DOI:
10.1039/C6MH00317F
(Communication)
Mater. Horiz., 2017,
4, 72-82
Ultrafast colorimetric humidity-sensitive polyelectrolyte coating for touchless control†
Received
22nd August 2016
, Accepted 24th October 2016
First published on 24th October 2016
Abstract
Herein we report the visible colorimetric humidity-sensitive properties of the layer-by-layer assembled poly(diallyldimethylammonium) (PDDA)/poly(styrenesulfonate) (PSS) polyelectrolyte coatings and their application for a touchless control system that can be accessed by humidity change. The as-developed touchless control system enables humidity signals, such as those imparted by human breath or a close-proximity fingertip, to switch a light-emitting diode and trigger a computer to implement commands (e.g., making phone calls and playing music). The PDDA/PSS coatings exhibit different colors at different thicknesses, which results from the interference of visible light in the coating. Notably, they can undergo vivid and reversible color changes that span the whole visible spectrum at an ultrafast speed (ca. 35 ms) according to the local humidity change. Exploiting the ability of the coating to modulate light, the output optical signal (i.e., color change) was converted into an electrical signal by irradiating the coating with a beam of monochromatic light and collecting the reflected modulated light using a photodetector. The obtained electrical signal was subsequently processed and used as an input signal for touchless control that is accessible by a humidity signal. The as-developed touchless control system is applicable for the touchless interface of electronic devices, smart switches, automotive, smart buildings, and so forth.
Conceptual insights
The “thin-film interference” effect is widely associated with many natural color-display phenomena and the skin color change of some environmentally sensitive living beings. However, the optical interference-induced color display and its response to the external stimuli of layer-by-layer (LbL) assembled polyelectrolyte thin films were little studied or exploited for practical applications. This communication is the first-ever report on the visible and ultrafast colorimetric humidity-sensitive properties of LbL assembled polyelectrolyte thin films/coatings, poly(diallyldimethylammonium) (PDDA)/poly(styrenesulfonate) (PSS) polyelectrolyte multilayers (PEMs). By combining the light-modulation ability of PDDA/PSS coatings and optical interrogation techniques, this work for the first time illustrates a new form of touchless control that is accessible by humidity change, such as that imparted by human breath or a close-proximity fingertip. This work not only opens up new applications of LbL-assembled PEMs, but also provides a new concept of exploiting the optical interference-induced sensing properties of a material to develop advanced touchless control systems. Such control systems are imperative for applications on electronic devices with a strong focus on disabled-user friendliness, hygiene and/or novelty.
|
1. Introduction
With the rapid development of electronic devices that cover almost all aspects of our daily lives, the development of touchless control systems is becoming ever more important. Compared to conventional hand-touch-based control systems, touchless control promises improved convenience, especially for users with impaired movement or occupied hands (e.g., driving), and hygiene-focused activities (e.g., performing surgery). Here, for the first time, we report a new form of touchless control based on an ultrafast colorimetric humidity-sensitive material, derived from a layer-by-layer (LbL) assembled polyelectrolyte multilayer (PEM) coating. The polyelectrolyte coating can undergo an ultrafast color change according to the change in local humidity, which results from the modulation of light through interference by its humidity-dependent thickness variation. The light-modulation ability of the polyelectrolyte coating enables us to convert its output optical signal (i.e., color change) into an input electrical signal of a touchless control system by photonics techniques. The as-developed touchless control system enables human breath or a close-proximity fingertip to switch a light-emitting diode (LED) and trigger a computer to implement commands, such as making phone calls, playing music, and so forth.
Since its discovery in 1991, the LbL assembled PEM thin film/coating1–3 of alternating polycation and polyanion has been extensively studied and a wide variety of applications thereof have been exploited.4–9 The applications of PEM films/coatings include anti-reflection coatings,10 superhydrophobic and slippery surfaces,11–14 drug delivery systems,15 anti-fouling surfaces,16 solar-energy conversion,17 separation membranes,18 electronic gas sensors,19,20 and so forth. In principle, a thin film gives rise to an interference phenomenon of light waves that are reflected by its upper and lower boundaries, which is known as “thin-film interference”.21,22 If the thickness of the film is in the nanoscale level (e.g., typically tens to hundreds of nanometers), the interference of light in the visible spectrum occurs and produces a highly visible color display.21–24 This mechanism is associated with many natural phenomena, such as the beautiful color of soap bubbles and the oil films on water.25 Many living beings also utilize this mechanism to display color, such as peacocks and butterflies.26 Some of them, such as squids and chameleons, are even capable of changing their colors by transforming the structure of the thin layers that affect the interference of light.27,28 However, the “thin-film interference” effect of PEM thin films/coatings has been far from sufficiently studied,29–31 and their applications in this aspect have been little exploited.
In this communication, we show that the LbL assembled poly(diallyldimethylammonium) (PDDA)/poly(styrenesulfonate) (PSS) multilayer coatings fabricated on polished Si wafers exhibit different colors at different thicknesses, which results from the interference of visible light reflected by the air–coating and coating–substrate interfaces (Scheme 1a). Intriguingly, we also found that the PDDA/PSS coatings can undergo ultrafast (ca. 35 ms of response time), vivid and reversible color changes according to the local humidity change, which provides a visible humidity-detection platform. This is due to the predominant thickness variation resulting from swelling or shrinking of the coating (Scheme 1b). This work is the first-ever report on the visually colorimetric humidity-sensitive properties of LbL assembled PEM coatings, though the humidity-sensitive properties of PEMs have been applied to resistive humidity sensors, actuators and fiber-optic humidity sensors.32–35 More significantly, the response time (ca. 35 ms) of the as-fabricated coatings is amongst the fastest of the previously reported humidity sensors,36,37 despite the preparation of our coatings being much simpler and of lower-cost. Note that the current state-of-the-art humidity sensors typically exhibit response times in the order of hundreds of milliseconds to several seconds (i.e., one or two orders of magnitude slower response compared to that reported in this work).38–43
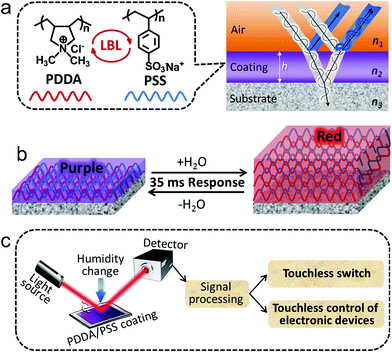 |
| Scheme 1 (a) Schematic sketch of the molecular structures of PDDA and PSS (left) and the schematic of the interference of the same-wavelength light across the PDDA/PSS coating (right). (b) Schematic illustration of the swelling and shrinking of the PDDA/PSS coating according to the local humidity change, which results in the color change of the coating. (c) Schematic illustration of the touchless control system based on the PDDA/PSS coating and photonics techniques. | |
The output optical signal of the PDDA/PSS coatings as a function of relative humidity (RH) can easily be converted into a digital readout (i.e., electrical signals) by using a photodetector. The touchless control system accessible with humidity changes was then achieved in a simple way. A beam of monochromatic light was irradiated on the coating and the reflected modulated light was detected using a photodetector. The color change of the coating was converted into an electrical signal that provided the input signal for touchless control (Scheme 1c). We show that the humidity change imparted by human breath or a close-proximity fingertip can be used as the trigger to implement the touchless control, such as switching on an LED and communicating with a computer. The ultrafast response of the coating towards humidity changes ensures near-zero latency of the commands implementation. Human breath typically has a RH of up to 100%, and the RH around human fingers can reach 100% due to the constant secretion of water through sweat, while the ambient RH is normally in the range of 30–70%. Therefore, compared to the currently available touchless control technologies (see Part 1.1 in the ESI†), humidity-detection-based touchless control promises the highest ratio of human signal to ambient noise in most situations, which ensures better accuracy and security. This new touchless control system can be readily used by many physically impaired users. Furthermore, the simple design of this touchless control system and the easy preparation of the PDDA/PSS PEM coatings significantly facilitate the easy take-up of this new technology.
2. Experimental
2.1. Materials
PDDA (Mw 100
000–200
000, 20 wt% in H2O), PSS (Mw 70
000), 3-aminopropyl triethoxysilane and anhydrous toluene were purchased from Sigma-Aldrich. H2O2 (30 wt%) and H2SO4 (98%) were purchased from Chem-Supply, Australia. All the chemicals were used as received without further purification. Silicon wafers were purchased from Si-Mat Silicon Materials, Germany. Deionized water (>18.2 MΩ cm−1) was used in all the experiments.
Prior to use, the silicon wafers were cleaned and oxidized via immersion in piranha solution (1
:
3 (v/v) mixture of 30% H2O2 and 98% H2SO4), followed by heating until no bubbles were released. After this treatment, the surface of the Si substrates was oxidized and fully covered with the silanol groups. Thus, the as-obtained Si substrates will be denoted as Si–OH substrates. Note that piranha solution reacts violently with organic materials and should be handled carefully.
2.2. Preparation of the PDDA/PSS coatings on Si–OH substrates
(PDDA/PSS)N coatings were prepared on the Si–OH substrates by alternating immersion of the substrates into the aqueous solutions of PDDA and PSS for 20 min until the desired layer number was reached. Each immersion step was followed by thorough rinsing with water and drying under a N2 flow. PDDA and PSS aqueous solutions contain PDDA and PSS with concentrations of 1 mg mL−1, and NaCl with a concentration of 1.0 M.
2.3. Preparation of the PSS/PDDA coatings on amino-group-modified Si substrates
The amino-group-modified Si substrates (denoted as Si–NH2 substrates) were prepared according to a previously reported protocol.32 (PSS/PDDA)N coatings were prepared on the Si–NH2 substrates via the same procedure as that used for the fabrication of (PDDA/PSS)N coatings on the Si–OH substrates, except the first deposition layer was PSS.
2.4. Characterization of the humidity-sensitive properties of the PDDA/PSS coatings
The reflectance spectra of the PDDA/PSS coatings were measured using the Filmetrics instrument (Thin-Film Analyzer, F20), which can also provide the thickness data of the coatings. The thickness data acquired using the Filmetrics instrument at ambient RH were further verified using a stylus profilometer (Bruker Dextak XTL, see Fig. S2, ESI†). The refractive indices of the coatings were deduced based on the thickness data using eqn (S17) in the ESI.† To take the photographs or measure the reflectance spectra and thickness of the coatings at different RHs, the samples were put in sealed quartz cuvettes which contained silica gel, pure water or various kinds of saturated salt solutions that could produce a specific RH (see Table S1 in the ESI†). The cross-section SEM images of the (PDDA/PSS)20 coatings at varying RHs were obtained on a FEI Quanta 450 SEM at the ESEM mode. The reported thickness for a specific RH, shown in Fig. 2e is the average value obtained from the same sample, out of three SEM images, each with 30 equidistant positions measured. Therefore, the average value with standard-deviation error bars was derived from 90 data points. Optical microscopy images were obtained on a Nikon DS-Ri2 microscope. Atomic force microscopy (AFM) imaging was performed using a MultiMode 8 AFM from Bruker in the ScanAsyst mode under ambient conditions using Si cantilevers.
2.5. Measurement of the temporal characteristics of the PDDA/PSS coatings
The experimental setup is shown in Fig. 3a. A narrow-linewidth laser (i.e. Thorlabs HNLS008R-EC) centered at around 633 nm wavelength was used to probe the coating at 55° from its normal. The reflected beam was measured using a photodetector (i.e. Thorlabs PDB450C) and displayed on an oscilloscope (i.e. Rigol DS6104) as shown in Fig. 3a. The coating was exposed to different levels of relative humidity by blowing nitrogen through a 5 mm diameter plastic tube positioned at 3 mm from the surface of the coating. At the initial stage, the nitrogen was controlled by mixing dry and wet (i.e. successively passing through 50 °C and 25 °C water) nitrogen through two flow meters, which yields a total air flow of 5 litres per minute. At the intermediate stage, the ratio and thus relative humidity were calibrated using a commercial humidity sensor (i.e. RisePro, 1% resolution). At the final stage, the insertion of a three-way tube switch before the output ensured that the system pressure was kept constant despite switching between the ambient and the desired relative humidities.
2.6. Touchless control devices based on the PDDA/PSS coatings
The experimental setup is shown in Fig. 4c and 6. The output BNC cable from the photodetector was modified such that the live and ground wires feed analog signals into the ports of a digital acquisition module (i.e. Dataq DI-149). The output USB cable of this module then delivers digital signals to the computer. Labview software was used to implement the touchless control of the computer by human breath or a close-proximity fingertip. To execute the command, such as opening a web browser, the windows console-style script was used, for example, “http://cmd/c start http://www.abc.net.au”.
3. Results and discussion
3.1. Colorimetric PDDA/PSS polyelectrolyte coating
The PDDA/PSS PEM coatings were prepared via alternate deposition of PDDA and PSS on piranha-solution-treated polished Si wafers in the presence of 1.0 M NaCl.14,44,45 The resultant coatings are denoted as (PDDA/PSS)N, where N represents the number of deposited bilayers. Half and whole bilayers denote the coatings with PDDA and PSS as the outmost layer, respectively. Although denoted as a “multilayer”, there is negligible refractive-index variation across the coating due to the polyelectrolyte diffusion,46,47 and thus the whole PDDA/PSS coating can be treated as an optically homogeneous layer. This is evident through the absence of Bragg reflection peaks in the reflectance spectra of the coatings, as further discussed later in this work. As shown in Scheme 1a, when the rays of light strike the coating from a specific angle, a fraction of the light is reflected at the air–coating interface and the rest is refracted into the coating. A second reflection of the refracted light at the coating–substrate interface leads to interference when the two groups of rays overlap at the air–coating interface. As a result, the total light reflection by the coating will be strengthened at certain wavelengths but weakened at others, resulting in an observable color if the interference dominates in the visible spectrum.21–24 The detailed theoretical explanation is given in the ESI† (see Part 1.2 that includes Fig. S1–S5). Si was chosen as the substrate because it exhibits a good optical reflectivity and blocks background light (see Part 1.2 that includes Fig. S1–S5, and Part 1.3 in the ESI†). Fig. 1a and Fig. S6 (ESI†) show the photographs of the (PDDA/PSS)N coatings with N varying from 7.5 to 15.0, taken at a viewing angle perpendicular to the coating surface. Depending on the number of bilayers varying from 7.5 to 10.5, the PDDA/PSS coatings exhibit different colors that are highly distinguishable under ambient conditions with a RH of ca. 45%, ranging from shades of blue, yellow, red and green (Fig. 1a). As shown in Fig. 1b and Fig. S7 (ESI†), the reflectance spectra of the coatings with N varying from 7.5 to 15.0 follow a sinusoidal pattern; the period of which increases with longer wavelengths and/or fewer bilayers (Fig. S8, ESI†). It can be seen that the visible color of the PDDA/PSS coatings becomes indistinguishable by the naked eye beyond 14.0 bilayers (Fig. S6, ESI†) with a thickness of 1298 nm. This is due to the multitude of interference fringes in the visible wavelength domain that no longer hosts a dominant wavelength/color, as well as the higher attenuation of light through the thicker coating that reduces the interference visibility. Note that the reflectance spectra do not show Bragg reflection peaks that are caused by multilayer (i.e., layers with different refractive indices) interference,31 which suggests that the PDDA/PSS coating can be regarded as an optically homogeneous thin film. The reflectance spectra show that the coatings selectively reflect light at particular wavelengths that correspond to the color of the coatings (Fig. 1a). The position of the nominated reflection peak (i.e., based on the sole reflection peak of the (PDDA/PSS)8.0 sample within the visible spectrum) proportionally red shifts with increasing N, while the coating thickness also linearly increases with N (Fig. 1c). The period (Δλ) of the sinusoidal reflectance spectrum can be theoretically predicted by the following equation (see Part 1.4 in the ESI†): |  | (1) |
This reveals that the period is inversely proportional to the refractive index of the coating (n2) and thickness (h), while it is proportional to the wavelength of light (λ). The latter two are the dominant factors in determining the period, as n2 of the coatings varies relatively little compared to h and λ for the coatings with different N (Fig. S9, ESI†). These trends are evident in Fig. 1b and Fig. S7 (ESI†). The relationship between the coating thickness (h) and the nominated peak position (λP) can be expressed by the following equation (see Part 1.5 in the ESI†): |  | (2) |
where m is an integer number representing the peak index. This suggests that the position of the nominated peak is proportional to the coating thickness based on the fact that n2 (e.g., 1.35) of the coatings varies relatively little, which explains the results shown in Fig. 1c and Fig. S10 (ESI†).
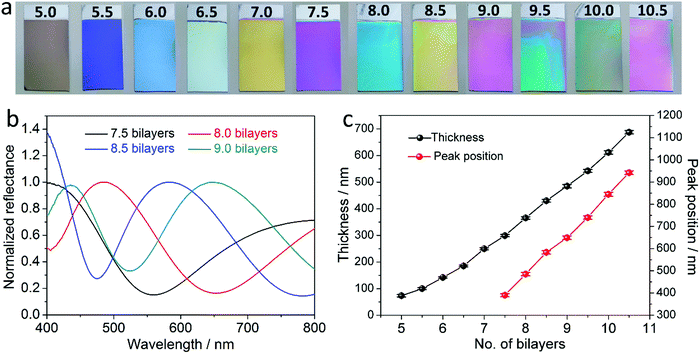 |
| Fig. 1 (a) Photographs of the (PDDA/PSS)N coatings with N varying from 5.0 to 10.5. Half and whole bilayers denote the coatings with PDDA and PSS as the outmost layer, respectively. (b) Reflectance spectra across the visible wavelengths for the (PDDA/PSS)N coatings with N of 7.5, 8.0, 8.5 and 9.0. (c) Thickness and peak position (derived from the reflectance spectra) of the (PDDA/PSS)N coatings as a function of the bilayer number (N). The PDDA/PSS coatings were fabricated on polished Si wafers in the presence of 1 M NaCl. All the measurements were conducted under ambient conditions with a RH of ca. 45%. The thickness of the coatings was provided by the Filmetrics instrument, derived from mathematical fitting based on the measured reflectance spectra. The data shown in (c) are the average values with standard-deviation error bars derived from the measurements of 3 baths of samples. | |
3.2. Colorimetric humidity-sensing properties of the PDDA/PSS coating
Fig. 2a and Fig. S11 (ESI†) show the photographs of the (PDDA/PSS)N coatings with N of 8.0, 8.5 and 7.5, which were exposed to a gradually increasing RH varying from 0% to 100%. The coatings exhibit a highly distinguishable color transition with increasing RH, which spans purple, blue, green, yellow and near-red, suggesting that the PDDA/PSS coatings can be directly utilized as visible humidity-indicator strips. Note that the best viewing angles for the PDDA/PSS coatings used as humidity-indicator strips are within 33° relative to the normal incidence (i.e., perpendicular to the coating surface) (Fig. S12, ESI†). The corresponding reflectance spectra of the (PDDA/PSS)N coatings measured at different RHs are shown in Fig. 2b and Fig. S13 (ESI†). Fig. 2c indicates that the position of the nominated peaks red shifts with increasing RH. More specifically, the RH-dependent peak-position shift for the (PDDA/PSS)8.0 or (PDDA/PSS)8.5 coating from a RH of 0% to 100% is 227 nm or 290 nm, respectively. It is worth noting that the peak-position shift of the PDDA/PSS coatings is at the highest level (e.g., >200 nm)42,48,49 compared to other reported colorimetric humidity-sensitive coatings that are normally derived from periodically structured photonic crystals and exhibit peak-position shifts below 150 nm38,50–54 for a RH ranging from 0% to 100%. Therefore, the as-developed PDDA/PSS coatings exhibit a relatively high sensitivity to humidity. Importantly, the color change and spectra shift of the coatings are completely reversible by restoring the initial RH, as evidenced by the test for 10 cycles (Fig. S14, ESI†). Fig. 2d shows that the thickness of the PDDA/PSS coatings increases with increasing RH, indicating the swelling of the coatings in a humid environment. Specifically, the thicknesses of the (PDDA/PSS)8.0 and (PDDA/PSS)8.5 coatings at 100% RH are respectively 482.6 nm and 623.0 nm, which are 1.63 and 1.65 times of those (i.e., 295.2 nm and 378.3 nm, respectively) measured at 0% RH, respectively. By comparing Fig. 2c and d, the peak position of the PDDA/PSS coatings follows a near-linear dependence on their thickness (Fig. S15, ESI†). This phenomenon can be well explained by eqn (2), where the refractive index change of the coatings at different RHs is relatively small (Fig. S16, ESI†) compared to the thickness change, which makes the thickness change the dominant contributor to the peak-position shift. To physically observe the thickness variation of a PDDA/PSS coating at various RHs, the cross-section of the (PDDA/PSS)20 coating was measured using an environmental scanning electron microscopy (ESEM) under different RHs. It can be seen that the thickness of the (PDDA/PSS)20 coating exhibits a 1.56-fold increase (i.e., 2.26 to 3.53 μm) in thickness when the RH is increased from 0% to 100% (Fig. 2e and Fig. S17, ESI†). This is in agreement with the results generated by the mathematical fitting (provided by the Filmetrics instrument and verified using the stylus profilometer; see Fig. S2 and S3, ESI†) for the (PDDA/PSS)8.0 and (PDDA/PSS)8.5 coatings. Note that the PDDA/PSS coatings are extremely stable in exposed environments with varying RH. The samples after being cyclically exposed to 100% and 0% RH 2000 times have still well kept their colorimetric humidity-sensing properties, though the reflectance spectra of the resulting sample exhibit slight drifts at a specific RH compared to those of the original one (e.g., blue shift by 14 nm at 0% RH and red shift by 16 nm at 100% RH). This indicates that the resulting sample exhibits an enlarged reflectance peak-position shift (i.e., increased humidity sensitivity) from 0% RH to 100% RH (Fig. S18, ESI†). This phenomenon can be attributed to the structural reorganization of the film during successive adsorption (i.e., swelling) and desorption (i.e., shrinking) of water, which increases the retractability of the entire film. Additionally, the humidity-sensing performance of the PDDA/PSS coating shows negligible change after the sample was incubated in a very high humidity environment (e.g., 97% RH) for a long time (e.g., 48 h) (Fig. S19, ESI†). It is also worth noting that the displayed color of the PDDA/PSS coating is insensitive to the pressure applied on top of it (Fig. S20, ESI†).
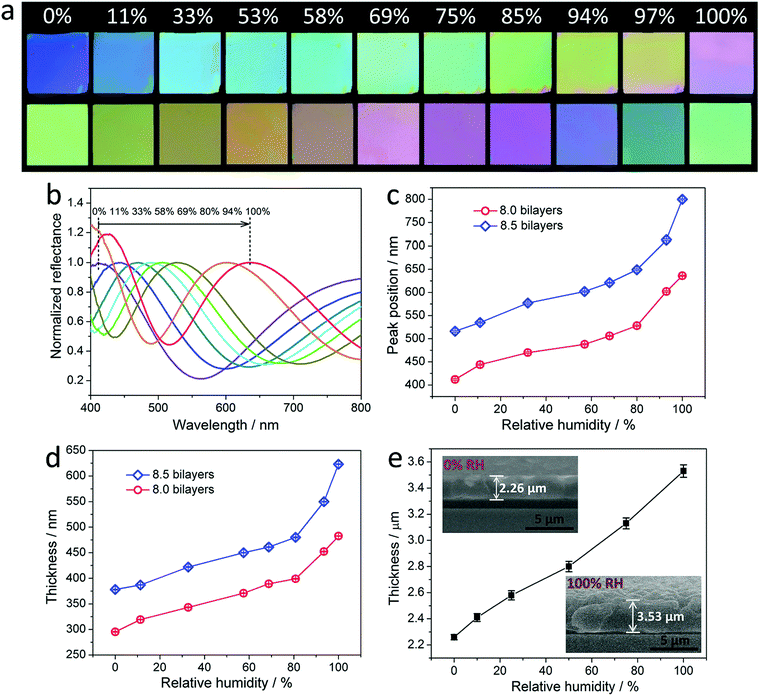 |
| Fig. 2 (a) Photographs of the (PDDA/PSS)8.0 (upper panel) and (PDDA/PSS)8.5 (lower panel) coatings at different RHs gradually varying from 0% to 100%. (b) Reflectance spectra of the (PDDA/PSS)8.0 coating across the visible wavelengths at different RHs gradually varying from 0% to 100%. (c and d) Peak position (c, derived from the reflectance spectra) and thickness (d) of the (PDDA/PSS)8.0 and (PDDA/PSS)8.5 coatings as a function of the RH to which the coatings were exposed. The thickness of the coatings was provided by the Filmetrics instrument, derived from mathematical fitting based on the measured reflectance spectra. (e) Thickness of the (PDDA/PSS)20 coating as a function of the RH to which the coating is exposed, which was measured using ESEM. Insets: Cross-section ESEM images of the (PDDA/PSS)20 coating measured at a RH of 0% and 100%. The data shown in (c and d) are the average values with standard-deviation error bars derived from 5 measurements on the same sample. | |
In addition, the effect of surface properties of the Si substrates on the colorimetric humidity-sensing properties of the deposited PDDA/PSS coatings was also investigated. Instead of directly depositing the PDDA/PSS multilayers onto the piranha-solution-treated Si substrates (Si–OH substrates), the amino-group-modified Si substrates that were prepared using the 3-aminopropyl triethoxysilane coupling agent were also used as substrates (Si–NH2 substrates) to deposit the PEMs with PSS as the first deposition layer.32 The substrate surface modification and the PEM deposition were verified using X-ray photoelectron spectroscopy (Fig. S21, ESI†). It was found that, under ambient conditions (ca. 45% RH), the (PSS/PDDA)9.0 coating deposited on the Si–NH2 substrate exhibits quite a similar reflectance peak position (i.e., 485 nm) and thickness (i.e., 360 nm) with those (i.e., 487 nm and 366 nm, respectively) of the (PDDA/PSS)8.0 coating prepared on the Si–OH substrate (Fig. S22, ESI†). Intriguingly, the nominated reflectance peak-position of the Si–NH2/(PSS/PDDA)9.0 sample shifts as much as 305 nm (i.e., from 420 nm to 725 nm) from a RH of 0% to 100%, which is much larger compared to that (i.e., 227 nm) of the Si–OH/(PDDA/PSS)8.0 sample (Fig. S22, ESI†). The reflectance peak-position shift of the Si–NH2/(PSS/PDDA)9.0 sample corresponds to a coating thickness increase of 1.86 times from 300 nm at 0% RH to 553 nm at 100% RH, which is much more significant compared to that (i.e., 1.63 times) of the Si–OH/(PDDA/PSS)8.0 sample. Accordingly, the PEMs deposited on the Si–NH2 substrate exhibit much greater humidity-induced swellability in the thickness direction compared to those deposited on the Si–OH substrate. It is hypothesized that the covalently anchored aminopropyl groups on the Si–NH2 substrates work as a spacer between the PEMs and the substrate, which considerably suppresses the restriction effect of the substrate on the swelling of the deposited films. Detailed investigation on the mechanism of substrate surface properties on the humidity-dependent swelling of PDDA/PSS PEMs is still underway and will be reported in our future work. Similar to that of the PDDA/PSS coatings deposited on the Si–OH substrates, the Si–NH2/(PSS/PDDA)9.0 coating also exhibits a slight reflectance peak-position drift at a specific RH (e.g., blue shift by 11 nm at 0% RH and red shift by 12 nm at 100% RH) after the sample was cyclically exposed to 100% and 0% RH 2000 times (Fig. S23, ESI†). This result further verifies that the reflectance spectra drift of the PEMs at a specific RH after successive shrinking and swelling results from the structural reorganization of the film, rather than the properties of the substrate. The PDDA/PSS coatings described henceforth all refer to those fabricated on the Si–OH substrates.
3.3. Temporal characteristics of the PDDA/PSS coating
The ability of the PDDA/PSS coatings to modulate light through “thin-film interference” enables them to change the wavelength-resolved power of the light after reflection. The shift of the reflectance spectra of the PDDA/PSS coatings with varying RH certifies the power change of the reflected light at any specific wavelength. Therefore, the temporal characteristics of the coating for humidity sensing can be determined by simply monitoring the power change of the reflected light at a particular wavelength. To do so, a monochromatic laser (i.e., narrow linewidth exhibiting 30 cm coherence length) centered at around a wavelength of 633 nm was used to probe the coating at 55° from its normal (see the Experimental section). The reflected beam was measured with a photodetector and analyzed using an oscilloscope (Fig. 3a). The coating was instantly exposed to different RHs by blowing a nitrogen flow that is a mixture of dry and wet nitrogen with varied ratios. The RH of the nitrogen was calibrated using a commercial humidity sensor. The photodetector converted the optical signal into an electrical one, and the measured voltage as a function of the RH for the (PDDA/PSS)8.0 coating is shown in Fig. 3b. The voltage increases when the RH increases from 0%, reaches its maximum (Vmax) at the near-ambient RH (50%) before dropping when the RH further increases, and reaches its minimum (Vmin) at 100% RH. Fig. 3c shows the temporal behavior of the measured signal when the RH exposed to the (PDDA/PSS)8.0 coating was cyclically switched between ambient RH (ca. 45%) and 90%. The slight fluctuation of the voltage signal at the particular RH results from the flocculation of the actual RH to which the sample was exposed. As shown in Fig. 3d, the coating exhibits ultrafast response and recovery times, which were measured to be 35 ms and 950 ms respectively based on the 10% and 90% levels of the maximum signal variation. These durations are similar for the detection of different RHs (Fig. S24, ESI†). Accordingly, by combining photonics and the PDDA/PSS coating, an ultrafast humidity sensor with a quantitative readout can be developed. Note that our (PDDA/PSS)N coatings show superior colorimetric humidity-sensing properties in terms of both faster response and larger RH-dependent wavelength shifts compared to the typical colorimetric humidity-sensitive materials reported previously.50–54 We hypothesize that the ultrafast response of the PDDA/PSS coating is due to its nanoscale thin-film characteristics (e.g., ca. 365.6 nm for the (PDDA/PSS)8.0 coating under ambient conditions), the hydrophilic nature of both the PDDA and PSS components, and the strong electrostatic interactions between the PDDA and PSS chains in the coating. The first two factors significantly enhance the diffusion of moisture through the coating. The third factor facilitates localized swelling or shrinking of the coating to rapidly spread out like springs (Scheme 1b). The larger wavelength shifts are consistent with the water absorption properties of PDDA and PSS components as they are both hydrophilic polyelectrolytes, and thus induce a more significant swelling or shrinking of the coating under different humidities. It is also worth mentioning that we found some other LbL assembled PEMs also exhibiting colorimetric humidity-sensitive properties, while their performance differs in terms of the color display and response/recovery speed. The systematic research in this aspect is still underway.
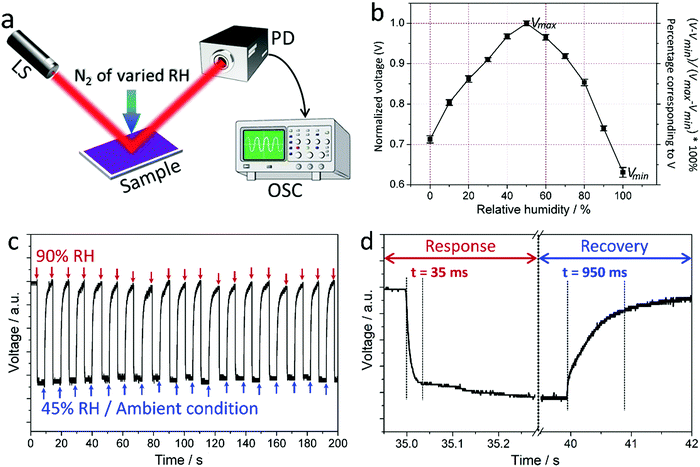 |
| Fig. 3 (a) Schematic of the setup for converting the coating color change into electrical signals. LS: laser source, PD: photodetector, and OSC: oscilloscope. (b) The normalized voltage (V, left y-axis) of the electrical signals generated from the modulation of light by the (PDDA/PSS)8.0 coating as a function of the RH to which the sample was exposed. The data shown are the average values with standard-deviation error bars derived from 20 measurements on the same sample. The right y-axis represents a percentage (x%) corresponding to V, x% = (V − Vmin)/(Vmax − Vmin) × 100%. (c) Temporal response of the electrical signal generated from the modulation of light by the (PDDA/PSS)8.0 coating while the RH exposed to the sample was switched between 45% and 90%. (d) Enlarged view of (c) in the time range of 34.9–42 s, which shows the response and recovery times of the (PDDA/PSS)8.0 coating in the course of RH switching from ca. 45% to 90% and then recovering to ca. 45%. | |
3.4. Touchless control based on the PDDA/PSS coating
The electrical signal generated by the modulation of monochromatic light from the colorimetric PDDA/PSS coating under different humidities can be used as an input signal to realize touchless control. This is demonstrated here by using this signal to switch on an LED and to control a computer using human breath or a close-proximity fingertip, because they can naturally produce a much higher RH than the ambient RH to trigger the coating for a physical reaction. As shown in Fig. 4a, Fig. S25a and Movie S1 (ESI†), the application of human breath guided by a glass tube onto the (PDDA/PSS)8.0 and (PDDA/PSS)8.5 coatings induced rapid color changes from blue and yellow into near-red and green, respectively, without any noticeable delay. Fig. 4b, Fig. S25b and Movie S2 (ESI†) show the distance-dependent color change when a finger was placed next to one side of the coating (without contact). By comparing the color of the coatings shown in Fig. 4a and b with those shown in Fig. 2, one can conclude that both the human breath and finger can generate RH as high as 100%. It was also found in both cases that the coating could easily recover from the dew point (i.e., when the RH reaches or exceeds 100%), which suggests good reversibility of the coating. Note that the reaction of the (PDDA/PSS)8.0 coating towards finger moisture is as fast as 40 ms at a sample–fingertip distance of 5 mm (Fig. S26, ESI†). The experimental setup for the touchless control of an LED is shown in Fig. 4c. The signal from the photodetector not only feeds into the oscilloscope but also to a comparator circuit powering an LED. The comparator circuit offers the function to manually tune the voltage threshold (Vref) for the input signal, below which the circuit will stop delivering the current needed to keep the LED on. The principle of how the comparator circuit works is described in detail in Part 1.6 in the ESI.†Vref is defined by following equation: | Vref = Vmin + x%(Vmax − Vmin) | (3) |
where Vmin and Vmax are defined in Fig. 3b, and thus Vref can be varied by changing the variable x. Hence, according to Fig. 3b, upon exposure of the coating to a higher RH (e.g., by a human breath or close-proximity fingertip) than that of the ambient surroundings, the voltage feed into the comparator circuit from the photodetector will drop and cause the LED to dim55 and switch off (Fig. 4d). The switch directionality can be changed by simply swapping the comparator terminals, though dimming the LED offers better visibility. Note that it is also possible to use a broadband light source such as a white-light-emitting diode or a halogen lamp combined with a wavelength filter to achieve the same effect as a monochromatic laser source in the touchless control system.
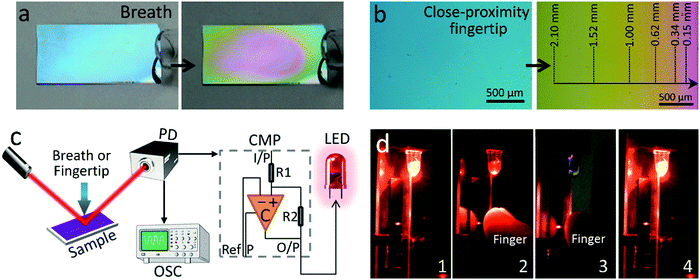 |
| Fig. 4 (a) Photographs of the (PDDA/PSS)8.0 coating before and after a human breath is exhaled onto its surface, the breath was guided by a glass tube. (b) Microscopy image of the (PDDA/PSS)8.0 coating before and after a finger was placed next to one side of the sample (without contact), with the distance from the localized position on the sample to the finger labelled on the image. (c) Schematic of the setup for coating interrogation and touchless control of switching on an LED. LS: laser source, PD: photodetector, OSC: oscilloscope, CMP: comparator, I/P: input voltage, Ref: reference voltage, P: power rail voltage, and O/P: output voltage. (d) A series of photographs demonstrating that a close-proximity fingertip on the sample causes the LED to dim (d2) and turn off (d3). d1 shows the LED in an “on” state under ambient conditions and d4 shows that the LED becomes “on” from an “off” state (shown in d3) after the proximate finger was retracted. Note that the finger does not obstruct the beam path of the angled-incidence light. | |
Fig. 5a and b show the electrical signal measured from the modulated light by the coating and the voltage measured across the LED, during the course of the cyclic application of a human breath or close-proximity of a fingertip onto the coating, respectively. The voltage threshold (Vref) of the comparator circuit was set by setting x% to be 90% in eqn (3). It can be observed that the LED voltage modulation follows the voltage modulation generated by the coating under human breath or a close-proximity fingertip. The LED switch triggered by human breath and a close-proximity fingertip is demonstrated in Movies S3 and S4 (ESI†). By adjusting the voltage threshold (Vref) of the comparator circuit, the critical humidity that induces the LED to reach certain states can be tailored. Fig. 5c depicts the Vref-dependence of the critical RH that causes the LED to begin to dim and turn off, indicating that a lower Vref requires a relatively high RH to implement the command. This is in good agreement with the data plotted in Fig. 3b. For practical applications, for example in the automotive industry, and in homes and hospitals, the threshold should be set based on the requirement and the ambient RH. Fig. 5d depicts the Vref-dependence of the critical finger-coating distance that causes the LED to switch. The trend suggests that a lower threshold needs a closer-proximity fingertip, because the RH is higher, closer to the finger. Note that the farthest finger distance that can trigger the control is approximately 8 mm.
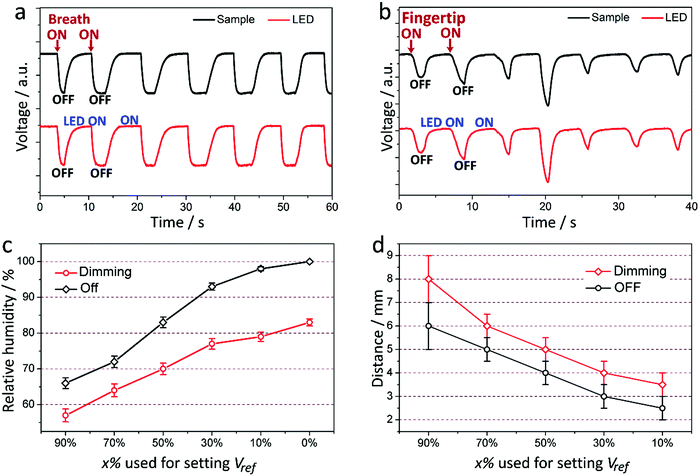 |
| Fig. 5 (a and b) Temporal response of the electrical signal acquainted from the coating and LED in the course of intermittently applying human breaths (a) and close-proximity fingertips (b) onto the sample. (c and d) The RH (c) and finger-sample distance (d) that are required to make the LED begin to dim and turn off as a function of the percentage (x%) used to set Vref based on eqn (3). The data shown in (c) and (d) are the average values with standard-deviation error bars derived from 5 measurements on the same sample. | |
Taking the touchless control to a more advanced stage, the electrical signal from the photodetector was fed into a digital acquisition module that converted analogue signals to digital ones and then sent them to a computer (Fig. 6). Based on this setup, it is possible to implement commands by using human breath or close-proximity fingertips toward the coating. We can give different commands by counting the number of signals generated by the user input within a certain timeframe (see the detailed description in Fig. S27, ESI†). As demonstrated in Movies S5–S8 (ESI†), human breath can invoke the computer to make a phone call, play music, open the internet explorer and open the calendar. We found that the access range for the touchless control by human breath with or without the tubing guidance (internal diameter of 4 mm) can be up to 5 m and 25 cm, respectively. However, the access range can be influenced by the depth of breath, the air flow of the ambient conditions, the diameter of the tubing, and the distance between the sensor head and outlet of the tubing. Note that our coatings are able to produce a large variation of electrical signals as shown in Fig. 3b, resulting from large RH-dependent reflectance peak-position shifts under different RHs. Therefore, for the more advanced application in practice, complex commands can be sent by users by modulating their interaction with the coating in terms of strength and time-varying patterns and using an advanced software interface.
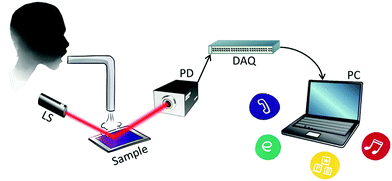 |
| Fig. 6 Schematic illustration of the touchless control of a computer to implement commands by human breath. LS: laser source, PD: photodetector, DAQ: digital acquisition, and PC: personal computer. Labview software was used to implement the touchless control of a computer via human breath. The functionality of the Labview software is essentially a comparator, a counter and a timer working in union. To execute a command such as opening a web browser, the windows console-style script was used, for example, “cmd/c start http://www.abc.net.au”. | |
In principle, electronically fabricated humidity sensors based on integrated circuits that sense humidity by altering their impedance or capacitance are also eligible for touchless control, if their sensitivity is high enough and their response time is fast enough. However, their fabrication always requires a complex multiple-step sequence involving lithographic and/or complex chemical processing.56 In addition, integrated circuits suffer from drawbacks such that the entire device would become difficult to repair or even useless if a critical component fails, and they are susceptible to electromagnetic interference and temperature drifts. Therefore, our touchless control utilizing photonics is much simpler because all the required components can be purchased off the shelf, and the resulting device is easily maintained due to the modular design.
4. Conclusions
In summary, we have reported the colorimetric humidity-sensing properties of the LbL assembled PDDA/PSS coating that varies its color according to the local humidity with an extremely short response time of ca. 35 ms. This phenomenon results from the swelling/shrinking of the coating at different humidities and the interference of visible light in the coating. The as-prepared PDDA/PSS coating can be directly used as visible humidity detector strips that do not require electrical components. In addition, the PDDA/PSS coating exhibits an excellent stability at exposed environments for humidity sensing. Significantly, a new form of touchless control based on the PDDA/PSS coating was developed by using a simple photonics system, which can be operated using a humidity signal, such as that imparted by human breath or a close-proximity fingertip. Considering that the PDDA/PSS coating can be easily fabricated at low cost, and the optical interrogation of the touchless control system can be assembled using off-the-shelf components, this newly developed technology holds excellent commercial viability. Furthermore, the modular design of the entire system makes repairs cost-effective and all of these components can be downscaled to realize compact devices with dimensions of a few centimeters or less (Fig. S28, ESI†). There is significant potential that this technology can be an important complementary touchless control technology of those currently available, to be used in the automotive industry, smart buildings, and for the touchless interface of next-generation electronic devices, which can even be readily used by disabled users.
Acknowledgements
T. M. acknowledges the support of an ARC Georgina Sweet Laureate Fellowship. X. L. acknowledges the support of a Research Connections Grant (RC44943) funded by the Department of Industry, Australian Government, and ITEK Ventures Pty Ltd. X. L. also thanks the financial support from the Future Industries Institute, University of South Australia.
Notes and references
- G. Decher and J. D. Hong, Macromol. Symp., 1991, 46, 321–327 CrossRef CAS.
- G. Decher, Science, 1997, 277, 1232–1237 CrossRef CAS.
- F. Caruso, R. A. Caruso and H. Möhwald, Science, 1998, 282, 1111–1114 CrossRef CAS PubMed.
-
Multilayer Thin Films, ed. G. Decher and J. Schlenoff, Wiley-VCH, Weinheim, 2nd edn, 2012 Search PubMed.
- Z. Tang, Y. Wang, P. Podsiadlo and N. A. Kotov, Adv. Mater., 2006, 18, 3203–3224 CrossRef CAS.
- X. Zhang, H. Chen and H. Zhang, Chem. Commun., 2007, 1395–1405 RSC.
- P. T. Hammond, Mater. Today, 2012, 15, 197–206 CrossRef.
- Y. Li, X. Wang and J. Sun, Chem. Soc. Rev., 2012, 41, 5998–6009 RSC.
- J. J. Richardson, M. Björnmalm and F. Caruso, Science, 2015, 348, 411 CrossRef CAS PubMed.
- J. Hiller, J. Mendelsohn and M. F. Rubner, Nat. Mater., 2002, 1, 59–63 CrossRef CAS PubMed.
- L. Zhai, F. C. Cebeci, R. E. Cohen and M. F. Rubner, Nano Lett., 2004, 4, 1349–1353 CrossRef CAS.
- U. Manna and D. M. Lynn, Adv. Mater., 2015, 27, 3007–3012 CrossRef CAS PubMed.
- U. Manna, N. Raman, M. A. Welsh, Y. M. Zayas-Gonzalez, H. E. Blackwell, S. P. Palecek and D. M. Lynn, Adv. Funct. Mater., 2016, 26, 3599–3611 CrossRef CAS.
- L. Yu, G. Y. Chen, H. Xu and X. Liu, ACS Nano, 2016, 10, 1076–1085 CrossRef CAS PubMed.
- B. B. Hsu, M.-H. Park, S. R. Hagerman and P. T. Hammond, Proc. Natl. Acad. Sci. U. S. A., 2014, 111, 12175–12180 CrossRef CAS PubMed.
- A. Vaterrodt, B. Thallinger, K. Daumann, D. Koch, G. M. Guebitz and M. Ulbricht, Langmuir, 2016, 32, 1347–1359 CrossRef CAS PubMed.
- H. Tokuhisa and P. T. Hammond, Adv. Funct. Mater., 2003, 13, 831–839 CrossRef CAS.
- K. L. Cho, A. J. Hill, F. Caruso and S. E. Kentish, Adv. Mater., 2015, 27, 2791–2796 CrossRef CAS PubMed.
- S. Bai, C. Sun, H. Yan, X. Sun, H. Zhang, L. Luo, X. Lei, P. Wan and X. Chen, Small, 2015, 11, 5807–5813 CrossRef CAS PubMed.
- Q. Ji, I. Honma, S.-M. Paek, M. Akada, J. P. Hill, A. Vinu and K. Ariga, Angew. Chem., Int. Ed., 2010, 49, 9737–9739 CrossRef CAS PubMed.
-
Z. Knittl, Optics of Thin Films: An Optical Multilayer Theory, John Wiley, 1976 Search PubMed.
-
Handbook of Optics, ed. M. Bass, Mcgraw-Hill Professional, New York, 3rd edn, 2010 Search PubMed.
- A. V. Yakovlev, V. A. Milichko, V. V. Vinogradov and A. V. Vinogradov, ACS Nano, 2016, 10, 3078–3086 CrossRef CAS PubMed.
- M. Xiao, Y. Li, M. C. Allen, D. D. Deheyn, X. Yue, J. Zhao, N. C. Gianneschi, M. D. Shawkey and A. Dhinojwala, ACS Nano, 2015, 9, 5454–5460 CrossRef CAS PubMed.
- I. Blei, J. Chem. Educ., 1981, 58, A179 CrossRef.
- D. G. Stavenga, Materials Today: Proceedings, 2014, 1S, 109–121 CrossRef.
- L. M. Mäthger, E. J. Denton, N. J. Marshall and R. T. Hanlon, J. R. Soc., Interface, 2009, 6, S149–S163 CrossRef PubMed.
- J. Teyssier, S. V. Saenko, D. van der Marel and M. C. Milinkovitch, Nat. Commun., 2015, 6, 6368 CrossRef CAS PubMed.
- E. D. Cranston and D. G. Gray, Biomacromolecules, 2006, 7, 2522–2530 CrossRef CAS PubMed.
- L. Wagberg, G. Decher, M. Norgren, T. Lindstrom, M. Ankerfors and K. Axnas, Langmuir, 2008, 24, 784–795 CrossRef PubMed.
- T. C. Wang, R. E. Cohen and M. F. Rubner, Adv. Mater., 2002, 14, 1534–1537 CrossRef CAS.
- C.-W. Lee, J.-G. Kim and M.-S. Gong, Macromol. Res., 2005, 13, 265–272 CrossRef CAS.
- Y. Ma, Y. Zhang, B. Wu, W. Sun, Z. Li and J. Sun, Angew. Chem., Int. Ed., 2011, 50, 6254–6257 CrossRef CAS PubMed.
- C. S. Lao, Q. Kuang, Z. L. Wang, M.-C. Park and Y. Deng, Appl. Phys. Lett., 2007, 90, 262107 CrossRef.
- F. J. Arregui, K. L. Cooper, Y. Liu and I. R. Matías, IEICE Trans. Electron., 2000, E83–C, 360–365 Search PubMed.
- S. Borini, R. White, D. Wei, M. Astley, S. Haque, E. Spigone, N. Harris, J. Kivioja and T. Ryhänen, ACS Nano, 2013, 7, 11166–11173 CrossRef CAS PubMed.
- U. Mogera, A. A. Sagade, S. J. George and G. U. Kulkarni, Sci. Rep., 2014, 4, 1–9 Search PubMed.
- M. M. Hawkeye and M. J. Brett, Adv. Funct. Mater., 2011, 21, 3652–3658 CrossRef CAS.
- A. Buvailoa, Y. Xing, J. Hines and E. Borguet, Sens. Actuators, B, 2011, 156, 444–449 CrossRef.
- H. Chi, Y. J. Liu, F. Wang and C. He, ACS Appl. Mater. Interfaces, 2015, 7, 19882–19886 CAS.
- X. Wang, Z. Xiong, Z. Liu and T. Zhang, Adv. Mater., 2015, 27, 1370–1375 CrossRef CAS PubMed.
- K. Szendrei, P. Ganter, O. Sànchez-Sobrado, R. Eger, A. Kuhn and B. V. Lotsch, Adv. Mater., 2015, 27, 6341–6348 CrossRef CAS PubMed.
- E. Kim, S. Y. Kim, G. Jo, S. Kim and M. J. Park, ACS Appl. Mater. Interfaces, 2012, 4, 5179–5187 CAS.
- R. A. Ghostine, M. Z. Markarian and J. B. Schlenoff, J. Am. Chem. Soc., 2013, 135, 7636–7646 CrossRef CAS PubMed.
- R. Zhang, K. Köhler, O. Kreft, A. Skirtach, H. Möhwald and G. Sukhorukov, Soft Matter, 2010, 6, 4742–4747 RSC.
- R. A. Ghostine, R. M. Jisr, A. Lehaf and J. B. Schlenoff, Langmuir, 2013, 29, 11742–11750 CrossRef CAS PubMed.
- O. Soltwedel, P. Nestler, H.-G. Neumann, M. Paßvogel, R. Köhler and C. A. Helm, Macromolecules, 2012, 45, 7995–8004 CrossRef CAS.
- E. Tian, J. Wang, Y. Zheng, Y. Song, L. Jiang and D. Zhu, J. Mater. Chem., 2008, 18, 1116–1122 RSC.
- R. Xuan, Q. Wu, Y. Yin and J. Ge, J. Mater. Chem., 2011, 21, 3672–3676 RSC.
- Z. Wang, J. Zhang, J. Xie, C. Li, Y. Li, S. Liang, Z. Tian, T. Wang, H. Zhang, H. Li, W. Xu and B. Yang, Adv. Funct. Mater., 2010, 20, 3784–3790 CrossRef CAS.
- R. A. Barry and P. Wiltzius, Langmuir, 2006, 22, 1369–1374 CrossRef CAS PubMed.
- M. C. Fuertes, S. Colodrero, G. Lozano, A. R. Gonzalez-Elipe, D. Grosso, C. Boissiere, C. Sanchez, G. J. de A. A. Soler-Illia and H. Miguez, J. Phys. Chem. C, 2008, 112, 3157–3163 CAS.
- I. Pavlichenko, A. T. Exner, M. Guehl, P. Lugli, G. Scarpa and B. V. Lotsch, J. Phys. Chem. C, 2012, 116, 298–305 CAS.
- C. Fenzl, T. Hirsch and O. S. Wolfbeis, Angew. Chem., Int. Ed., 2014, 53, 3318–3335 CrossRef CAS PubMed.
- Note: Unlike a mechanical switch that instantaneously changes its output, a comparator gradually transitions its output state in response to its input signals, which induces the LED gradually but not instantly switched off.
- J. Feng, L. Peng, C. Wu, X. Sun, S. Hu, C. Lin, J. Dai, J. Yang and Y. Xie, Adv. Mater., 2012, 24, 1969–1974 CrossRef CAS PubMed.
Footnote |
† Electronic supplementary information (ESI) available: Supplementary notes, schemes, figures and tables. See DOI: 10.1039/c6mh00317f |
|
This journal is © The Royal Society of Chemistry 2017 |
Click here to see how this site uses Cookies. View our privacy policy here.