DOI:
10.1039/C6RA17949E
(Paper)
RSC Adv., 2017,
7, 9662-9668
A feasible approach to dispose of soil washing wastes: adsorptive removal of chlorobenzene compounds in aqueous solutions using humic acid modified with monoolein (HA–M)†
Received
14th July 2016
, Accepted 18th January 2017
First published on 1st February 2017
Abstract
Humic acid (HA), one of most ubiquitous natural resources in the world, was modified with monoolein to prepare a novel hydrophobic adsorbent (HA–M) for removal of chlorobenzene compounds (CBs) from aqueous solutions. The influence of pH, temperature, coexisting organics and initial concentration on the adsorption capacity of HA–M for CBs were investigated to obtain the optimum removal efficiency. Furthermore, the adsorption kinetics and isotherms were researched as well as the column adsorption studies to reveal the mechanism for HA–M to adsorb CBs. The experimental results indicated that the HA–M materials exhibit high removal efficiency of CBs (>90%) in a wide range of pH values and temperatures. The adsorption of CBs with HA–M was realized by the hydrophobic interactions between CBs and HA–M. In addition, the HA–M also performed high removal efficiency toward organochlorine pesticides (OCPs) in aqueous solutions. Most important, the excellent adsorbability of the super-hydrophobic HA–M adsorbent could be comparable or fairly good compared with other adsorbents. Consequently, our present investigations provided a facile synthesis route for the highly effective and recyclable hydrophobic adsorbent for the adsorptive removal of CBs and OCPs from aqueous solutions.
Introduction
Chlorobenzene compounds (CBs) are conventionally used as effective components in commercial pesticides owing to their low cost, and high efficiency in insect disinfestation,1 fungicides,2 and deodorants.3 The CBs-containing pesticides have been widely used in many countries, which may result in serious leakage of CBs into the soil. However, CBs have poor water solubility with high toxicity, are difficult to decompose in the natural environment (aqueous and soil environment) and can easily accumulate in fatty tissues of organs, causing potential threats to people's health via the food chains.4,5 Although the use of several CBs with extreme toxicity, such as hexachlorobenzene (HCB), has been banned in agriculture in most countries,6 many new types of CBs (e.g. tetradifon and hexythiazox) are still used in producing pesticides. Unfortunately, the utilization of these CB-containing pesticides inevitably generates a large amount of polluted soil sites due to the stability of CBs. Currently, the problem of highly CB-polluted soil has becoming one of the most worrying issues in environmental protection. Soil washing is one of the most efficient technologies for removing of CBs from polluted site soil.
However, further issues arise following the soil washing since the collected large volume of waste washing solution, (e.g. toxic metals,7 persistent organic pollutants,8) need to be adopted suitable treatments. Various strategies have been developed to address the attendant organic contamination problems in soil washing solutions, including biological degradation,9 photo-catalysis,10 and adsorption.11 As for bioremediation, it usually requires a long degradation cycle to decompose the containments, and this degradation process is even time-consuming, especially for those organic hydrophobic containments.12,13 Photo-catalysis is a recently emerged promising strategy to solve the contamination of soil washing wastes, while its practical application is still hampered by difficulty in catalyst recycling and low catalytic efficiency under visible light.14,15 On the contrary, adsorption is currently the most promising and practical applicable approach for the purification of aqueous solutions with a large volume and variety of washing contaminations because it's distinct advantages of low-cost, high efficiency and recyclable treatment capacity.
Along that line, great effort has been dedicated to developing adsorbents of CBs, including activated carbon,16 porous metal–organic framework,17 and DNA hydrogel beads,18 etc. Although these adsorbent indeed exhibit adsorption capacities to CBs in aqueous solutions, their adsorption capacity, treatment efficiency and/or recycling ability are still have a plenty of room to improve. In principle, the lipoid-type adsorbents should be ideal candidates for CBs removal when considering the hydrophobic nature of CBs. This type of adsorbent is able to adsorb the CBs through a solid extraction mechanism, and desorption by organic solvent can be easily carried out to recycle the adsorbent. Several groups have found that lipoid, such as biolipoid-triolein, shows great potential for effective removal of trace amount of CBs from aqueous solution, with a high accumulating capacity (105–107).19,20 However, these lipoid materials are in liquid status, which makes it difficult to recycle. To facilitate the recycle, these lipoids need to be immobilized onto solid matrices to prepare water insoluble adsorbents.
To date, most of the reported lipoid-type adsorbents rely on physical interactions to immobilize lipoid species.21 Owing to the weak interactions, the immobilized lipoids often suffer from serious detaching from the supporting matrix, resulting in decreased adsorption capacity upon cycling. To this end, it is essential to enhance the stability of the lipoid species on the supporting matrices by chemical grafting. On the other hand, the adsorption kinetics of adsorbent is closely related to the porosity and specific surface area of supporting matrices, which accordingly determine the adsorption rate and kinetics parameters. All these issues must be taken into consideration when developing novel adsorbents to CBs, which should have high capacity, fast adsorption rate and good cycling stability.
Here, humic acid (HA) which was derived from plants and microbial residues was selected as the supporting matrix because it has well-defined porosity and large specific surface area, favoring the access of CBs to inner pores of HA immobilized with monoolein adsorbents (HA–M). Moreover, HA has abundant functional group (Fig. 1a),22,23 including –OH and –COOH, etc., which enables an easy and stable chemical grafting of monoolein (the chemical structure of monoolein was shown in Fig. S1†) onto HA via etherification. HA is also rich in earth, which ensures low-cost of the prepared HA–M. To evaluate the adsorption properties of HA–M to CBs, 12 kinds of CBs and were utilized as the target contaminations in aqueous solutions. Batch experiments were carried out by investigating the influences of pH and coexisting organics on the adsorption capacity of CBs, the adsorption kinetics, and the adsorption isotherms were systematically investigated as well. Column adsorption experiment was also conducted to investigate the dynamic adsorption efficiency of CBs in aqueous solutions. Furthermore, 4 types of organochlorine pesticides (OCPs) were also utilized to investigate the adsorption properties of HA–M toward the commercial pesticides. The HA–M has exhibited satisfactory performance in removal of CBs and OCPs in aqueous solution.
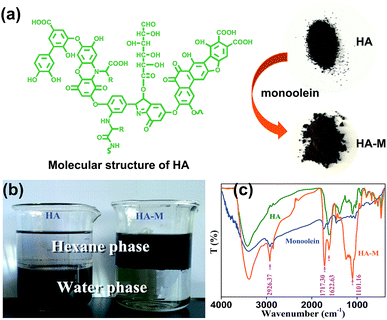 |
| Fig. 1 (a) Molecular structure of HA, (b) photograph of HA and HA–M in water/hexane biphasic system, (c) FT-IR spectra of HA, monoolein and HA–M. | |
Results and discussion
Characterization of HA–M adsorbent
HA is one of the ubiquitous resources in natural aquatic environment, which contains a variety of functional groups in its structure, such as hydroxyl, carbonyl, carboxyl and phenol (see Fig. 1a). As shown in Fig. 1b, HA only disperses in water phase since the hydrophilic hydroxyls have endowed HA with enough hydrophilicity, while the HA–M adsorbent just disperses in organic phase after the modification with monoolein, which suggests that the hydrophobic monoolein has been grafted onto HA. Hence, the as-obtained HA–M is a typical lipoid-type hydrophobic adsorbent. Additionally, HA–M can finely disperse well in organic/aqueous heterogeneous system following with constant shaking.
FT-IR spectra of HA, monoolein and HA–M are shown in Fig. 1c. The absorption band near 3400 cm−1 confirms the existence of hydroxyls in HA–M. The aliphatic characteristic representing the absorption band at 2926.37 cm−1 indicates that monoolein has been successfully grafted onto HA.24,25 The bands at 1622.53 cm−1 was attributed to aromatic –C
C stretching. The appearance of band at 1717.30 cm−1 belongs to the carboxyl groups (–COOH) in HA–M.26 In addition, the stretching vibration peak of –C–O– group with obvious increased intensity around 1101.16 cm−1, which could be attributed to the esterification of HA with monoolein.27
Effect of pH on the adsorption capacity of CBs
The adsorptions of HA–M to five typical CBs with proper concentration (50 mg L−1) were investigated in a wide range of pH. As shown in Fig. S3,† the adsorption capacities of HA–M to all the CBs are close to 23 mg g−1, and slightly change in the range pH of 3–9, which is different from the adsorption to metal ions. A higher pH value can promote the dissociation of hydroxyls in HA–M, increasing the chelating adsorption to metal ions. However, the CBs are hydrophobic compounds without electron accepting ability. Hence, the adsorption of HA–M to CBs is more likely attributed to the hydrophobic interactions between HA–M and CBs, where the benzene rings in the HA–M can form “hydrophobic bond” with CBs. The slight influence of pH on adsorption capacity suggests that the removal of CBs by HA–M can be carried out in a wide range of pH, which is preferred in practical applications, due to the excellent hydrophobicity of HA–M. In consideration of environmental benign issue, the solution pH was fixed at 7.0 in the subsequent adsorption experiments. Additionally, control experiment suggested that the organic membrane filter had little adsorption to CBs.
Adsorption kinetic studies
Adsorption kinetic studies can provide valuable parameters to simulate specific treatment process. The equilibrium constant and adsorption isotherm were measured at constant HA–M amount (0.2 g) while varying types of CBs. The adsorption capacity of HA–M to CBs was high up to 18 mg g−1 in the initial 1 h of adsorption process, and the adsorption equilibrium was achieved around 8 hours with 80% of CBs removal efficiency. The fast adsorption rate could be explained by the strong hydrophobic interactions between HA–M and CBs. Furthermore, the mesoporous structure of HA–M is another important causation. The mesoporous structure of HA–M (Fig. S4†) was beneficial for the convenient accesses of CBs into the inner pores, which substantially decreases the mass transfer resistance usually encountered in microporous adsorbents.28,29
As shown in Fig. 2 and S5a,† the pseudo-first order kinetics cannot fit the adsorption kinetics (R2 < 0.98) while the pseudo-second order model can give a well description with a high correlation constant (R2 > 0.98) (Table S1†). The calculated equilibrium adsorption capacity of each CBs obtain from the pseudo-second order model fitting is very close to the adsorption capacity determined by experiments. For example, the Qe(cal) of HCB is 23.088 mg g−1 while the Qe(exp) of HCB is 23.882 mg g−1. The intraparticle diffusion model was also used to simulate the adsorption kinetic data but the low correlation constant suggests that the inner-diffusion resistance can be neglected during the adsorption (Fig. S5b†). Among the three models, it is obvious that the pseudo-second-order model can well describe the adsorption behaviors of HA–M to CBs. In this regard, “solid phase extraction” should be the rate controlling step in the adsorption of CBs with HA–M. The mesoporous structure of HA–M could alleviate the inner-diffusion resistance efficiently.
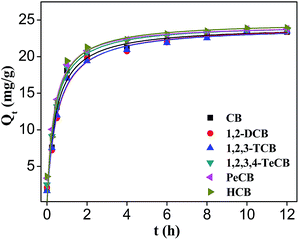 |
| Fig. 2 Adsorption kinetics of HA–M to CBs; pseudo-second-order model fitting. | |
Adsorption isotherm studies
To evaluate the theoretical adsorption capacity of HA–M to CBs, the adsorption isotherms of CBs on HA–M were investigated, and fitted by the Langmuir and Freundlich models (Fig. 3). As shown in Fig. S6 and Table S2,† the Freundlich model fits the adsorption isotherms very well, and all the values of 1/n are smaller than 1, which suggest that the adsorption of CBs on HA–M is easy to proceed.30 From the Freundlich model, we can obtain the maximum adsorption capacity. At room temperature (25 °C), the maximum adsorption capacities of HA–M to the 12 kinds of CBs could reach 120 mg g−1, showing superior capacities compared to previously reported absorbents (Table S3†) such as activated montmorillonite31 and grapheme.32 This should be attributed to the strong hydrophobic properties of HA–M, which provide high affinity toward the hydrophobic CBs.
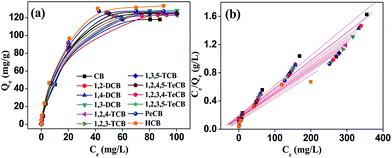 |
| Fig. 3 Adsorption isotherms of CBs onto HA–M (a) and adsorption isotherm plots for adsorption of CBs onto HA–M, Langmuir model (b). | |
Adsorption thermodynamics studies
Thermodynamics studies were also carried out to investigate adsorption of CBs with HA–M. The adsorption capacities of HA–M to six selected CBs were researched from 15 °C to 45 °C. The adsorption capacities slightly decreased slightly along with the rise of temperature (Fig. S7†). To explain this phenomenon, the thermodynamic parameters are evaluated using eqn (1) and (2):33,34 |
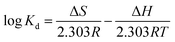 | (1) |
|
ΔG = −RT ln Kd
| (2) |
(Kd = Qe/Ce: adsorption constant, ΔS: entropy change, ΔH: enthalpy change, ΔG: Gibb's free energy change).
Concretely, these parameters were obtained through fitting log
Kd with 1/T. All of thermodynamic parameters are given in Table S4.† The negative ΔH values signified the exothermic nature of adsorption of CBs with HA–M. It is also confirmed by the decrease of the Kd value with the rise of temperature. Therefore, the HA–M exhibits lower adsorption capacity at higher temperature. Furthermore, the adsorption process of CBs with HA–M is achieved by hydrophobic interactions formed between CBs and HA–M,35 which is in accord with the previous results. The negative ΔG values have proved the feasibility and the spontaneous nature of the adsorption process.36
Influence of coexisting organics on the adsorption capacity
Various organics usually coexist in soil washing wastewater. Therefore, typical organic matters (benzene and trichloroethylene) were utilized as the coexisting organics to investigate their influences on the adsorption of CBs on HA–M. It is worthwhile to note that the coexisting organic matters also have negligible influence on the adsorption of CBs on HA–M (Table S5†). These results, contributed unambiguously by the super-hydrophobicity of HA–M, indicate the potential application of HA–M for adsorptive removal of uncharged CBs in practical aqueous solutions.
Adsorption efficiency in multi-component aqueous solutions
In practical wastewater, different types of CBs usually coexist. Hence, the adsorption performance of HA–M was investigated in aqueous solutions containing six typical CBs at different concentrations. As shown in Fig. 4, the HA–M exhibits satisfied removal efficiency (>90%) to all kinds of CBs both at low and high concentrations. In addition, the removal efficiencies of these CBs are slightly reduced compared to the removal efficiency in signal component solution, which suggest that a mild competitive adsorption among the CBs. However, the influence of the mild competitive adsorption can be negligible compared with superior high removal efficiency.
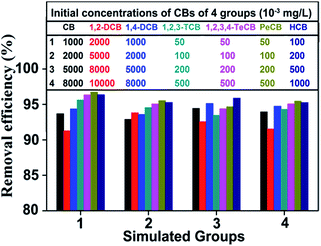 |
| Fig. 4 Adsorption efficiency of CBs on HA–M in multi-component aqueous solutions. | |
Dynamic adsorption experiment
Two typical CBs, 1,2,3,4-TeCB and 1,4-DCB, were utilized in column study to the dynamic adsorption behaviors of HA–M to CBs. The concentration of each CBs was fixed at 0.04 mg L−1 or 0.06 mg L−1 to simulate the practical aqueous solutions in environment. When the concentration was fixed at 0.04 mg L−1, the treated volume of solution was high up to 1.4 L at the breakthrough point (Fig. 5a). Moreover, the concentration of both CBs reached to the initial concentration quickly, which suggests a high treatment capacity of HA–M column. When adjusting the concentrations of 1,2,3,4-TeCB/1,4-DCB to 0.06 mg L−1 (Fig. 5b), the treated volume at breakthrough point reduced to 1.2 L, and the column efficiency was still satisfied.
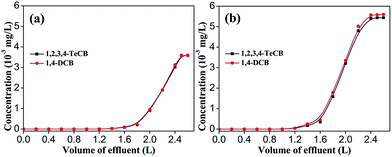 |
| Fig. 5 Breakthrough curves of CBs on HA–M; bed depth ≈ 10.0 cm, flow rate: 0.8 mL min−1, contact time: 54 h. (a) Influent concentration: 0.04 mg L−1, (b) influent concentration: 0.06 mg L−1. | |
Adsorption of conventionally pesticides studies
Based on the high adsorptive removal efficiency of HA–M to CBs, typical organochlorine pesticides (OCPs), such as α-HCH, p′p-DDT, were used to evaluate the removal performance of HA–M to these toxic compounds widely used. As expected, HA–M exhibits high adsorption capacity to 4 OCPs in aqueous solution under the optimized pH and temperature (Fig. S8†). The adsorption capacities of HA–M to the 4 OCPs with low concentrations (0.02 mg L−1) are all up to 9 mg g−1 in a wide range of pH and temperature. The kinetic data can also be well described by the pseudo-second-order kinetic model (R2 > 0.99) (Fig. S9 and Table S6†), indicating that the adsorption process is familiar to those of CBs on HA–M. The HA–M also exhibits high removal efficiency (>90%) to the 4 OCPs in simulated solutions (Fig. S9†). Furthermore, as an important issue for absorbent in practical application, the recyclability of HA–M in adsorption of aldrin was investigated. As shown in Fig. 6b, the HA–M can be recycled without significant loss decrease of removal efficiency, exhibiting satisfied recyclability.
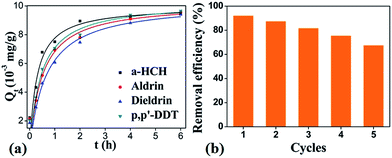 |
| Fig. 6 Adsorption results of OCPs on HA–M. (a) Pseudo-second-order model fitting, (b) recyclability of HA–M for the adsorptive removal of aldrin. | |
Experimental
Materials and methods
Humic acid (HA, CAS: 1415-93-6) was purchased from Tianjin Guangfu Fine Chemical Research Institute co., Ltd (China) and used after passing a 100 mesh sieve. Monoolein (CAS: 111-03-5), sulfuric acid (CAS: 7664-93-9) and hexane (CAS: 110-54-3) were of analytical grade (ChengDu Kelong Chemical Co., Ltd, China). 12 kinds of chlorobenzene compounds (CBs) were purchased from Aladdin Industrial Corporation, which includes, chlorobenzene (CB), 1,2-, 1,3-, 1,4-dichlorobenzene (DCB), 1,2,3-, 1,2,4-, 1,3,5-trichlorobenzene (TCB), 1,2,4,5-, 1,2,3,4-, 1,2,3,5-tetrachlorobenzene (TeCB), pentachlorobenzene (PeCB) and hexachlorobenzene (HCB). 4 types of organochlorine pesticides (OCPs) were obtained from Agro-Environmental Protection Institute, Ministry of Agriculture (China), including α-hexachlorocyclohexane (α-HCH), p,p′-dichlorodiphenyltrichloroethane (p,p′-DDT), aldrin and dieldrin, purity >99.5%. Physicochemical data of the CBs and OCPs are shown in Table S7.†
Preparation and characterization of HA–M
25.425 g of monoolein was mixed with 8.475 g of HA in 0.619 g of H2SO4. The resultant mixture was stirred at 120 °C for 24 h, allowing the chemical grafting of monoolein onto HA. After the reaction, the mixture was cooled down to room temperature, followed by centrifugation, washing and vacuum drying. The obtained HA–M was ground and screened with 100 meshes for further adsorption experiments.
Batch adsorption experiments
Various CBs solutions used in this experiment were diluted by deionized water from the stock solutions prepared by CH3OH. Moreover, the adsorption system was kept sealed during all batch experiments.
pH influences on the adsorption capacity. 0.2 g of HA–M was added into 100 mL of CBs solutions (50 mg L−1 of CBs). The solution pH was adjusted in the range of 3–9 using HCl solutions (1.0 M L−1) and NaOH solutions (1.0 M L−1). The mixture solution was shaken at 25 °C for 12 h to achieve the adsorption progress. When the adsorption was completed, the mixture solution was filtrated. The collected filtrate was extracted by hexane for 3 times, then 5 g NaCl was added into the collected hexane to increase the ionic strength of organic matters. Subsequently, the collected hexane was dehydrated using anhydrous sodium sulfate and then concentrated to 2 mL via rotary evaporation. The obtained solutions was further filtrated by passing an organic membrane (0.45 μm), the concentrations of CBs or OCPs in these filtrates were analyzed by gas chromatograph (GC, D7900, Teccomp Ltd, China). The adsorption capacities of HA–M (Qe, mg g−1) was calculated by eqn (3): |
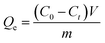 | (3) |
where C0 (mg L−1) and Ct (mg L−1) are the initial concentrations of CBs (or OCPs) and the remaining concentration at time t, respectively, V (L) is the volume of aqueous solution, and m (g) is the mass of HA–M used.
Adsorption kinetics. 0.2 g of HA–M was added into 100 mL of solutions (50 mg L−1 of CBs, or 0.02 mg L−1 of OCPs). The solution pH was adjusted to 7.0, while the adsorption process was conducted at 25 °C with constant shaking, promising that lipohilic HA–M disperse well in the heterogeneous adsorption system formed by CBs and HA–M. The concentration of CBs (or OCPs) in the extracted phase was measured at intervals by GC, and the adsorption capacities at time t (min) were obtained by mass balance calculation, which were denoted as Qt (mg g−1). The adsorption kinetic data was further fitted by the pseudo-first-order rate model,37 the pseudo-second-order rate model,38 and the intraparticle diffusion model,39 which are expressed as eqn (4)–(6): |
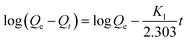 | (4) |
|
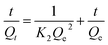 | (5) |
where Qe (mg g−1) and Qt (mg g−1) are the adsorption amount of CBs and OCPs at equilibrium and time t (h), respectively; K1 (h−1) and K2 (g mg−1 h−1) are the rate constant, K3 (mg g−1 h0.5) is the intraparticle diffusion rate constant.
Adsorption isotherms. Adsorption isothermal experiments were conducted with the initial concentration of CBs from 1 to 325 mg L−1. The solution pH was adjusted to 7.0 and the adsorption was carried out at 25 °C for 12 h. The concentration of CBs in the extracted phase was measured by GC. The adsorption capacities at equilibrium (Qe) were obtained by mass balance calculation. Adsorption isotherms data were further fitted by Langmuir40 and Freundlich41 isothermal equations. The Langmuir eqn (7) and Freundlich eqn (8) are expressed as follows: |
Qe = QmaxK4Ce/(1 + K4Ce)
| (7) |
Effect of coexisting organics. Benzene and trichloroethylene were utilized as coexisting organics to investigated the removal efficiency of HA–M to CBs. Batch absorption experiments were carried out by adding 0.2 g of HA–M into 100 mL solutions of 1,3,5-TCB, respectively. The concentrations of each kind of organics were fixed at 0.5 g L−1 or 1.0 g L−1. The initial pH of the solutions was adjusted 7.0 and adsorption time was 12 h. The concentrations of 1,3,5-TCB in the solutions was analyzed by GC.
Adsorption efficiency in multi-component aqueous solutions. 0.2 g of HA–M was added into a series of 100 mL aqueous solutions, which containing CBs and OCPs with different concentrations. The initial pH of the solutions was adjusted to 7.0 and the adsorption was carried out under constant stirring for 12 h. After adsorption, the remaining CBs and OCPs concentrations were determined by GC.
Dynamic adsorption experiment. 1.0 g of HA–M was mixed with 8.0 g of quartz sand, and the resultant mixture was packed into a column with the length of height of 10 cm and the diameter of 1.0 cm. During the dynamic adsorption process, 1,2-DCB/1,2,3,4-TeCB mixed solutions with two different concentration (0.04 mg L−1 and 0.06 mg L−1) was pumped through the column at the flow rate of 0.8 mL min−1, respectively. The initial pH value was fixed at 7.0. The residual CBs in the solution extracted by hexane and analyzed by GC.
Regeneration of HA–M. Regeneration of HA–M was performed in desorption solution of methylene chloride. 0.2 g of HA–M, which has used to absorb the conventionally pesticide (aldrin, 0.05 mg L−1, 100 mL), was eluted in 20 mL methylene chloride with shaking at 25 °C for 1 h. Then, the adsorbent was reused for the adsorption of aldrin.
Conclusions
In this study, natural humic acid was utilized as raw material to prepared hydrophobic adsorbent (HA–M) by modification with monoolein. The HA–M exhibited high efficiency in adsorptive removal of CBs and OCPs at certain concentration in aqueous solution. Super-hydrophobic interaction was dominated in the adsorption behaviors of HA–M to CBs and OCPs, which was not influenced by pH value, temperature or coexisting organics. More importantly, the low-cost HA–M can be recycled 5 times for the adsorption of OCPs. Therefore, the HA–M shows great potential as recyclable adsorbent for effective removal of CBs and OCPs in aqueous solutions. This provides valuable information for the implication of adsorption removal CBs from soil washing wastewater.
Acknowledgements
We acknowledge the National Natural Science Foundation of China (21506134, 51173122, 21406147, 51641209).
References
- J. Pernak, B. Markiewicz, A. Zgoła-Grześkowiak, Ł. Chrzanowski, R. Gwiazdowski, K. Marcinkowska and T. Praczyk, RSC Adv., 2014, 4, 39751–39754 RSC.
- P. Chakraborty, G. Zhang, J. Li, A. Sivakumar and K. C. Jones, Environ. Pollut., 2015, 204, 74–80 CrossRef CAS PubMed.
- Z. Kurt and J. C. Spain, Environ. Sci. Technol., 2013, 47, 6846–6854 CAS.
- E. J. Kim, Y.-M. Park, J.-E. Park and J.-g. Kim, Sci. Total Environ., 2014, 476–477, 327–335 CrossRef CAS PubMed.
- J. Michałowicz, K. Mokra, K. Rosiak, P. Sicińska and B. Bukowska, Environ. Toxicol. Pharmacol., 2013, 36, 979–988 CrossRef PubMed.
- W.-f. Chen, L. Pan, L.-f. Chen, Q. Wang and C.-c. Yan, RSC Adv., 2014, 4, 46689–46696 RSC.
- Y. Li, X. Hu, X. Song, Y. Hou and Q. Guo, RSC Adv., 2015, 5, 75033–75043 RSC.
- U. Stoin, A. Mojon and Y. Sasson, RSC Adv., 2015, 5, 6571–6577 RSC.
- G. Santacruz, E. R. Bandala and L. G. Torres, J. Environ. Sci. Health, Part B, 2005, 40, 571–583 CrossRef PubMed.
- D. Fabbri, A. B. Prevot, V. Zelano, M. Ginepro and E. Pramauro, Chemosphere, 2008, 71, 59–65 CrossRef CAS PubMed.
- X. Zou, J. E. Fallah, J.-M. Goupil, G. Zhu, V. Valtchev and S. Mintova, RSC Adv., 2012, 2, 3115–3122 RSC.
- S. Tandy, A. Ammann, R. Schulin and B. Nowack, Environ. Pollut., 2006, 142, 191–199 CrossRef CAS PubMed.
- S. Lladó, S. Covino, A. M. Solanas, M. Viñas, M. Petruccioli and A. D’annibale, J. Hazard. Mater., 2013, 248–249, 407–414 CrossRef PubMed.
- J. M. Buth, M. R. Ross, M. N. Kristopher and W. A. Arnold, Chemosphere, 2011, 85, 284–289 CrossRef CAS PubMed.
- P. Chowdhury, J. Moreira, H. Gomaa and A. K. Ray, Ind. Eng. Chem. Res., 2012, 51, 4523–4532 CrossRef CAS.
- K.-J. Choi, S.-G. Kim and S.-H. Kim, J. Hazard. Mater., 2008, 151, 38–43 CrossRef CAS PubMed.
- Z. Huang and H. K. Lee, J. Chromatogr. A, 2015, 1401, 9–16 CrossRef CAS PubMed.
- X. D. Liu, Y. Murayama, M. Matsunaga, M. Nomizu and N. Nishi, Int. J. Biol. Macromol., 2005, 35, 193–199 CrossRef CAS PubMed.
- Y. Lu, Z. Wang and J. Huckins, Aquat. Toxicol., 2002, 60, 139–153 CrossRef CAS PubMed.
- J. D. Petty, C. E. Orazio, J. N. Huckins, R. W. Gale, J. A. Lebo, J. C. Meadows, K. R. Echols and W. L. Cranor, J. Chromatogr. A, 2000, 879, 83–95 CrossRef CAS PubMed.
- Y. Xu, Z. Wang, R. Ke and S. U. Khan, Environ. Sci. Technol., 2005, 39, 1152–1157 CrossRef CAS PubMed.
- N. Meems, C. E. W. Steinberg and C. Wiegand, Sci. Total Environ., 2004, 319, 123–136 CrossRef CAS PubMed.
- S. Yang, J. Hu, C. Chen, D. Shao and X. Wang, Environ. Sci. Technol., 2011, 45, 3621–3627 CrossRef CAS PubMed.
- L. Mansuy, Y. Bourezgui, E. Garnier-Zarli, E. Jardé and V. Réveillé, Org. Geochem., 2001, 32, 223–231 CrossRef CAS.
- D. Xu, S. Zhu, H. Chen and F. Li, Colloids Surf., A, 2006, 276, 1–7 CrossRef CAS.
- I. Christal, H. Knicker, I. Kögel-Knabner and R. Kretzschmar, Eur. J. Soil Sci., 2000, 51, 617–625 CrossRef.
- H. Wang, J. Zhu, Q.-L. Fu, J.-W. Xiong, C. Hong, H.-Q. Hu and A. Violante, Pedosphere, 2015, 25, 405–414 CrossRef.
- J. Du, X. Lai, N. Yang, J. Zhai, D. Kisailus, F. Su, D. Wang and L. Jiang, ACS Nano, 2011, 5, 590–596 CrossRef CAS PubMed.
- X. Huang, Y. Wang, X. Liao and B. Shi, J. Hazard. Mater., 2010, 183, 793–798 CrossRef CAS PubMed.
- F. Haghseresht and G. Q. Lu, Energy Fuels, 1998, 12, 1100–1107 CrossRef CAS.
- R. Sennour, G. Mimane, A. Benghalem and S. Taleb, Appl. Clay Sci., 2009, 43, 503–506 CrossRef CAS.
- Z. Pei, L. Li, L. Sun, S. Zhang, X.-q. Shan, S. Yang and B. Wen, Carbon, 2013, 51, 156–163 CrossRef CAS.
- H. R. Nodeh and H. Sereshti, RSC Adv., 2016, 6, 89953–89965 RSC.
- G. Cui, M. Liu, Y. Chen, W. Zhang and J. Zhao, Carbohydr. Polym., 2016, 154, 40–47 CrossRef CAS PubMed.
- T. E. M. T. Hulscher and G. Cornelissen, Chemosphere, 1996, 32, 609–629 CrossRef.
- M. Kilic, E. Apaydin-Varol and A. E. Pütün, J. Hazard. Mater., 2011, 189, 397–403 CrossRef CAS PubMed.
- C. W. Hoogendam, A. d. Keizer, M. A. C. Stuart and B. H. Bijsterbosch, Langmuir, 1998, 14, 3825–3839 CrossRef CAS.
- Y. Liu and L. Shen, Langmuir, 2008, 24, 11625–11630 CrossRef CAS PubMed.
- X. Sun, X. Huang, X. P. Liao and B. Shi, J. Hazard. Mater., 2010, 179, 295–302 CrossRef CAS PubMed.
- I. Langmuir, J. Am. Chem. Soc., 1916, 38, 2221–2295 CrossRef CAS.
- P. Tan, J. Sun, Y. Hu, Z. Fang, Q. Bi, Y. Chen and J. Cheng, J. Hazard. Mater., 2015, 297, 251–260 CrossRef CAS PubMed.
Footnotes |
† Electronic supplementary information (ESI) available. See DOI: 10.1039/c6ra17949e |
‡ These authors contributed equally to this work. |
|
This journal is © The Royal Society of Chemistry 2017 |
Click here to see how this site uses Cookies. View our privacy policy here.