg-exposed epiphytic communities living on macrophytes collected from various locations in the US.70 The abundance of this gene was also strongly correlated with total Hg concentrations measured in biofilms exposed to a gradient of Hg pollution in the Idrijca River, but at much higher concentrations of accumulated Hg (between 5 and 295 nmol gdw−1).28 Indeed, in contrast to our study, no correlation was found between the abundance of the mer A gene and the total accumulated Hg at lower concentrations than 5 nmol gdw−1. Our study suggests that the reduction of IHg might also take place at the pM level of [THg].
Finally, a negative relationship was obtained between P-IHgn-ext and the percentage of the biotic fractions, suggesting that a higher proportion of microorganisms in a biofilm would lead to a lower non-extractable IHg concentration. That phenomenon could be due to several interrelated effects. Firstly, a dilution effect could occur within biofilms, decreasing the intracellular Hg content in microorganisms. Such an effect is well documented for phytoplankton, and noticeable in eutrophic lakes and during algal bloom.71 A dilution effect in accumulated Hg and MeHg has also been reported in biofilms grown under several conditions of light and displaying different growth rates.72 Secondly, Hg tolerant algal species have been observed to be smaller than sensitive species,73 which would result in a lower algal fraction colonizing the substrata in Hg contaminated reservoirs.
5. Conclusion
The present study demonstrates that the thermodynamic modelling of inorganic IHg forms has limited applications to predict Hg bioavailability in our Hg contaminated system. The measurement of {IHg}n-ext in biofilms is however a proxy of interest to evaluate Hg bioavailability. Moreover, specific functional genes such as merA and hgcA represent good biomarkers of IHg bioavailability and as such of Hg exposure for microorganisms. The assessment of MeHg bioavailability for microorganisms was proved to be more challenging and further research is needed to examine the parameters controlling its bioaccumulation.
Acknowledgements
This work is part of the Romanian-Swiss Research Programme with the project number IZERZO-142228, funded by the Swiss National Science Foundation (SNF) and Romanian Executive Agency for Higher Education, Research, Development and Innovation Funding (UEFISCDI). We gratefully acknowledge J. L. Loizeau (University of Geneva), C. Ungureanu, G. Ungureanu and M. Zazu from GeoEcoMar as well as the National Administration Apele Romana Olt for their help during the field campaign. We also thank E. Roden (University of Wisconsin-Madison) and B. Converse (University of Wisconsin-Madison) for providing bacterial genomic DNA, Alexandre Poulain (University of Ottawa) for providing pJMY2 plasmid, D. Ariztegui (University of Geneva) for the guidance for the fabrication of the setup and L. Bonin for their realization. We would like to thank M. Wildi for his advice for the microscopy analyses, C. Moinecourt for her help during Hg analyses and R. Bridou for useful discussions.
References
-
UNEP, Global Mercury Assessment 2013: Sources, Emissions, Releases, and Environmental Transport, 2013 Search PubMed.
-
R. Eisler, Mercury Hazards to Living Organisms, CRC Press Taylor & Francis, Boca Raton, FL, USA, 2006 Search PubMed.
-
J. Dellinger, M. Dellinger and J. S. Yauck, in Mercury in the Environment – Pattern and Process, ed. M. Bank, University of California Press, Los Angeles, CA, USA, 2012, ch. 14, pp. 289–300 Search PubMed.
- C. Cosio, R. Fluck, N. Regier and V. I. Slaveykova, Effects of macrophytes on the fate of mercury in aquatic systems, Environ. Toxicol. Chem., 2014, 33, 1225–1237 CrossRef CAS PubMed.
- P. Dranguet, R. Fluck, N. Regier, C. Cosio, S. Le Faucheur and V. I. Slaveykova, Towards mechanistic understanding of mercury availability and toxicity to aquatic primary producers, Chimia, 2014, 68, 799–805 CrossRef CAS PubMed.
- S. Le Faucheur, P. G. C. Campbell, C. Fortin and V. I. Slaveykova, Interactions between mercury and phytoplankton: speciation, bioavailability, and internal handling, Environ. Toxicol. Chem., 2014, 33, 1211–1224 CrossRef CAS PubMed.
- L. B. Cleckner, C. C. Gilmour, J. P. Hurley and D. P. Krabbenhoft, Mercury methylation in periphyton of the Florida Everglades, Limnol. Oceanogr., 1999, 44, 1815–1825 CrossRef CAS.
- S. Gentes, M. Monperrus, A. Legeay, R. Maury-Brachet, S. Davail, J. M. Andre and R. Guyoneaud, Incidence of invasive macrophytes on methylmercury budget in temperate lakes: central role of bacterial periphytic communities, Environ. Pollut., 2013, 172, 116–123 CrossRef CAS PubMed.
- W. R. Hill, A. J. Stewart and G. E. Napolitano, Mercury speciation and bioaccumulation in lotic primary producers and primary consumers, Can. J. Fish. Aquat. Sci., 1996, 53, 812–819 CrossRef CAS.
- S. Zizek, M. Horvat, D. Gibicar, V. Fajon and M. J. Toman, Bioaccumulation of mercury in benthic communities of a river ecosystem affected by mercury mining, Sci. Total Environ., 2007, 377, 407–415 CrossRef CAS PubMed.
- A. H. Bell and B. C. Scudder, Mercury accumulation in periphyton of eight river ecosystems, J. Am. Water Resour. Assoc., 2007, 43, 957–968 CrossRef CAS.
- S. Zizek, R. Milacic, N. Kovac, R. Jacimovic, M. J. Toman and M. Horvat, Periphyton as a bioindicator of mercury pollution in a temperate torrential river ecosystem, Chemosphere, 2011, 85, 883–891 CrossRef CAS PubMed.
- F. M. M. Morel, A. M. L. Kraepiel and M. Amyot, The chemical cycle and bioaccumulation of mercury, Annu. Rev. Ecol. Syst., 1998, 29, 543–566 CrossRef.
- J. M. Benoit, C. C. Gilmour, R. P. Mason and A. Heyes, Sulfide controls on mercury speciation and bioavailability to methylating bacteria in sediment pore waters, Environ. Sci. Technol., 1999, 33, 951–957 CrossRef CAS.
- R. P. Mason, J. R. Reinfelder and F. M. M. Morel, Uptake, toxicity, and trophic transfer of mercury in a coastal diatom, Environ. Sci. Technol., 1996, 30, 1835–1845 CrossRef CAS.
- H. A. Moye, C. J. Miles, E. J. Phlips, B. Sargent and K. K. Merritt, Kinetics and uptake mechanisms for monomethylmercury between freshwater algae and water, Environ. Sci. Technol., 2002, 36, 3550–3555 CrossRef CAS PubMed.
- M. Leclerc, D. Planas and M. Amyot, Relationship between extracellular low-molecular-weight thiols and mercury species in natural lake periphytic biofilms, Environ. Sci. Technol., 2015, 49, 7709–7716 CrossRef CAS PubMed.
- U. Ndu, R. P. Mason, H. Zhang, S. J. Lin and P. T. Visscher, Effect of inorganic and organic ligands on the bioavailability of methylmercury as determined by using a mer-lux bioreporter, Appl. Environ. Microbiol., 2012, 78, 7276–7282 CrossRef CAS PubMed.
- J. K. Schaefer, S. S. Rocks, W. Zheng, L. Y. Liang, B. H. Gu and F. M. M. Morel, Active transport, substrate specificity, and
methylation of Hg(II) in anaerobic bacteria, Proc. Natl. Acad. Sci. U. S. A., 2011, 108, 8714–8719 CrossRef CAS PubMed.
- A. Szczuka, F. M. M. Morel and J. K. Schaefer, Effect of thiols, zinc, and redox conditions on Hg uptake in Shewanella oneidensis, Environ. Sci. Technol., 2015, 49, 7432–7438 CrossRef CAS PubMed.
- A. M. Graham, G. R. Aiken and C. C. Gilmour, Effect of dissolved organic matter source and character on microbial Hg methylation in Hg-S-DOM solutions, Environ. Sci. Technol., 2013, 47, 5746–5754 CrossRef CAS PubMed.
- T. Zhang, B. Kim, C. Leyard, B. C. Reinsch, G. V. Lowry, M. A. Deshusses and H. Hsu-Kim, Methylation of mercury by bacteria exposed to dissolved, nanoparticulate, and microparticulate mercuric sulfides, Environ. Sci. Technol., 2012, 46, 6950–6958 CrossRef CAS PubMed.
- S. Hamelin, D. Planas and M. Amyot, Spatio-temporal variations in biomass and mercury concentrations of epiphytic biofilms and their host in a large river wetland (Lake St. Pierre, Qc, Canada), Environ. Pollut., 2015, 197, 221–230 CrossRef CAS PubMed.
- D. Acha, H. Hintelmann and J. Yee, Importance of sulfate reducing bacteria in mercury methylation and demethylation in periphyton from Bolivian Amazon region, Chemosphere, 2011, 82, 911–916 CrossRef CAS PubMed.
- R. R. S. Correia, M. R. Miranda and J. R. D. Guimaraes, Mercury methylation and the microbial consortium in periphyton of tropical macrophytes: effect of different inhibitors, Environ. Res., 2012, 112, 86–91 CrossRef CAS PubMed.
- E. J. Fleming, E. E. Mack, P. G. Green and D. C. Nelson, Mercury methylation from unexpected sources: molybdate-inhibited freshwater sediments and an iron-reducing bacterium, Appl. Environ. Microbiol., 2006, 72, 457–464 CrossRef CAS PubMed.
- S. Hamelin, M. Amyot, T. Barkay, Y. P. Wang and D. Planas, Methanogens: principal methylators of mercury in lake periphyton, Environ. Sci. Technol., 2011, 45, 7693–7700 CrossRef CAS PubMed.
- M. Kovac Virsek, B. Hubad and A. Lapanje, Mercury induced community tolerance in microbial biofilms is related to pollution gradients in a long-term polluted river, Aquat. Toxicol., 2013, 144–145, 208–217 CrossRef CAS PubMed.
- D. Kelly, K. Budd and D. D. Lefebvre, Mercury analysis of acid- and alkaline-reduced biological samples: identification of meta-cinnabar as the major biotransformed compound in algae, Appl. Environ. Microbiol., 2006, 72, 361–367 CrossRef CAS PubMed.
- I. Lavoie, M. Lavoie and C. Fortin, A mine of information: benthic algal communities as biomonitors of metal contamination from abandoned tailings, Sci. Total Environ., 2012, 425, 231–241 CrossRef CAS PubMed.
- S. Meylan, R. Behra and L. Sigg, Influence of metal speciation in natural freshwater on bioaccumulation of copper and zinc in periphyton: a microcosm study, Environ. Sci. Technol., 2004, 38, 3104–3111 CrossRef CAS PubMed.
- P. Bradac, E. Navarro, N. Odzak, R. Behra and L. Sigg, Kinetics of cadmium accumulation in periphyton under freshwater conditions, Environ. Toxicol. Chem., 2009, 28, 2108–2116 CrossRef CAS PubMed.
- S. Meylan, R. Behra and L. Sigg, Accumulation of copper and zinc in periphyton in response to dynamic variations of metal speciation in freshwater, Environ. Sci. Technol., 2003, 37, 5204–5212 CrossRef CAS PubMed.
- M. Nordberg, J. H. Duffus and D. M. Templeton, Explanatory dictionary of key terms in toxicology: part II (IUPAC Recommendations 2010), Pure Appl. Chem., 2010, 82, 679–751 CrossRef CAS.
- A. G. Bravo, C. Cosio, D. Amouroux, J. Zopfi, P. A. Chevalley, J. E. Spangenberg, V. G. Ungureanu and J. Dominik, Extremely elevated methyl mercury levels in water, sediment and organisms in a Romanian reservoir affected by release of mercury from a chlor-alkali plant, Water Res., 2014, 49, 391–405 CrossRef CAS PubMed.
- A. G. Bravo, J. L. Loizeau, L. Ancey, V. G. Ungureanu and J. Dominik, Historical record of mercury contamination in sediments from the Babeni Reservoir in the Olt River, Romania, Environ. Sci. Pollut. Res., 2009, 16, 66–75 CrossRef PubMed.
- A. G. Bravo, J. L. Loizeau, S. Bouchet, A. Richard, J. F. Rubin, V. G. Ungureanu, D. Amouroux and J. Dominik, Mercury human exposure through fish consumption in a reservoir contaminated by a chlor-alkali plant: Babeni reservoir (Romania), Environ. Sci. Pollut. Res., 2010, 17, 1422–1432 CrossRef CAS PubMed.
- S. Guedron, S. Devin and D. A. Vignati, Total and methylmercury partitioning between colloids and true solution: from case studies in sediment overlying and porewaters to a generalized model, Environ. Toxicol. Chem., 2016, 35, 330–339 CrossRef CAS PubMed.
-
USEPA, Method 1631, Revision E: Mercury in Water by Oxidation, Purge and Trap, and Cold Vapor Atomic Fluorescence Spectrometry, Washington DC, USA, 2002 Search PubMed.
- E. Tipping, Modelling the interactions of Hg(II) and methylmercury with humic substances using WHAM/Model VI, Appl. Geochem., 2007, 22, 1624–1635 CrossRef CAS.
- K. J. Powell, P. L. Brown, R. H. Byrne, T. Gadja, G. Hefter, S. Sjöberg and H. Wanner, Chemical speciation of environmentally significant heavy metals with inorganic ligands. Part 1: the Hg2+-Cl−, OH−, CO32−, SO42−, and PO43− aqueous systems (IUPAC report), Pure Appl. Chem., 2005, 77, 739–800 CrossRef CAS.
-
R. L. Malcom, in Humic Substances in Soil, Sediment and Water. Geochemistry, Isolation and Characterization, ed. G. R. Aiken, D. M. McKnight, R. L. Wershaw and P. MacCarthy, Wiley-Interscience, New York, 1985, pp. 181–209 Search PubMed.
- S. Le Faucheur, Y. Tremblay, C. Fortin and P. G. C. Campbell, Acidification increases mercury uptake by a freshwater alga, Chlamydomonas reinhardtii, Environ. Chem., 2011, 8, 612–622 CrossRef CAS.
- B. Liu, H. Y. Yan, C. P. Wang, Q. H. Li, S. Guedron, J. E. Spangenberg, X. B. Feng and J. Dominik, Insights into low fish mercury bioaccumulation in a mercury-contaminated reservoir, Guizhou, China, Environ. Pollut., 2012, 160, 109–117 CrossRef CAS PubMed.
-
USEPA, Methylmercury in Water by Distillation, Aqueous Ethylation, Purge and Trap, and Cold Vapor Atomic Fluorescence Spectrometry, Washington, D.C., USA, 1998 Search PubMed.
-
M. Wildi, Maîtrise Universitaire en Sciences de l'Environnement, University of Geneva, 2014 Search PubMed.
- K. Valnes and P. Brandtzaeg, Retardation of immunofluorescence fading during microscopy, J. Histochem. Cytochem., 1985, 33, 755–761 CrossRef CAS PubMed.
-
W. S. Rasband, ImageJ, U. S. National Institutes of Health, Bethesda, Maryland, USA, 1997-2016, http://imagej.nih.gov/ij/ Search PubMed.
- A. Bravo Garcia, J.-L. Loizeau, P. Dranguet, S. Makri, E. Björn, G. Ungureanu, V. Slaveykova and C. Cosio, Persistent Hg contamination and occurrence of Hg methylating transcript (hgcA) downstream of a chlor-alkali plant in the Olt River (Romania), Environ. Sci. Pollut. Res., 2015, 23, 10529–10541 CrossRef PubMed.
-
RCore-Team, A Language and Environment for Statistical Computing, R Foundation for Statistical Computing, Vienna, Austria, 2015, https://www.R-project.org Search PubMed.
- N. E. Selin, Global change and mercury cycling: challenges for implementing a global mercury treaty, Environ. Toxicol. Chem., 2014, 33, 1202–1210 CrossRef CAS PubMed.
- S. M. Ullrich, M. A. Ilyushchenko, I. M. Kamberov and T. W. Tanton, Mercury contamination in the vicinity of a derelict chlor-alkali plant. Part I: sediment and water contamination of Lake Balkyldak and the River Irtysh, Sci. Total Environ., 2007, 381, 1–16 CrossRef CAS PubMed.
- N. S. Bloom, L. M. Moretto and P. Ugo, A comparison of the speciation and fate of mercury in two contaminated coastal marine ecosystems: the Venice Lagoon (Italy) and Lavaca Bay (Texas), Limnol. Oceanogr., 2004, 49, 367–375 CrossRef CAS.
- S. Le Faucheur, D. Vasiliu, I. Catianis, M. Zazu, P. Dranguet, R. Beauvais-Flück, J. L. Loizeau, C. Cosio, C. Ungureanu, V. G. Ungureanu and V. I. Slaveykova, Environmental quality assessment of reservoirs impacted by Hg from chlor-alkali technologies: case study of a recovery, Environ. Sci. Pollut. Res., 2016, 23, 22545–22553 CrossRef PubMed.
- W. Wang and C. T. Driscoll, Patterns of total mercury concentrations in
Onondaga lake, new york, Environ. Sci. Technol., 1995, 29, 2261–2266 CrossRef CAS PubMed.
- T. Barkay, M. Gillman and R. Turner, Effects of dissolved organic carbon and salinity on bioavailability of mercury, Appl. Environ. Microbiol., 1997, 63, 4267–4271 CAS.
- P. R. Gorski, D. E. Armstrong, J. P. Hurley and D. P. Krabbenhoft, Influence of natural dissolved organic carbon on the bioavailability of mercury to a freshwater alga, Environ. Pollut., 2008, 154, 116–123 CrossRef CAS PubMed.
- H. Hsu-Kim, K. H. Kucharzyk, T. Zhang and M. A. Deshusses, Mechanisms regulating mercury bioavailability for methylating microorganisms in the aquatic environment: a critical review, Environ. Sci. Technol., 2013, 47, 2441–2456 CrossRef CAS PubMed.
- K. K. Mueller, S. Lofts, C. Fortin and P. G. C. Campbell, Trace metal speciation predictions in natural aquatic systems: incorporation of dissolved organic matter (DOM) spectroscopic quality, Environ. Chem., 2012, 9, 356–368 CrossRef CAS.
- C. Toader, S. Chitimiea and C. Patroescu, Spreading and degradation of some persistent pesticides in a hydrological regulation reservoir biota, Rev. Roum. Chim., 2000, 45, 197–201 CAS.
- C. M. Santegoeds, T. G. Ferdelman, G. Muyzer and D. de Beer, Structural and functional dynamics of sulfate-reducing populations in bacterial biofilms, Appl. Environ. Microbiol., 1998, 64, 3731–3739 CAS.
- S. Okabe, T. Itoh, H. Satoh and Y. Watanabe, Analyses of spatial distributions of sulfate-reducing bacteria and their activity in aerobic wastewater biofilms, Appl. Environ. Microbiol., 1999, 65, 5107–5116 CAS.
- M. Bahr, B. C. Crump, V. Klepac-Ceraj, A. Teske, M. L. Sogin and J. E. Hobbie, Molecular characterization of sulfate-reducing bacteria in a New England salt marsh, Environ. Microbiol., 2005, 7, 1175–1185 CrossRef CAS PubMed.
- D. E. Holmes, K. P. Nevin, R. A. O'Neil, J. E. Ward, L. A. Adams, T. L. Woodard, H. A. Vrionis and D. R. Lovley, Potential for quantifying expression of the Geobacteraceae citrate synthase gene to assess the activity of Geobacteraceae in the subsurface and on current-harvesting electrodes, Appl. Environ. Microbiol., 2005, 71, 6870–6877 CrossRef CAS PubMed.
- M. Desrosiers, D. Planas and A. Mucci, Mercury methylation in the epilithon of boreal shield aquatic ecosystems, Environ. Sci. Technol., 2006, 40, 1540–1546 CrossRef CAS PubMed.
- E. J. Kerin, C. C. Gilmour, E. Roden, M. T. Suzuki, J. D. Coates and R. P. Mason, Mercury methylation by dissimilatory iron-reducing bacteria, Appl. Environ. Microbiol., 2006, 72, 7919–7921 CrossRef CAS PubMed.
- J. K. Schaefer, R. M. Kronberg, F. M. Morel and U. Skyllberg, Detection of a key Hg methylation gene, hgcA, in wetland soils, Environ. Microbiol. Rep., 2014, 6, 441–447 CrossRef CAS PubMed.
- Y. R. Liu, R. Q. Yu, Y. M. Zheng and J. Z. He, Analysis of the microbial community structure by monitoring an Hg methylation gene (hgcA) in paddy soils along an Hg gradient, Appl. Environ. Microbiol., 2014, 80, 2874–2879 CrossRef PubMed.
- T. Barkay, S. M. Miller and A. O. Summers, Bacterial mercury resistance from atoms to ecosystems, FEMS Microbiol. Rev., 2003, 27, 355–384 CrossRef CAS PubMed.
- L. F. Caslake, S. S. Harris, C. Williams and N. McCreary Waters, Mercury-resistant bacteria associated with macrophytes from a polluted lake, Water, Air, Soil Pollut., 2006, 174, 93–105 CrossRef CAS.
- A. E. Kirkwood, P. Chow-Fraser and G. Mierle, Seasonal mercury levels in phytoplankton and their relationship with algal biomass in two dystrophic shield lakes, Environ. Toxicol. Chem., 1999, 18, 523–532 CrossRef CAS.
- W. R. Hill and I. L. Larsen, Growth dilution of metals in microalgal biofilms, Environ. Sci. Technol., 2005, 39, 1513–1518 CrossRef CAS PubMed.
- E. Peres, M. Coste, F. Ribeyre, M. Ricard and A. Boudou, Effects of methylmercury and inorganic mercury on periphytic diatom communities in freshwater indoor microcosms, J. Appl. Phycol., 1997, 9, 215–227 CrossRef.
Footnote |
† Electronic supplementary information (ESI) available. See DOI: 10.1039/c6em00493h |
|
This journal is © The Royal Society of Chemistry 2017 |
Click here to see how this site uses Cookies. View our privacy policy here.