DOI:
10.1039/C6QM00097E
(Research Article)
Mater. Chem. Front., 2017,
1, 100-110
Spiro[fluorene-9,9′-xanthene]-based hole transporting materials for efficient perovskite solar cells with enhanced stability†
Received
21st June 2016
, Accepted 22nd July 2016
First published on 16th August 2016
Abstract
Four spiro[fluorene-9,9′-xanthene] (SFX)-based hole transporting materials (HTMs) functionalized with four-armed arylamine moieties located at different positions are designed and synthesized. These compounds exhibit highest occupied molecular orbital (HOMO) energy levels of −4.9 to −5.1 eV and a hole mobility of 2.2 to 15 × 10−5 cm2 V−1 s−1 after doping. Perovskite solar cells (PSCs) based on a methylammonium lead iodide (MAPbI3) active layer using one of these HTMs (mp-SFX-2PA) exhibit power conversion efficiencies (PCEs) of up to 16.8%, which is higher than that of the control devices based on benchmark spiro-OMeTAD under the same conditions (15.5%). PSCs based on mp-SFX-2PA exhibit better stability (retain 90% of their initial PCEs after 2000 h storage in an ambient atmosphere) than the control devices based on spiro-OMeTAD (retain only 28% of their initial PCEs). mp-SFX-2PA based devices employing a mixed formamidinium lead iodide (FAPbI3)/methylammonium lead bromine (MAPbBr3) perovskite layer exhibit an improved PCE of 17.7%. The effects of arylamines and their location positions on device performance are discussed.
1. Introduction
In the last few years, organic–inorganic hybrid perovskite solar cells (PSCs) have rapidly developed and have received great attention as a low-cost alternative candidate for third generation solar cells. As their photoactive layer, organic-lead trihalide perovskite materials (e.g. methylammonium lead halides MAPbX3, where X = Cl−, I−, Br−) possess a unique combination of intrinsic properties, such as an appropriate direct band gap, strong and broad absorption, a low exciton binding energy, ambipolar charge transport, and a long carrier diffusion length and lifetime.1–3 Since the first attempt to employ this class of materials as sensitizers in liquid-based dye-sensitized solar cells (DSSCs) by Miyasaka and coworkers in 2009,4 tremendous progress has been achieved in the field of PSCs, along with the development of processing techniques, device architectures and novel materials.5–13 One of the best performing device structures of PSCs consists of a mesoporous TiO2 layer, which is infiltrated with a perovskite active layer and capped with hole transporting materials (HTMs).14–17 HTMs extract photogenerated holes from the perovskite active layer and transport to the back contact metal electrode and play an important role in minimizing the recombination loss. For the further improvement of the photovoltaic performance and stability of PSCs, it is important to develop high-performance HTMs.18
So far, the most widely used and efficient HTM employed for PSCs is 2,2′,7,7′-tetrakis(N,N-di-p-methoxyphenylamine)-9,9′-spirobifluorene (spiro-OMeTAD), which yields a maximum power conversion efficiency (PCE) over 20%.19 However, spiro-OMeTAD is quite expensive due to difficulty in its synthesis and purification, which hinders its further commercial application. Moreover, its relatively low intrinsic hole mobility makes it necessary to be doped with lithium bis(trifluoromethylsulfonyl) imide (Li-TFSI) and 4-tert-butylpyridine (tBP). Since these additives are hydrophilic and cause instability in devices, the exploration of novel HTMs with low cost, good stability and high efficiency is urgently needed. Generally, HTMs used in PSCs include inorganic, small molecular and polymeric materials. Inorganic HTMs (i.e. NiO, CuI and CuSCN) have attracted attention mainly because of their high hole mobility and good chemical stability.20–23 Small molecular HTMs offer potential advantages, such as a defined molecular structure, a definite molecular weight, easy purification and good batch-to-batch reproducibility. Various small molecular HTMs, such as linear,24–28 star-shaped29–34 and four-armed structures35–39 have been developed and exhibit excellent efficiencies up to 18%. On the other hand, PSCs employing polymeric HTMs also exhibit a comparable photovoltaic performance.15,40–48 However, batch-to-batch variation in their molecular weights may limit their commercial application.
Spiro[fluorene-9,9′-xanthene] (SFX) derivatives have received increasing attention as next-generation spirofluorenes in the last ten years due to their competitive one-pot route.49 Due to its cruciform-shaped structure and facile synthesis, SFX provides a platform for the molecular design of multi-dimensional organic semiconductors via various C–X cross-coupling reactions, such as the Suzuki coupling reaction and the Ullmann reaction. SFX derivatives are used as wide-bandgap blue fluorescent materials, phosphorescent host and guest materials, and hole-transporting materials for organic light-emitting diodes (OLEDs).50–52 When we prepared this manuscript, Sun and coworkers reported a SFX-based HTM for application in PSCs.53
Here, we report the synthesis and photovoltaic application of four SFX-based HTMs functionalized with electron-donating bis(4-methoxyphenyl)aniline or bis(4-methoxyphenyl)amine groups (Fig. 1), and investigate the relationship between the molecular structure and the photovoltaic properties. PSCs with the structure of FTO/TiO2/MAPbI3/mp-SFX-2PA/Au exhibit a maximum PCE of 16.8%, which is slightly superior to a PSC based on spiro-OMeTAD (15.5%) under the same conditions. Moreover, mp-SFX-2PA-based devices show a much better stability than the spiro-OMeTAD-based devices. Replacing MAPbI3 with mixed formamidinium lead iodide (FAPbI3)/methylammonium lead bromine (MAPbBr3) further enhances the PCE of the mp-SFX-2PA-based devices to 17.7%.
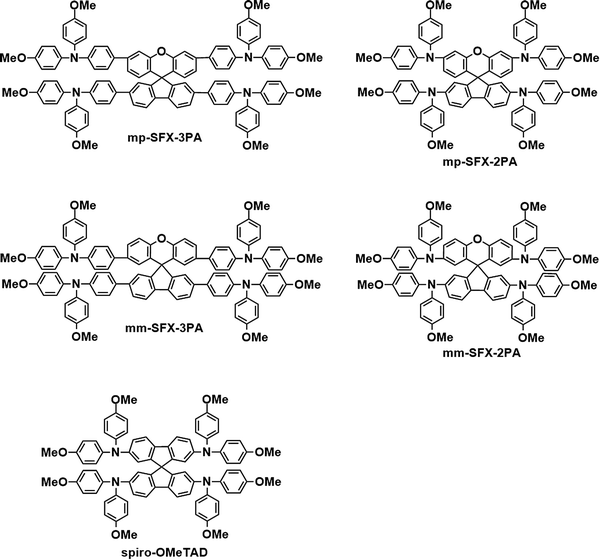 |
| Fig. 1 Chemical structures of HTMs. | |
2. Results and discussion
2.1 Molecular design and synthesis
In order to gain insights into the geometrical configuration and electronic distribution of these four compounds and spiro-OMeTAD, density functional theory (DFT) calculations were carried out using the Gaussian 09 program54 at the B3LYP/6-31G* level.55–57 The optimized molecular geometries, electron distribution of the highest occupied molecular orbital (HOMO), HOMO−1 and the lowest unoccupied molecular orbital (LUMO) of these molecules are shown in Fig. S1 (ESI†), and the calculated values of energy levels are summarized in Table S1 (ESI†). Similar to spiro-OMeTAD, these four SFX-based compounds exhibit highly twisted configurations, which is beneficial for isotropic charge carrier transport. For SFX-based compounds, the HOMO and HOMO−1 distribute on the upside or downside of the molecule, while for spiro-OMeTAD, the HOMO and HOMO−1 are delocalized over the whole molecule.
The SFX tetrabromides SFX-1 and SFX-2 were prepared according to our previous literature.49 Compounds mp-SFX-3PA and mm-SFX-3PA were synthesized through Suzuki coupling reactions between SFX-1 or SFX-2 and triphenylamine boron ester (3PA-B) using Pd(PPh3)4 as the catalyst (Scheme S1, ESI†). Compounds mp-SFX-2PA and mm-SFX-2PA were synthesized through Ullmann reactions between SFX-1 or SFX-2 and bis(4-methoxyphenyl)amine using Pd2dba3 and P(t-Bu)3 as the catalysts. These four compounds were characterized by matrix-assisted laser desorption/ionization-time-of flight mass spectrometry (MALDI-TOF MS), 1H NMR, 13C NMR, and elemental analysis. These four compounds exhibit good thermal stability with decomposition temperature (Td) above 250 °C in a nitrogen atmosphere (Fig. S2, ESI,†Table 1).
Table 1 Optical and thermal properties of different HTMs
HTM |
λ
max (nm) |
ε (104 M−1 cm−1) |
E
g
(eV) |
T
d (°C) |
Solution |
Film |
Estimated from the onset of normalized UV-vis spectra in thin film with the equation Eg = 1240/λonset.
|
mp-SFX-3PA |
352 |
358 |
9.1 |
2.83 |
437 |
mm-SFX-3PA |
352 |
358 |
8.5 |
2.81 |
420 |
mp-SFX-2PA |
304 |
306 |
7.1 |
2.94 |
418 |
mm-SFX-2PA |
306 |
306 |
7.6 |
2.93 |
261 |
spiro-OMeTAD |
386 |
392 |
6.0 |
2.92 |
381 |
2.2 Optical, electrochemical and charge transporting properties
Normalized UV-vis absorption spectra of these four compounds and spiro-OMeTAD in thin film and dichloromethane (DCM) solution are shown in Fig. 2a and Fig. S3 (ESI†), respectively. These four compounds in solution exhibit two absorption bands around 300 and 350 nm with a molar extinction coefficient (ε) of 7–9 × 104 M−1 cm−1 (Table 1), which is similar to spiro-OMeTAD. mp-SFX-3PA and mm-SFX-3PA exhibit absorption maxima at 352 nm, while mp-SFX-2PA and mm-SFX-2PA exhibit absorption maxima at 304–306 nm. Compared with mp-SFX-2PA and mm-SFX-2PA, mp-SFX-3PA and mm-SFX-3PA exhibit a red shift of 46–48 nm of the absorption maxima, which is attributed to the increase in the conjugation length of the SFX-3PA molecules. These compounds exhibit an absorption edge of <450 nm (optical bandgap >2.8 eV), which is beneficial for the perovskite active layer to absorb photons in the visible range.
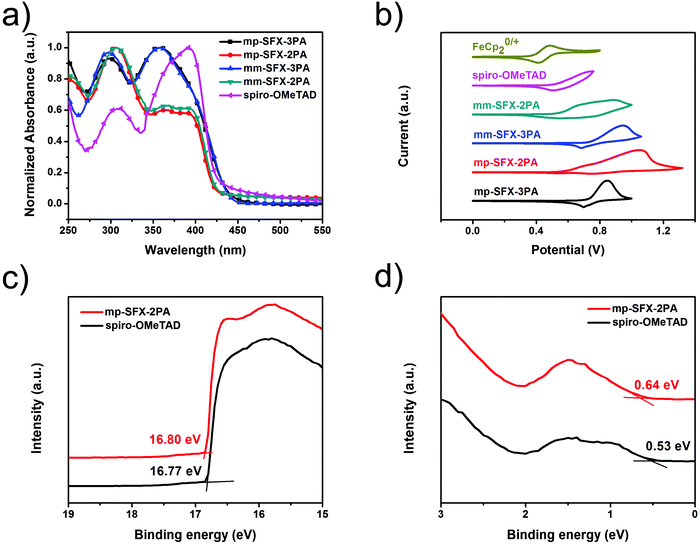 |
| Fig. 2 (a) Normalized UV-vis absorption spectra of different HTMs in thin film. (b) CV diagrams of HTMs. UPS spectra in the (c) cutoff energy region and (d) the onset energy region of mp-SFX-2PA and spiro-OMeTAD films. | |
Cyclic voltammetry (CV) measurements were carried out to estimate the energy levels of these four compounds and to compare with spiro-OMeTAD (Fig. 2b). The onset oxidation potentials versus Fc/Fc+ (0.45 V vs. Ag/AgCl) of mp-SFX-3PA, mm-SFX-3PA, mp-SFX-2PA and mm-SFX-2PA are 0.28, 0.28, 0.12 and 0.09 V, respectively. The HOMO energy levels of mp-SFX-3PA and mm-SFX-3PA are estimated to be −5.08 eV, lower than those of mp-SFX-2PA and mm-SFX-2PA (−4.92 to −4.89 eV) assuming the absolute energy level of Fc/Fc+ to be 4.8 eV below vacuum (Table 2). The LUMO energy levels were calculated from the optical bandgap and the corresponding HOMO energy levels. The LUMOs of mp-SFX-3PA and mm-SFX-3PA are −2.25 to −2.27 eV, lower than those of mp-SFX-2PA and mm-SFX-2PA (−1.97 to −1.98 eV). The trend in energy levels estimated from CV measurements coincides well with the trend from DFT calculation. These four compounds have slightly deeper HOMO and LUMO energy levels compared with spiro-OMeTAD.
Table 2 Energy levels and hole mobilities of HTMs
HTM |
E
ox
(V) |
HOMOb (eV) |
LUMOc (eV) |
μ
h
(cm2 V−1 s−1) |
μ
h
(cm2 V−1 s−1) |
Onset oxidation potential versus Fc/Fc+.
Estimated from the Eox assuming the absolute energy level of Fc/Fc+ to be 4.8 eV below vacuum.
Calculated with the equation ELUMO = EHOMO − Eg.
Pristine state.
Doped state.
|
mp-SFX-3PA |
0.28 |
−5.08 |
−2.25 |
4.0 × 10−6 |
3.3 × 10−5 |
mm-SFX-3PA |
0.28 |
−5.08 |
−2.27 |
5.3 × 10−6 |
2.2 × 10−5 |
mp-SFX-2PA |
0.12 |
−4.92 |
−1.98 |
3.0 × 10−5 |
1.5 × 10−4 |
mm-SFX-2PA |
0.09 |
−4.89 |
−1.97 |
8.7 × 10−6 |
9.9 × 10−5 |
spiro-OMeTAD |
0.03 |
−4.83 |
−1.91 |
4.5 × 10−6 |
2.0 × 10−5 |
The energy levels of mp-SFX-2PA and spiro-OMeTAD films were also measured using ultraviolet photoelectron spectroscopy (UPS) (Fig. 2c and d). The HOMO energy levels of mp-SFX-2PA and spiro-OMeTAD are −5.06 and −4.98 eV, respectively, calculated from the equation EHOMO = −[21.22 − (Ecutoff − Eonset)]. The LUMO energy levels of mp-SFX-2PA and spiro-OMeTAD are −2.12 and −2.00 eV, respectively, calculated from EHOMO and the optical bandgap. mp-SFX-2PA exhibits slightly deeper HOMO and LUMO energy levels relative to spiro-OMeTAD, and the difference in the energy levels between these two HTMs measured by UPS resembles that from CV.
Considering that charge carrier transport in thin film solar cells occurred along the out-of-plane direction, the hole mobilities of the pristine and doped states of these new HTMs were measured using the space charge limited current (SCLC) method, employing hole-only devices with the configuration of ITO/PEDOT:PSS/HTM/Au (Fig. S4, ESI†). The average hole mobility of mp-SFX-2PA in the pristine state is 3 × 10−5 cm2 V−1 s−1, higher than those of mp-SFX-3PA, mm-SFX-3PA and mm-SFX-2PA (4.0–8.7 × 10−6 cm2 V−1 s−1) (Table 2). After doping with Li-TFSI and tBP, the average values of the hole mobility for these four HTMs are improved by one order of magnitude relative to their pristine states; in particular the hole mobility of mp-SFX-2PA is improved to 1.5 × 10−4 cm2 V−1 s−1. The hole mobilities of spiro-OMeTAD in pristine and doped states are 4.5 × 10−6 and 2 × 10−5 cm2 V−1 s−1, respectively, which are lower than those of mp-SFX-2PA.
2.3 Perovskite solar cells
MAPbI3 based conventional mesoporous PSCs with FTO/TiO2/MAPbI3/HTM/Au configuration were fabricated using these HTMs. The total thickness of the devices is around 660 nm, consisting of a 30 nm compact TiO2 layer, a 400 nm mesoporous TiO2/MAPbI3 overlayer, a 150 nm HTM layer and an 80 nm Au counter electrode (Fig. 3a). Fig. 3b shows an energy level diagram of the PSC, estimated by UPS. The energy levels of TiO2 and MAPbI3 are taken from previous literature.5 The energy levels of mp-SFX-2PA match with the valance band of MAPbI3.
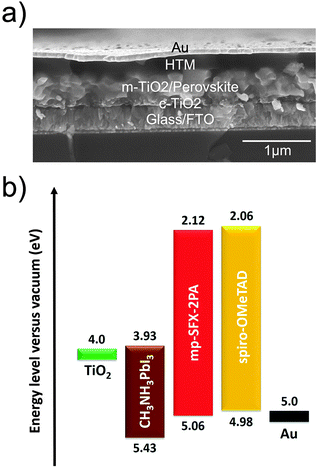 |
| Fig. 3 (a) Cross-sectional SEM image of a representative PSC with the conventional mesoporous structure of FTO/TiO2/MAPbI3/HTM/Au. (b) Energy level diagram of the PSCs, estimated by UPS. | |
The effect of mp-SFX-2PA thickness on the performance of MAPbI3 based PSCs is shown in Fig. S5 and Table S2 (ESI†). The optimum thickness of the doped mp-SFX-2PA layer is 100 nm. Fig. 4a shows the J–V curves of MAPbI3 based PSCs using these HTMs with additives of Li-TFSI and tBP, measured under AM 1.5G illumination, 100 mW cm−2. Unless stated otherwise, the reverse scan condition is employed for all J–V characterization. Both electron-donating groups and substitution positions affect the photovoltaic performance of PSCs (Table 3). After the optimization of the additive concentrations, the mp-SFX-2PA based devices exhibit a maximum PCE of 16.77%, with a JSC of 21.17 mA cm−2, a VOC of 1023 mV and a FF of 77.44%. Changing the position of two diphenylamine moieties from 3′,6′ to 2′,7′ on the SFX core leads to a decreased photovoltaic performance. The mm-SFX-2PA based devices exhibit a maximum PCE of 14.1%, with a JSC of 19.07 mA cm−2, a VOC of 970 mV and a FF of 76.23% under the same fabrication conditions. On the other hand, replacing four bis(4-methoxyphenyl)amine arms with bis(4-methoxyphenyl)aniline reduces the PCEs. The devices based on mp-SFX-3PA and mm-SFX-3PA shows a maximum PCE of 14.05% and 13.25%, respectively.
Table 3 Photovoltaic parameters of the devices based on doped HTMs
HTM |
J
SC (mA cm−2) |
Calculated JSC (mA cm−2) |
V
OC (mV) |
FF (%) |
PCE (%) |
Average |
Best |
(FAPbI3)0.85(MAPbBr3)0.15 based PSCs.
|
mp-SFX-3PA |
18.94 |
18.14 |
1001 |
74.11 |
13.46 |
14.05 |
mp-SFX-2PA |
21.17 |
19.57 |
1023 |
77.44 |
15.59 |
16.77 |
mm-SFX-3PA |
18.02 |
17.07 |
1012 |
72.65 |
12.56 |
13.25 |
mm-SFX-2PA |
19.07 |
18.31 |
970.0 |
76.23 |
13.43 |
14.10 |
spiro-OMeTAD |
20.58 |
19.45 |
1012 |
74.18 |
14.98 |
15.45 |
mp-SFX-2PAa |
20.87 |
20.21 |
1083 |
78.31 |
17.21 |
17.70 |
spiro-OMeTADa |
20.82 |
19.94 |
1091 |
77.66 |
17.12 |
17.64 |
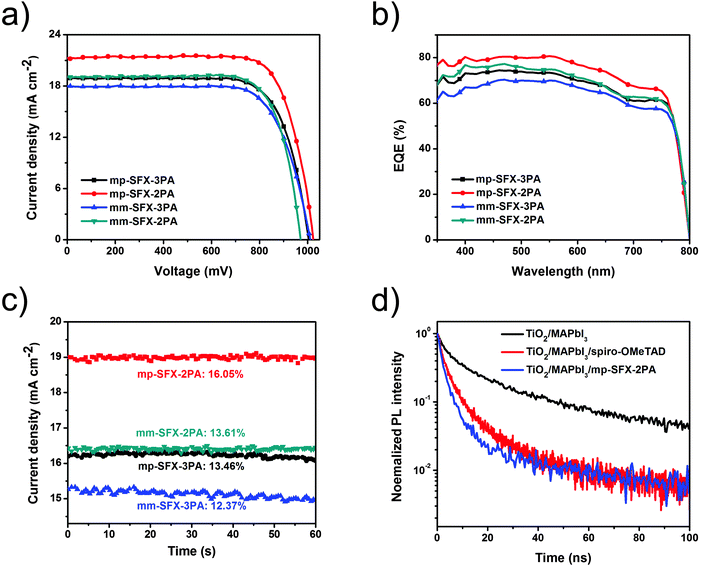 |
| Fig. 4 (a) J–V curves of best performing MAPbI3 based PSCs employing these HTMs with dopants, measured under AM 1.5G illumination, 100 mW cm−2. (b) EQE spectra of the corresponding devices. (c) Steady-state current density and power output measured at the maximum power point. (d) Time-resolved PL decay transient spectra. | |
The control devices based on benchmark spiro-OMeTAD exhibit a maximum PCE of 15.45%, a JSC of 20.58 mA cm−2, a VOC of 1012 mV and a FF of 74.18% under the same conditions (Fig. S6, ESI†). The slightly higher PCE of the devices based on mp-SFX-2PA compared with that of the devices based on spiro-OMeTAD is attributed to a slightly improved JSC, VOC and FF. The higher hole mobility of mp-SFX-2PA leads to an improved JSC and FF, and the slightly deeper HOMO energy level of mp-SFX-2PA leads to a slightly higher VOC.
The external quantum efficiency (EQE) spectra of PSCs are shown in Fig. 4b. The devices based on mp-SFX-2PA exhibit the highest photon-to-current response with a maximum EQE of 80.7% at 550 nm. In order to evaluate the accuracy of the measured photovoltaic performance, JSC values are calculated from the integration of the EQE spectra. The calculated JSC values have a 4.0–7.6% mismatch with those measured under an AM 1.5G solar simulator. As shown in Fig. 4c, the devices based on these four HTMs exhibit a steady-state current density output under the maximum power point, with stabilized efficiencies of 12.37–16.05%, which are close to the values measured from J–V characterization. Efficient PSCs based on these four HTMs could be fabricated reproducibly (Fig. S7, ESI†). The hysteresis effect in the J–V curves was observed for the mp-SFX-2PA and spiro-OMeTAD based devices (Fig. S8, ESI†); the hysteresis effect of the mp-SFX-2PA based devices is weaker than that of the spiro-OMeTAD based devices.
In order to achieve a higher VOC and PCE, we also fabricated (FAPbI3)0.85(MAPbBr3)0.15 based PSCs using the fast crystallization deposition method. The mp-SFX-2PA based devices with this mixed perovskite layer exhibit a maximum PCE of 17.70%, with a JSC of 20.87 mA cm−2, a VOC of 1083 mV and a FF of 78.31%. The spiro-OMeTAD based devices exhibit a very similar photovoltaic performance, with a maximum PCE of 17.64%, a JSC of 20.82 mA cm−2, a VOC of 1091 mV and a FF of 77.66% (Table 3 and Fig. 5a). The VOC of these FAPbI3/MAPbBr3 based devices is larger than that of MAPbI3 based devices, due to the larger bandgap of the perovskite active layer caused by the introduction of a small proportion of Br. Fig. 5b shows the EQE spectra of the (FAPbI3)0.85(MAPbBr3)0.15 based PSCs employing mp-SFX-2PA and spiro-OMeTAD as HTMs. The calculated JSC is 20.21 and 19.94 mA cm−2 for the mp-SFX-2PA and spiro-OMeTAD based devices, respectively, which have a 3.2 and 4.2% mismatch with those measured using the AM 1.5G solar simulator. Fig. S9a (ESI†) shows the steady-state current density output of the mixed perovskite devices under the maximum power point, with stabilized efficiencies of 16.72 and 16.47% for mp-SFX-2PA and spiro-OMeTAD, respectively, which are close to the values measured from J–V characterization. As shown in Fig. S9b and c (ESI†), the hysteresis effect of the mixed perovskite devices employing mp-SFX-2PA is weaker than that of the devices employing spiro-OMeTAD.
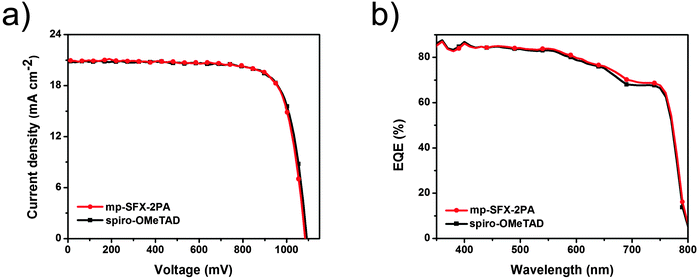 |
| Fig. 5 (a) J–V curves and (b) EQE spectra of the best performing (FAPbI3)0.85(MAPbBr3)0.15 devices based on doped mp-SFX-2PA and doped spiro-OMeTAD. | |
Since efficient interfacial charge transfer between the perovskite and the HTM layer is crucial for the photovoltaic performance of PSCs, time-resolved photoluminescence (PL) decay transient spectra of TiO2/perovskite, TiO2/perovskite/spiro-OMeTAD and TiO2/perovskite/mp-SFX-2PA films were measured in order to evaluate the hole extraction capability of these HTMs (Fig. 4d). From fitting with a bi-exponential decay function, TiO2/MAPbI3 exhibits the longest decay time, with the average decay time of 12.7 ns. After covering the perovskite layer with HTMs, PL quenching becomes much faster, indicating the process of hole transfer at the interface of MAPbI3/HTMs. The TiO2/MAPbI3/spiro-OMeTAD film exhibits a quick decay time, with an average decay time of 2.42 ns. The TiO2/MAPbI3/mp-SFX-2PA film exhibits even faster PL quenching compared with the TiO2/MAPbI3/spiro-OMeTAD film, with an average decay time of 2.05 ns. This result indicates that mp-SFX-2PA can extract and transfer holes more efficiently than sprio-OMeTAD, which is one of the reasons for the slightly improved JSC and weaker hysteresis effect of mp-SFX-2PA based devices than the spiro-OMeTAD based devices.
Electrochemical impedance spectroscopy (EIS) measurements were used to further investigate the charge transfer behaviors at the interface of the solar cells. The Nyquist plots of the representative devices employing mp-SFX-2PA and spiro-OMeTAD were obtained in the applied potential bias from 500 to 900 mV and in the frequency range from 105 to 0.1 Hz (Fig. 6). The inset graph of Fig. 6c shows the equivalent circuit used for simulation of the process of charge transport and recombination in the PSCs,58,59 where Rs is the series resistance of the devices, RHTM and CHTM correspond to the resistance of the HTM/Au interface and its chemical capacitance, and Rrec and Crec correspond to the recombination resistance of the TiO2/perovskite/HTM interface and its chemical capacitance. In the Nyquist plots, the main arc located at lower frequency is related to charge transfer at the TiO2/perovskite/HTM interface, mainly due to the recombination of electrons from mesoporous TiO2 with holes in the HTM.60,61 The recombination rate at the TiO2/perovskite/HTM interface is related to the electron blocking ability of the HTM, and is inversely proportional to Rrec obtained by fitting. The relationships between the bias voltage and Rrec or Rs are shown in Fig. 6c and d. At the same bias voltage, Rrec of the mp-SFX-2PA based device is slightly higher than that of the spiro-OMeTAD based device, indicating that the mp-SFX-2PA based device prevents charge recombination more efficiently than the spiro-OMeTAD based device, which is one of the reasons for the slightly higher VOC of the mp-SFX-2PA based devices. The relationship between bias voltage and Rs exhibits the opposite trend. Rs of the mp-SFX-2PA based device is lower than that of the spiro-OMeTAD based device, which is one of the reasons for the slightly higher FF and JSC of the mp-SFX-2PA based devices.
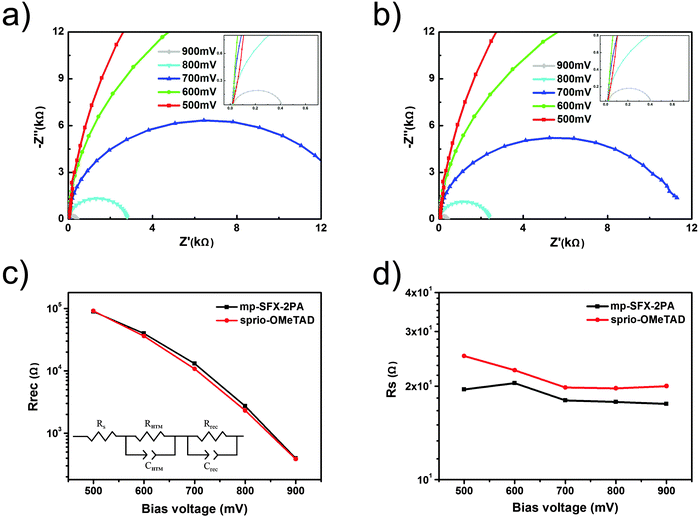 |
| Fig. 6 Nyquist plots of representative MAPbI3 devices based on (a) doped mp-SFX-2PA and (b) doped spiro-OMeTAD measured in the dark under different bias voltage. Inset: The expanded figure in the high frequency range. Relationship between bias voltages and (c) Rrec and (d) Rs for the device employing doped mp-SFX-2PA and doped spiro-OMeTAD. Inset: Equivalent circuit for simulation of the process of charge transfer and recombination. | |
In order to compare the stability of the PSCs employing mp-SFX-2PA and benchmark spiro-OMeTAD, a stability test of these unencapsulated MAPbI3 based devices (10 devices for each) was carried out under ambient conditions (20 ± 5 °C, 15 ± 5% relative humidity). The average PCE of the mp-SFX-2PA based devices retains around 90% of the initial PCEs after 2000 h storage, while the average PCE of the spiro-OMeTAD based devices retains only 28% of the initial PCEs under the same conditions (Fig. 7a). The mp-SFX-2PA based devices show better stability than the spiro-OMeTAD based devices. We monitored the change in hole mobility of doped mp-SFX-2PA and doped spiro-OMeTAD under the same storage conditions (Fig. S10, ESI†). The doped mp-SFX-2PA film retains above 60% of its initial hole mobility after 800 h, while the doped spiro-OMeTAD film retains only 10% of its initial hole mobility. The better hole mobility stability of the doped mp-SFX-2PA is one of the reasons for the better stability of the PSCs. A larger water contact angle of 111.4° was observed for the doped mp-SFX-2PA film, compared with that of the doped spiro-OMeTAD film (80.6°) (Fig. 7b). This result indicates that mp-SFX-2PA could prevent moisture from penetrating into the perovskite layer more efficiently than spiro-OMeTAD, leading to a better stability.28,47,62
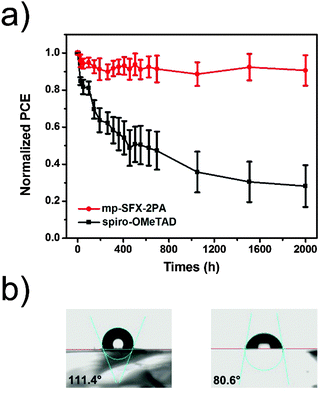 |
| Fig. 7 (a) Stability test of the unencapsulated MAPbI3 based PSCs employing doped mp-SFX-2PA and doped spiro-OMeTAD, stored under ambient conditions (20 ± 5 °C, 15 ± 5% relative humidity). (b) Water contact angles of doped mp-SFX-2PA (left) and doped spiro-OMeTAD (right) thin films. | |
3. Conclusions
We designed and synthesized four SFX based compounds with four triphenylamine or diphenylamine arms at different substitution positions. The triphenylamine and diphenylamine arms exert obvious impacts on absorption and the energy levels of these HTMs, while the effects of the substitution positions on absorption and the energy levels of these HTMs are negligible. The arms and their substitution positions affect the hole mobility values of the HTMs. These compounds exhibit suitable energy levels matching with the valance band of perovskite materials, good solubility, relatively high hole mobility, and efficient hole extracting and electron blocking capability, which are suitable for their potential application in PSCs as HTMs. The MAPbI3 based devices employing mp-SFX-2PA exhibit the best photovoltaic performance among these four HTMs, with an impressive PCE of 16.8%, which is slightly superior to that of the devices based on the widely used spiro-OMeTAD (15.5%) under the same conditions, due to their higher hole mobility, slightly deeper HOMO energy level, and better charge extraction and transport capability. The mp-SFX-2PA based devices employing the mixed FAPbI3/MAPbBr3 perovskite layer exhibit an improved PCE of 17.7%, which is comparable to that of the control device based on spiro-OMeTAD (17.6%). Moreover, the mp-SFX-2PA based devices show much better stability (retain 90% of their initial PCEs after 2000 h storage under ambient atmosphere) than the spiro-OMeTAD based devices (retain only 28% of their initial PCEs), due to better hole mobility stability and the more hydrophobic nature of doped mp-SFX-2PA. This work demonstrates that the SFX based HTMs are potential alternatives for efficient PSCs with improved stability.
4. Experimental section
4.1 Materials
Unless otherwise stated, solvents and chemicals were obtained commercially and were used without further purification. Tris(dibenzylideneacetone)dipalladium(0) (Pd2dba3), tri-tert-butylphosphine (P(t-Bu)3, 10 wt% in hexanes), and sodium tert-butoxide (t-BuONa) were purchased from J&K Inc. Bis(4-methoxyphenyl)amine (2PA) was purchased from Alfa Aesar, and 4-methoxy-N-(4-methoxyphenyl)-N-[4-(4,4,5,5-tetramethyl-1,3,2-dioxaborolan-2-yl)phenyl]benzenamine (3PA-B) was purchased from Sunatech Inc. The TiO2 paste has 20 nm anatase particles and was purchased from Dyesol. PbI2 was purchased from Sigma-Aldrich Inc., N,N-dimethylformide (DMF) and chlorobenzene (CB) from Alfar Aesar, methylamine (AR, 27% in methanol) from Sinopharm Chemical Reagent Co. Ltd, and MAI was self-synthesized.5 Spiro-OMeTAD was purchased from Luminescence Technology Corp., Taiwan. Substrates were FTO coated glasses (Pilkington; thickness: 2.2 mm, sheet resistance: 14 Ω per square). Toluene was distilled from sodium benzophenone under nitrogen prior to use.
4.2 Synthesis
Synthesis of mp-SFX-3PA.
To a three-necked round bottom flask were added SFX-1 (129 mg, 0.2 mmol), 3PA-B (431 mg, 1 mmol), toluene (20 mL) and K2CO3 aqueous solution (2 M, 10 mL). The mixture was deoxygenated with nitrogen for 30 min. Pd(PPh3)4 (60 mg, 0.05 mmol) was added under nitrogen. The mixture was refluxed for 48 h and then cooled down to room temperature. Brine (25 mL) was added and the mixture was extracted with ethyl acetate (2 × 25 mL). The organic phase was dried with anhydrous MgSO4 and filtered. After removing the solvent from the filtrate, the residue was purified by column chromatography on silica gel using ethyl acetate/petroleum ether (1
:
3) as an eluent, yielding a yellowish green solid (200 mg, 64%). 1H NMR (400 MHz, CD2Cl2): δ 7.84 (d, J = 8.0 Hz, 2H), 7.60 (d, J = 8.0 Hz, 2H), 7.41 (s, 2H), 7.38 (m, 6H), 7.30 (d, J = 8.8 Hz, 4H), 7.05 (d, J = 8.8 Hz, 8H), 6.99 (m, 10H), 6.90 (d, J = 8.4 Hz, 4H), 6.84 (m, 20H), 6.51 (d, J = 8.4 Hz, 2H), 3.76 (d, J = 8.8 Hz, 24H). 13C NMR (100 MHz, CD2Cl2): δ 156.30, 156.17, 151.92, 148.64, 148.38, 141.13, 140.93, 140.84, 140.79, 138.15, 132.54, 131.70, 128.37, 127.41, 126.92, 126.67, 126.27, 123.46, 123.15, 121.57, 120.63, 120.44, 120.30, 114.80, 114.74, 114.34, 55.55, 55.53. MS (MALDI): m/z 1544.4 (M+). Anal. calc. for C105H84N4O9: C, 81.58; H, 5.48; N, 3.62%. Found: C, 80.98; H, 5.60; N, 3.96%.
Synthesis of mp-SFX-2PA.
To a three-necked round bottom flask were added SFX-1 (129 mg, 0.2 mmol), 2PA (229 mg, 1 mmol), t-BuONa (114 mg, 1.2 mmol), and toluene (20 mL). The mixture was deoxygenated with nitrogen for 30 min. Pd2dba3 (24 mg, 0.025 mmol) and P(t-Bu)3 (0.5 mL, 10 wt% in hexanes) were added under nitrogen. The mixture was refluxed for 24 h and then cooled down to room temperature. Brine (25 mL) was added and the mixture was extracted with ethyl acetate (2 × 25 mL). The organic phase was dried with anhydrous MgSO4 and filtered. After removing the solvent from the filtrate, the residue was purified by column chromatography on silica gel using ethyl acetate/petroleum ether (1
:
3) as an eluent, yielding a light brownish solid (150 mg, 60%). 1H NMR (400 MHz, acetone-D6): δ 7.57 (d, J = 8.8 Hz, 2H), 7.07 (d, J = 8.8 Hz, 8H), 6.97 (d, J = 8.8 Hz, 8H), 6.94 (d, J = 8.8 Hz, 8H), 6.85 (d, J = 8.8 Hz, 8H), 6.82 (s, 4H), 6.47 (d, J = 8.8 Hz, 2H), 6.40 (d, J = 8.8 Hz, 4H), 3.81 (d, J = 2.8Hz, 24H). 13C NMR (100 MHz, acetone-D6): δ 156.45, 155.91, 155.79, 151.74, 148.90, 148.02, 140.93, 140.94, 140.39, 132.75, 127.84, 126.87, 125.97, 120.44, 119.67, 117.88, 117.09, 115.15, 114.76, 114.57, 106.83, 54.84, 54.81, 53.31. MS (MALDI): m/z 1240.5 (M+). Anal. calc. for C81H68N4O9: C, 78.37; H, 5.52; N, 4.51%. Found: C, 78.38; H, 5.70; N, 4.44%.
Synthesis of mm-SFX-3PA.
To a three-necked round bottom flask were added SFX-2 (129 mg, 0.2 mmol), 3PA-B (431 mg, 1 mmol), toluene (20 mL) and K2CO3 aqueous solution (2 M, 10 mL). The mixture was deoxygenated with nitrogen for 30 min. Pd(PPh3)4 (60 mg, 0.05 mmol) was added under nitrogen. The mixture was refluxed for 48 h and then cooled down to room temperature. Brine (25 mL) was added and the mixture was extracted with ethyl acetate (2 × 25 mL). The organic phase was dried with anhydrous MgSO4 and filtered. After removing the solvent from the filtrate, the residue was purified by column chromatography on silica gel using ethyl acetate/petroleum ether (1
:
3) as an eluent, yielding a yellowish green solid (100 mg, 32%). 1H NMR (400 MHz, CD2Cl2): δ 7.79 (d, J = 7.6 Hz, 2H), 7.56 (d, J = 7.6 Hz, 2H), 7.36 (s, 4H), 7.29 (d, J = 6.8 Hz, 6H), 6.98 (d, J = 7.6 Hz, 12H), 6.93 (d, J = 8.4 Hz, 8H), 6.83 (m, 24H), 6.62 (s, 2H), 3.74 (d, J = 5.2 Hz, 24H). 13C NMR (100 MHz, CD2Cl2): δ 156.80, 156.72, 151.27, 148.97, 148.61, 141.55, 141.50, 138.82, 136.85, 133.23, 132.85, 128.07, 127.74, 127.31, 127.22, 127.09, 126.96, 126.36, 126.04, 123.96, 121.32, 121.28, 121.16, 117.90, 115.38, 115.34, 56.18, 55.44. MS (MALDI): m/z 1545.5 (M+). Anal. calc. for C105H84N4O9: C, 81.58; H, 5.48; N, 3.62%. Found: C, 81.36; H, 5.40; N, 3.58%.
Synthesis of mm-SFX-2PA.
To a three-necked round bottom flask were added SFX-2 (129 mg, 0.2 mmol), 2PA (229 mg, 1 mmol), t-BuONa (114 mg, 1.2 mmol), and toluene (20 mL). The mixture was deoxygenated with nitrogen for 30 min. Pd2dba3 (24 mg, 0.025 mmol) and P(t-Bu)3 (0.5 mL, 10 wt% in hexanes) were added under nitrogen. The mixture was refluxed for 24 h and then cooled down to room temperature. Brine (25 mL) was added and the mixture was extracted with ethyl acetate (2 × 25 mL). The organic phase was dried with anhydrous MgSO4 and filtered. After removing the solvent from the filtrate, the residue was purified by column chromatography on silica gel using ethyl acetate/petroleum ether (1
:
3) as an eluent, yielding a light brownish solid (70 mg, 28%). 1H NMR (400 MHz, acetone-D6): δ 7.39 (d, J = 8.4 Hz, 2H), 7.00 (s, 1H), 6.97 (d, J = 8.8 Hz, 8H), 6.87 (d, J = 9.2 Hz, 8H), 6.84 (m, 16H), 6.80 (m, 5H), 6.76 (d, J = 8.4 Hz, 2H), 6.34 (d, J = 2.4 Hz, 2H), 3.80 (m, 24H) 13C NMR (100 MHz, acetone-D6): δ 157.47, 157.05, 156.88, 149.57, 147.78, 145.26, 142.78, 142.38, 133.96, 127.52, 126.85, 126.18, 124.79, 123.16, 122.12, 121.21, 118.77, 118.70, 116.11, 115.99, 56.31, 55.81. MS (MALDI): m/z 1240.3 (M+). Anal. calc. for C81H68N4O9: C, 78.37; H, 5.52; N, 4.51%. Found: C, 78.11; H, 5.72; N, 4.60%.
4.3 Device fabrication
Perovskite solar cells were fabricated using the following procedure. First of all, FTO coated glass substrates were cleaned under ultra-sonication sequentially in soap water, dilute NaOH solution, deionized water and ethanol for 20 min each. Next, a 30 nm thick TiO2 compacted layer was deposited on the pre-cleaned substrates by spin coating the precursor solution of titanium tetraisopropanolate in ethanol at 2000 rpm. After sintering at 500 °C for 30 min, a 300 nm thick mesoporous TiO2 layer was deposited on the compacted TiO2 layer by spin coating diluted TiO2 paste (1
:
3, v/v diluted with ethanol) at 4000 rpm and sintering at 500 °C for 30 min. After that, the mesoporous TiO2 film was treated with 25 mM aqueous TiCl4 solution at 70 °C and sintered at 500 °C for 30 min again. The MAPbI3 based perovskite layer was deposited in a glove box using repeated sequential deposition methods according to the previous literature.63 1.3 M PbI2 dissolved in DMF was spin coated on the mesoporous TiO2 film at 3500 rpm and heated at 90 °C to remove the solvent. After cooling down to room temperature, the PbI2 film was immersed into 10 mg mL−1 MAI in isopropanol at 100 °C for 3 min. The HTM layers were spin coated on the perovskite layer at 2500 rpm. The composition of the HTM solutions was 70 mg for mp-SFX-3PA, mp-SFX-2PA, mm-SFX-3PA and mm-SFX-2PA, and 72 mg for spiro-OMeTAD in 1 mL CB, 35 µL 4-tert-butylpyridine, and doped with 60 µL LiTFSI in acetonitrile (150 mg mL−1) for mp-SFX-3PA, 67 µL for mp-SFX-2PA, 87 µL for mm-SFX-3PA, 67 µL for mm-SFX-2PA and 61 µL for spiro-OMeTAD. Finally, an 80 nm thick Au counter electrode was deposited on the top of the HTM layer by thermal evaporation at a pressure of 10−6 Torr. The (FAPbI3)0.85(MAPbBr3)0.15 thin film was fabricated according to the previous literature.19
4.4 Characterization
NMR spectra were measured on a Bruker AVANCE 400 MHz spectrometer. Mass spectra were measured on a Bruker Daltonics BIFLEX III MALDI-TOF Analyzer using MALDI mode. Elemental analyses were carried out using a FLASH EA1112 elemental analyzer. TGA measurements were performed on a Shimadzu thermogravimetric analyzer (model DTG-60) under a nitrogen flow at a heating rate of 10 °C min−1. UV-vis absorption spectra of solution (DCM, 10−6 M) and thin-film (on quarts substrate) were recorded on a JASCO V-570 spectrometer. CV measurements were carried out under nitrogen in a deoxygenated solution of tetra-n-butylammonium hexafluorophosphate (0.1 M) in acetonitrile (CH3CN) using a computer-controlled CHI660C electrochemical workstation, a glassy-carbon working electrode coated with samples, a platinum-wire auxiliary electrode, and Ag/AgCl as a reference electrode. Potentials were referenced to the ferrocenium/ferrocene (FeCp0/+2) couple by using ferrocene as an internal standard. Hole mobility was estimated using a hole-only device with a configuration of ITO/PEDOT:PSS/HTM/Au. J–V characteristics were carried out under dark conditions, and the hole mobility was estimated using Mott–Gurney's equation, J = 9εrε0μhV2/8L3, where εr is the relative dielectric constant of the transport medium (for organic materials, εr is 3), ε0 is the permittivity of free space (8.85 × 10−12 F m−1), L is the thickness of the HTM layer, and V is the voltage drop through the device. Cross-sectional SEM images were obtained using a Hitachi S-4800 SEM. The HOMO energy level was also estimated using UPS (AXIS ultra DLD). UPS spectra were obtained from He I (21.22 eV) excitation lines with a sample bias of 9 V. The photovoltaic performance was characterized using a Keithley 2602 system source meter and a sunlight simulator of Oriel Solar Simulator 91192 with irradiation of AM 1.5G, 100 mW cm−2, calibrated with the standard silicon reference cell. When testing, PSCs were masked with a black aperture to confine the active area of the device to 0.1 cm2. EQE spectra were measured using a lab-made spectrometer with wavelength ranging from 350 to 850 nm. Time-resolved PL decay transient spectra were obtained using a PL spectrometer (Edinburgh Instruments, FLS 900), excited with a pulsed diode laser (EPL-445, 0.8 µJ cm−2) and measured at 775 nm after excitation at 445 nm. EIS measurements were carried out using an IM6ex electrochemical workstation (ZAHNER), in which the scanning frequency was set between 0.1 and 105 Hz, and the amplitude of the sine perturbation bias was set to 10 mV.
Acknowledgements
We thank the National Key Basic Research Program of China (973) (No. 2013CB834702, 2015CB932200), the National Natural Science Funds for Excellent Young Scholar (No. 21322402), the National Natural Science Foundation of China (No. U1301243), the Doctoral Fund of Ministry of Education of China (No. 20133223110007), and the Excellent Science and Technology Innovation Team of Jiangsu Higher Education Institutions (2013) for financial support. The Supercomputing Center of Chinese Academy of Sciences is acknowledged for molecular modeling.
Notes and references
- M. Gratzel, Nat. Mater., 2014, 13, 838 CrossRef CAS PubMed
.
- M. A. Green, A. Ho-Baillie and H. J. Snaith, Nat. Photonics, 2014, 8, 506 CrossRef CAS
.
- T. C. Sum and N. Mathews, Energy Environ. Sci., 2014, 7, 2518 CAS
.
- A. Kojima, K. Teshima, Y. Shirai and T. Miyasaka, J. Am. Chem. Soc., 2009, 131, 6050 CrossRef CAS PubMed
.
- H. S. Kim, C. R. Lee, J. H. Im, K. B. Lee, T. Moehl, A. Marchioro, S. J. Moon, R. Humphry-Baker, J. H. Yum, J. E. Moser, M. Gratzel and N. G. Park, Sci. Rep., 2012, 2, 591 Search PubMed
.
- M. M. Lee, J. Teuscher, T. Miyasaka, T. N. Murakami and H. J. Snaith, Science, 2012, 338, 643 CrossRef CAS PubMed
.
- J. Burschka, N. Pellet, S. J. Moon, R. Humphry-Baker, P. Gao, M. K. Nazeeruddin and M. Gratzel, Nature, 2013, 499, 316 CrossRef CAS PubMed
.
- M. Liu, M. B. Johnston and H. J. Snaith, Nature, 2013, 501, 395 CrossRef CAS PubMed
.
- N. J. Jeon, J. H. Noh, Y. C. Kim, W. S. Yang, S. Ryu and S. I. Seok, Nat. Mater., 2014, 13, 897 CrossRef CAS PubMed
.
- D. Liu and T. L. Kelly, Nat. Photonics, 2014, 8, 133 CrossRef CAS
.
- H. Zhou, Q. Chen, G. Li, S. Luo, T. B. Song, H. S. Duan, Z. Hong, J. You, Y. Liu and Y. Yang, Science, 2014, 345, 542 CrossRef CAS PubMed
.
- N. J. Jeon, J. H. Noh, W. S. Yang, Y. C. Kim, S. Ryu, J. Seo and S. I. Seok, Nature, 2015, 517, 476 CrossRef CAS PubMed
.
- W. S. Yang, J. H. Noh, N. J. Jeon, Y. C. Kim, S. Ryu, J. Seo and S. I. Seok, Science, 2015, 348, 1234 CrossRef CAS PubMed
.
- J. M. Ball, M. M. Lee, A. Hey and H. J. Snaith, Energy Environ. Sci., 2013, 6, 1739 CAS
.
- J. H. Heo, S. H. Im, J. H. Noh, T. N. Mandal, C.-S. Lim, J. A. Chang, Y. H. Lee, H.-J. Kim, A. Sarkar, M. K. Nazeeruddin, M. Grätzel and S. I. Seok, Nat. Photonics, 2013, 7, 486 CrossRef CAS
.
- J. H. Noh, S. H. Im, J. H. Heo, T. N. Mandal and S. I. Seok, Nano Lett., 2013, 13, 1764 CrossRef CAS PubMed
.
- N. J. Jeon, H. G. Lee, Y. C. Kim, J. Seo, J. H. Noh, J. Lee and S. I. Seok, J. Am. Chem. Soc., 2014, 136, 7837 CrossRef CAS PubMed
.
- Z. Yu and L. Sun, Adv. Energy Mater., 2015, 5, 1500213 CrossRef
.
- D. Bi, W. Tress, M. I. Dar, P. Gao, J. Luo, C. Renevier, K. Schenk, A. Abate, F. Giordano, J.-P. Correa Baena, J.-D. Decoppet, S. M. Zakeeruddin, M. K. Nazeeruddin, M. Grätzel and A. Hagfeldt, Sci. Adv., 2016, 2, e1501170 Search PubMed
.
- S. Chavhan, O. Miguel, H.-J. Grande, V. Gonzalez-Pedro, R. S. Sánchez, E. M. Barea, I. Mora-Seró and R. Tena-Zaera, J. Mater. Chem. A, 2014, 2, 12754 CAS
.
- J. A. Christians, R. C. Fung and P. V. Kamat, J. Am. Chem. Soc., 2014, 136, 758 CrossRef CAS PubMed
.
- J. Y. Jeng, K. C. Chen, T. Y. Chiang, P. Y. Lin, T. D. Tsai, Y. C. Chang, T. F. Guo, P. Chen, T. C. Wen and Y. J. Hsu, Adv. Mater., 2014, 26, 4107 CrossRef CAS PubMed
.
- K.-C. Wang, J.-Y. Jeng, P.-S. Shen, Y.-C. Chang, E. W.-G. Diau, C.-H. Tsai, T.-Y. Chao, H.-C. Hsu, P.-Y. Lin, P. Chen, T.-F. Guo and T.-C. Wen, Sci. Rep., 2014, 4, 4756 Search PubMed
.
- H. Li, K. Fu, A. Hagfeldt, M. Gratzel, S. G. Mhaisalkar and A. C. Grimsdale, Angew. Chem., Int. Ed., 2014, 53, 4085 CrossRef CAS PubMed
.
- J. Liu, Y. Wu, C. Qin, X. Yang, T. Yasuda, A. Islam, K. Zhang, W. Peng, W. Chen and L. Han, Energy Environ. Sci., 2014, 7, 2963 CAS
.
- J. Wang, S. Wang, X. Li, L. Zhu, Q. Meng, Y. Xiao and D. Li, Chem. Commun., 2014, 50, 5829 RSC
.
- Y. Liu, Z. Hong, Q. Chen, H. Chen, W. H. Chang, Y. M. Yang, T. B. Song and Y. Yang, Adv. Mater., 2016, 28, 440 CrossRef CAS PubMed
.
- S. S. Reddy, K. Gunasekar, J. H. Heo, S. H. Im, C. S. Kim, D. H. Kim, J. H. Moon, J. Y. Lee, M. Song and S. H. Jin, Adv. Mater., 2016, 28, 686 CrossRef CAS PubMed
.
- K. Do, H. Choi, K. Lim, H. Jo, J. W. Cho, M. K. Nazeeruddin and J. Ko, Chem. Commun., 2014, 50, 10974 RSC
.
- P. Qin, S. Paek, M. I. Dar, N. Pellet, J. Ko, M. Gratzel and M. K. Nazeeruddin, J. Am. Chem. Soc., 2014, 136, 8516 CrossRef CAS PubMed
.
- S. D. Sung, M. S. Kang, I. T. Choi, H. M. Kim, H. Kim, M. Hong, H. K. Kim and W. I. Lee, Chem. Commun., 2014, 50, 14161 RSC
.
- H. Choi, J. W. Cho, M. S. Kang and J. Ko, Chem. Commun., 2015, 51, 9305 RSC
.
- K. Rakstys, A. Abate, M. I. Dar, P. Gao, V. Jankauskas, G. Jacopin, E. Kamarauskas, S. Kazim, S. Ahmad, M. Gratzel and M. K. Nazeeruddin, J. Am. Chem. Soc., 2015, 137, 16172 CrossRef CAS PubMed
.
- C. Huang, W. Fu, C. Z. Li, Z. Zhang, W. Qiu, M. Shi, P. Heremans, A. K. Jen and H. Chen, J. Am. Chem. Soc., 2016, 138, 2528 CrossRef CAS PubMed
.
- N. J. Jeon, J. Lee, J. H. Noh, M. K. Nazeeruddin, M. Gratzel and S. I. Seok, J. Am. Chem. Soc., 2013, 135, 19087 CrossRef CAS PubMed
.
- T. Krishnamoorthy, F. Kunwu, P. P. Boix, H. Li, T. M. Koh, W. L. Leong, S. Powar, A. Grimsdale, M. Grätzel, N. Mathews and S. G. Mhaisalkar, J. Mater. Chem. A, 2014, 2, 6305 CAS
.
- B. Xu, E. Sheibani, P. Liu, J. Zhang, H. Tian, N. Vlachopoulos, G. Boschloo, L. Kloo, A. Hagfeldt and L. Sun, Adv. Mater., 2014, 26, 6629 CrossRef CAS PubMed
.
- M. Franckevičius, A. Mishra, F. Kreuzer, J. Luo, S. M. Zakeeruddin and M. Grätzel, Mater. Horiz., 2015, 2, 613 RSC
.
- Y.-K. Wang, Z.-C. Yuan, G.-Z. Shi, Y.-X. Li, Q. Li, F. Hui, B.-Q. Sun, Z.-Q. Jiang and L.-S. Liao, Adv. Funct. Mater., 2016, 26, 1375 CrossRef CAS
.
- B. Cai, Y. Xing, Z. Yang, W.-H. Zhang and J. Qiu, Energy Environ. Sci., 2013, 6, 1480 CAS
.
- F. Di Giacomo, S. Razza, F. Matteocci, A. D'Epifanio, S. Licoccia, T. M. Brown and A. Di Carlo, J. Power Sources, 2014, 251, 152 CrossRef CAS
.
- Y. S. Kwon, J. Lim, H.-J. Yun, Y.-H. Kim and T. Park, Energy Environ. Sci., 2014, 7, 1454 CAS
.
- O. Malinkiewicz, A. Yella, Y. H. Lee, G. M. Espallargas, M. Graetzel, M. K. Nazeeruddin and H. J. Bolink, Nat. Photonics, 2013, 8, 128 CrossRef
.
- P. Qin, H. Kast, M. K. Nazeeruddin, S. M. Zakeeruddin, A. Mishra, P. Bäuerle and M. Grätzel, Energy Environ. Sci., 2014, 7, 2981 CAS
.
- C. Roldán-Carmona, O. Malinkiewicz, A. Soriano, G. Mínguez Espallargas, A. Garcia, P. Reinecke, T. Kroyer, M. I. Dar, M. K. Nazeeruddin and H. J. Bolink, Energy Environ. Sci., 2014, 7, 994 Search PubMed
.
- S. Ryu, J. H. Noh, N. J. Jeon, Y. Chan Kim, W. S. Yang, J. Seo and S. I. Seok, Energy Environ. Sci., 2014, 7, 2614 CAS
.
- L. Zheng, Y. H. Chung, Y. Ma, L. Zhang, L. Xiao, Z. Chen, S. Wang, B. Qu and Q. Gong, Chem. Commun., 2014, 50, 11196 RSC
.
- G.-W. Kim, J. Kim, G.-Y. Lee, G. Kang, J. Lee and T. Park, Adv. Energy Mater., 2015, 5, 1500471 CrossRef
.
- L.-H. Xie, F. Liu, C. Tang, X.-Y. Hou, Y.-R. Hua, Q.-L. Fan and W. Huang, Org. Lett., 2006, 8, 2787 CrossRef CAS PubMed
.
- Y.-H. Tseng, P.-I. Shih, C.-H. Chien, A. K. Dixit, C.-F. Shu, Y.-H. Liu and G.-H. Lee, Macromolecules, 2005, 38, 10055 CrossRef CAS
.
- J.-F. Gu, G.-H. Xie, L. Zhang, S.-F. Chen, Z.-Q. Lin, Z.-S. Zhang, J.-F. Zhao, L.-H. Xie, C. Tang, Y. Zhao, S.-Y. Liu and W. Huang, J. Phys. Chem. Lett., 2010, 1, 2849 CrossRef CAS
.
- Y. Qian, G. Xie, S. Chen, Z. Liu, Y. Ni, X. Zhou, L. Xie, J. Liang, Y. Zhao, M. Yi, Y. Zhao, W. Wei and W. Huang, Org. Electron., 2012, 13, 2741 CrossRef CAS
.
- B. Xu, D. Bi, Y. Hua, P. Liu, M. Cheng, M. Gratzel, L. Kloo, A. Hagfeldt and L. Sun, Energy Environ. Sci., 2016, 9, 873 CAS
.
-
M. J. Frisch, G. W. Trucks, H. B. Schlegel, G. E. Scuseria, M. A. Robb, J. R. Cheeseman, G. Scalmani, V. Barone, B. Mennucci, G. A. Petersson, H. Nakatsuji, M. Caricato, X. Li, H. P. Hratchian, A. F. Izmaylov, J. Bloino, G. Zheng, J. L. Sonnenberg, M. Hada, M. Ehara, K. Toyota, R. Fukuda, J. Hasegawa, M. Ishida, T. Nakajima, Y. Honda, O. Kitao, H. Nakai, T. Vreven, J. A. Montgomery Jr., J. E. Peralta, F. Ogliaro, M. J. Bearpark, J. Heyd, E. N. Brothers, K. N. Kudin, V. N. Staroverov, R. Kobayashi, J. Normand, K. Raghavachari, A. P. Rendell, J. C. Burant, S. S. Iyengar, J. Tomasi, M. Cossi, N. Rega, N. J. Millam, M. Klene, J. E. Knox, J. B. Cross, V. Bakken, C. Adamo, J. Jaramillo, R. Gomperts, R. E. Stratmann, O. Yazyev, A. J. Austin, R. Cammi, C. Pomelli, J. W. Ochterski, R. L. Martin, K. Morokuma, V. G. Zakrzewski, G. A. Voth, P. Salvador, J. J. Dannenberg, S. Dapprich, A. D. Daniels, Ö. Farkas, J. B. Foresman, J. V. Ortiz, J. Cioslowski and D. J. Fox, Gaussian, Inc., Wallingford, CT, USA, 2009
.
- R. Krishnan, J. S. Binkley, R. Seeger and J. A. Pople, J. Chem. Phys., 1980, 72, 650 CrossRef CAS
.
- C. Lee, W. Yang and R. G. Parr, Phys. Rev. B: Condens. Matter Mater. Phys., 1988, 37, 785 CrossRef CAS
.
- A. D. Becke, J. Chem. Phys., 1993, 98, 5648 CrossRef CAS
.
- H. S. Kim, J. W. Lee, N. Yantara, P. P. Boix, S. A. Kulkarni, S. Mhaisalkar, M. Gratzel and N. G. Park, Nano Lett., 2013, 13, 2412 CrossRef CAS PubMed
.
- V. Gonzalez-Pedro, E. J. Juarez-Perez, W. S. Arsyad, E. M. Barea, F. Fabregat-Santiago, I. Mora-Sero and J. Bisquert, Nano Lett., 2014, 14, 888 CrossRef CAS PubMed
.
- M. Cheng, B. Xu, C. Chen, X. Yang, F. Zhang, Q. Tan, Y. Hua, L. Kloo and L. Sun, Adv. Energy Mater., 2015, 5, 1401720 CrossRef
.
- L. Zhu, J. Xiao, J. Shi, J. Wang, S. Lv, Y. Xu, Y. Luo, Y. Xiao, S. Wang, Q. Meng, X. Li and D. Li, Nano Res., 2014, 8, 1116 CrossRef
.
- Y. Hou, H. Zhang, W. Chen, S. Chen, C. O. R. Quiroz, H. Azimi, A. Osvet, G. J. Matt, E. Zeira, J. Seuring, N. Kausch-Busies, W. Lövenich and C. J. Brabec, Adv. Energy Mater., 2015, 5, 1500543 CrossRef
.
- L. Zhu, J. Shi, S. Lv, Y. Yang, X. Xu, Y. Xu, J. Xiao, H. Wu, Y. Luo, D. Li and Q. Meng, Nano Energy, 2015, 15, 540 CrossRef CAS
.
Footnote |
† Electronic supplementary information (ESI) available: Synthesis routes, simulation results, TGA curves, UV-vis absorption spectra in solutions, SCLC curves, the effect of mp-SFX-2PA thickness, the performance of PSCs based on spiro-OMeTAD, statistical distribution histogram, J–V curves under different scanning conditions, steady-state current density and power output of (FAPbI3)0.85(MAPbBr3)0.15 based PSCs, and mobility stability curves. See DOI: 10.1039/c6qm00097e |
|
This journal is © the Partner Organisations 2017 |
Click here to see how this site uses Cookies. View our privacy policy here.