DOI:
10.1039/C6RA24954J
(Paper)
RSC Adv., 2017,
7, 13244-13249
Synthesis of quaternary phosphonium N-chloramine biocides for antimicrobial applications†
Received
10th October 2016
, Accepted 21st February 2017
First published on 27th February 2017
Abstract
The recently developed quaternary ammonium (QA) N-chloramine has been proved to be a promising “composite” biocide with drastically boosted antibacterial efficacy afforded by the QA moiety. In this work, a series of quaternary phosphonium (QP) N-chloramine biocides, covalently combining an N-chloramine moiety and a QP moiety via varied aliphatic methylene units, were synthesized by means of multi-step chemical reactions. Preliminary antibacterial tests against both Escherichia coli (E. coli) and Staphylococcus aureus (S. aureus) showed that the synthetic QP N-chloramine exhibited distinctively higher biocidal efficacy than the QA counterpart. Furthermore, bactericidal tests also indicated that QP N-chloramine with a linker of –(CH2)12– showed the highest biocidal efficacy, suggesting synergistic action between the N-chloramine moiety and QP moiety.
1 Introduction
Organic N-chloramine, defined as synthetic compounds that contain one or multiple N–Cl bonds, is now recognized as one of the most powerful biocidal agents due to its numerous advantages such as outstanding efficacy, low toxicity, low cost, facial regenerability upon bleaching treatment and substantial stability under aqueous and dry conditions.1,2 It is believed that the N-chloramine exhibits its biocidal function by transferring the oxidative chlorine to bacterial cellular receptors,2–4 thus resulting in a highly efficacious inactivating mode. Till now, biocides based on organic N-chloramines have been extensively applied in portable water disinfection and pathogenic bacteria inactivation in hospital and public venues,1,2 and many focus have been directed to the development of novel organic N-chloramine compounds1,4–6 and polymeric N-chloramine antibacterial materials7–15 in past decades.
Nevertheless, the aqueous solubility of organic N-chloramines usually become pretty poor once the N–Cl bond is produced form N–H bond, which is quite disadvantageous to its contact killing manner.5,9,16 Recently, Li et al.5 developed a new type of N-chloramine biocide 1 (Fig. 1) which contains a QA unit and a N-chloramine unit based on 5,5-dimethyl-hydantoin (DMH) structure. The introduced QA moiety, which undoubtedly improved the aqueous solubility, was found to greatly boost antibacterial efficacy of N-chloramine moiety both against multi-drug-resistant Escherichia coli (MDRE. coli) and methicillin-resistant Staphylococcus aureus (MRSA). The faster killing action was proposed that QA moiety probably facilitates the biocide–bacterium contact process via electrostatic interactions between the positive charged 1 and the negatively charged bacterial cell, and consequently promotes the transfer of oxidative chlorine (Cl+) to bacterial cellular receptors. Liang et al.9 prepared crossed linked quaternarized N-chloramine antimicrobial resins with improved surface hydrophilicity together with powerful antimicrobial properties. In the same lab, a quaternarized N-chloramine precursor was also prepared and successfully grafted to cotton surface without using organic solvents.16
 |
| Fig. 1 Structure of QA N-chloramine 1 and QP N-chloramines designed in this work. | |
Quaternary phosphonium (QP) compounds possess similar chemical structure with QA salts, and usually exert antibacterial capabilities once a long alkyl chain (C8–C18) is covalently tethered to the positive charge center.17–20 Compared with the nitrogen atom of QA salt, the phosphorus atom of QP salt has larger atomic radius and lower electronegativity.21 Accordingly, as compared to QA disinfectants, QP compounds usually exhibit much higher antimicrobial activity in that QP salts are capable of been more readily adsorbed on negatively charged bacterial cell wall due to the stronger polarization effect of phosphorus atom.18–20 To this end, we hypothesized that integration of QP moiety and DMH-based N-chloramine moiety would probably produce even higher antibacterial efficacies.
In this work, we described convenient synthesis of QP N-chloramines 3–6 (Fig. 1), which contain QP moiety and N-chloramine moiety covalently linked by aliphatic methylene chains with different length. The structure of these ionic N-chloramines and corresponding N-chloramine precursors was characterized by NMR and HRMS analysis. Given 1 as control, antibacterial performance of the QP N-chloramine biocide 3 was evaluated. The effect of methylene chain linker length on antibacterial activity was also investigated, since the designed QP N-chloramines could also been regarded as conventional QP salts bearing a alkyl chain terminated with a chlorinated DMH ring structure.
2 Experiments
2.1 Reagents and materials
Reagents and chemicals used in this research were purchased from commercial suppliers (reagent grade or higher) and used directly unless otherwise noted. Purification of all products and intermediates were conducted by flash column chromatography. Silica gel received from Qingdao Haiyang Chemical Plant, China, and TLC plates were visualized by means of iodine fumigation.
DMH-bromide 7, QA N-chloramine 1 and its precursor 2 were prepared using our reported procedure, respectively.5,22 t-Butyl hypochlorite, the chlorination agent used in this work to produce N-chloramines, was synthesized as described.5,6,23 The obtained NMR data of all this published compounds were identical with those found in previous literatures.
NMR spectra were recorded at room temperature on Bruker DRX 500 instrument. Accurate mass measurements were performed using a Q-TOF Micro mass spectrometry (Manchester, UK) equipped with Z-spray ionization source. All the HRMS data of ionic compounds came to the positive mode.
2.2 Chemistry
General synthesis of DMH-(CH2)n-Br (DMH-bromide 8–10). To a solution of DMH (3.10 g, 24.4 mmol) in acetone (250 mL) was added potassium carbonate (12.0 g, 87.2 mmol, 3.6 equiv.), and then the mixture was heated to reflux for 0.5 h. Afterward, dibromoalkanes (81.3 mmol, 3.6 equiv.) was added to the suspension, and the mixture was refluxed overnight. The inorganic salts was filtered and the filtrate was concentrated under reduced pressure to give the crude product. Further purification was performed on chromatography column with petroleum ether/ethyl acetate (1
:
1, v/v) to afford the DMH-bromides as white solid.
Hydantoin-bromide 8 (6.23 g, 87.7%). 1H NMR (CDCl3, 500 MHz) δ 3.49 (t, J = 6.5 Hz, 2H), 3.40 (t, J = 6.5, 2H), 1.81–1.91 (m, 2H), 1.58–1.68 (m, 2H), 1.40–1.52 (m, 2H), 1.44 (s, 6H), 1.28–1.37 (m, 2H); 13C NMR (CDCl3, 125 MHz) δ 177.4, 156.6, 58.7, 38.4, 33.8, 32.6, 27.9, 27.7, 25.8, 25.1; HRMS m/z: [M + H]+ calcd for C11H20BrN2O2, 291.0703; found: 291.0700.
Hydantoin-bromide 9 (7.21 g, 92.4%). 1H NMR (CDCl3, 500 MHz) δ 3.48 (t, J = 7.0 Hz, 2H), 3.40 (t, J = 6.5 Hz, 2H), 1.80–1.90 (m, 2H), 1.57–1.66 (m, 2H), 1.39–1.50 (m, 2H), 1.44 (s, 6H), 1.25–1.36 (m, 6H); 13C NMR (CDCl3, 125 MHz) δ 177.4, 156.7, 58.64, 38.5, 34.1, 32.7, 28.9, 28.6, 28.1, 28.0, 26.5, 25.1; HRMS m/z: [M + H]+ calcd for C13H24BrN2O2, 319.1016; found: 319.1013.
Hydantoin-bromide 10 (6.50 g, 71.1%). 1H NMR (CDCl3, 500 MHz) δ 3.48 (t, J = 7.25 Hz, 2H), 3.41 (t, J = 6.75 Hz, 2H), 1.80–1.90 (m, 2H), 1.57–1.65 (m, 2H), 1.37–1.47 (m, 2H), 1.43 (s, 6H), 1.23–1.33 (m, 14H); 13C NMR (CDCl3, 125 MHz) δ 177.4, 156.7, 58.6, 38.6, 34.2, 32.8, 29.5, 29.5, 29.4, 29.2, 28.8, 28.2, 28.1, 26.6, 25.1; HRMS m/z: [M + H]+ calcd for C17H32BrN2O2, 375.1642; found: 375.1645.
General synthesis of QP-DMH 11–14. To a solution of DMH-bromide (9.6 mmol, 1.2 equiv.) in acetonitrile (50 mL) was added tributylphosphine (1.60 g, 2.01 mL, 8.01 mmol) under N2 atmosphere condition, and then the mixture was heated to gentle reflux for 24 h. The solvent was removed under reduced pressure to give the crude product. Further purification by chromatography with dichloromethane/methanol (10
:
1 to 5
:
1, v/v) gave the phosphonium salt (Br− form) as white solid (3.41 g, 95.0%); the bromo form salt was dissolved in minimum DI water and passed through anion exchange resin (Amberlite® IRA-900, Cl−), and all the corresponding fractions were collected and concentrated to afford the final phosphonium salts of QP-DMH.
QP-DMH 11 (3.41 g, 95.0% yield based on tributylphosphine). 1H NMR (D2O, 500 MHz) δ 3.52 (t, J = 6.75 Hz, 2H), 2.04–2.14 (m, 8H), 1.76–1.84 (m, 2H), 1.30–1.48 (m, 12H), 1.33 (s, 6H), 0.82 (t, J = 7.25 Hz, 9H); 13C NMR (D2O, 125 MHz) δ 180.8, 157.2, 59.2, 38.6, 23.5, 23.3, 22.7, 19.7, 17.8, 15.7, 12.6; 31P NMR (202 MHz, D2O) δ 33.3; HRMS m/z: [M − Cl]+ calcd for C20H40N2O2P, 371.2822; found: 371.2818.
QP-DMH 12 (3.59 g, 94.2% yield based on tributylphosphine). 1H NMR (D2O, 500 MHz) δ 3.39 (t, J = 6.75 Hz, 2H), 2.01–2.10 (m, 8H), 1.32–1.55 (m, 18H), 1.31 (s, 6H), 1.19–1.25 (m, 2H), 0.82 (t, J = 7.25 Hz, 9H); 13C NMR (D2O, 125 MHz) δ 181.1, 157.8, 59.1, 38.3, 29.1, 26.7, 24.9, 23.5, 23.3, 22.7, 20.1, 17.9, 17.5, 12.6; 31P NMR (202 MHz, D2O) δ 33.3; HRMS m/z: [M − Cl]+ calcd for C23H46N2O2P, 413.3291; found: 413.3288.
QP-DMH 13 (3.90 g, 95.0% yield based on tributylphosphine). 1H NMR (D2O, 500 MHz) δ 3.39 (t, J = 7.0 Hz, 2H), 2.02–2.12 (m, 8H), 1.33–1.55 (m, 18H), 1.32 (s, 6H), 1.15–1.28 (m, 6H), 0.84 (t, J = 7.25 Hz, 9H); 13C NMR (D2O, 125 MHz) δ 181.0, 157.8, 59.0, 38.5, 29.6, 27.7, 27.6, 27.1, 25.7, 23.5, 23.3, 22.8, 20.5, 17.9, 17.5, 12.6; 31P NMR (202 MHz, D2O) δ 33.3; HRMS m/z: [M − Cl]+ calcd for C25H50N2O2P, 441.3604; found: 441.3618.
QP-DMH 14 (4.00 g, 86.2% yield based on tributylphosphine). 1H NMR (D2O, 500 MHz) δ 3.38 (t, J = 6.75 Hz, 2H), 2.01–2.11 (m, 8H), 1.12–1.54 (m, 32H), 1.31 (s, 6H), 0.83 (t, J = 7.25 Hz, 9H); 13C NMR (D2O, 125 MHz) δ 180.0, 157.1, 58.8, 38.3, 30.1, 29.2, 29.1, 28.8, 28.8, 28.3, 27.6, 26.3, 23.8, 23.4, 22.9, 20.9, 20.8, 18.1, 17.7, 12.7; 31P NMR (202 MHz, D2O) δ 33.2; HRMS m/z: [M − Cl]+ calcd for C29H58N2O2P, 497.4230; found: 497.4247.
General chlorination procedure to produce QP N-chloramine 3–6. To a solution of QP-DMH 11–14 (5.01 mmol) in 20 mL H2O/t-butanol (1/4) was added t-butyl hypochlorite (2.20 g, 2.30 mL, 19.8 mmol, 4.0 equiv.). The mixture was then sealed and stirred in dark for 24 h. After removing excess t-butyl hypochlorite and the solvent, the final QP N-chloramines were obtained in quantitative amount.
QP N-chloramine 3. 1H NMR (D2O, 500 MHz) δ 3.60 (t, J = 6.75 Hz, 2H), 2.05–2.14 (m, 8H), 1.77–1.87 (m, 2H), 1.27–1.49 (m, 12H), 1.40 (s, 6H), 0.82 (t, J = 7.25 Hz, 9H); 13C NMR (D2O, 125 MHz) δ 177.0, 155.6, 66.4, 39.8, 23.3, 22.7, 21.0, 19.7, 17.7, 15.7, 12.6; 31P NMR (202 MHz, D2O) δ 33.8; HRMS m/z: [M − Cl]+ calcd for C20H39ClN2O2P, 405.2432; found: 405.2440.
QP N-chloramine 4. 1H NMR (D2O, 500 MHz) δ 3.58 (t, J = 6.5 Hz, 2H), 3.09–3.19 (m, 8H), 1.93–2.02 (m, 2H), 1.48–1.62 (m, 6H), 1.41 (s, 6H), 1.20–1.39 (m, 6H), 0.85 (t, J = 7.5 Hz, 9H); 13C NMR (D2O, 125 MHz) δ 177.3, 156.1, 66.2, 39.5, 29.1, 26.6, 24.8, 23.3, 22.8, 21.0, 20.4, 17.9, 17.5, 12.6; 31P NMR (202 MHz, D2O) δ 33.3; HRMS m/z: [M − Cl]+ calcd for C23H45ClN2O2P, 447.2902; found: 447.2915.
QP N-chloramine 5. 1H NMR (D2O, 500 MHz) δ 3.47 (t, J = 7.0 Hz, 2H), 2.01–2.11 (m, 8H), 1.14–1.55 (m, 24H), 1.38 (s, 6H), 0.83 (t, J = 7.25 Hz, 9H); 13C NMR (D2O, 125 MHz) δ 177.3, 156.2, 66.2, 39.7, 29.6, 27.6, 26.9, 25.5, 23.4, 23.2, 22.8, 21.0, 20.5, 17.9, 17.5, 12.6; 31P NMR (202 MHz, D2O) δ 33.3; HRMS m/z: [M − Cl]+ calcd for C25H49ClN2O2P, 475.3215; found: 475.3212.
QP N-chloramine 6. 1H NMR (D2O, 500 MHz) δ 3.44 (t, J = 6.75 Hz, 2H), 2.05–2.17 (m, 8H), 1.12–1.56 (m, 32H), 1.37 (s, 6H), 0.85 (t, J = 7.25 Hz, 9H); 13C NMR (D2O, 125 MHz) δ 175.6, 155.0, 66.0, 39.5, 30.3, 29.7, 29.3, 29.3, 29.0, 28.5, 27.6, 26.3, 23.5, 23.0, 21.6, 21.1, 21.0, 18.2, 17.8, 12.9; 31P NMR (202 MHz, D2O) δ 33.2; HRMS m/z: [M − Cl]+ calcd for C29H57ClN2O2P, 531.3841; found: 531.3854.
2.3 Antibacterial test
We here used tryptone soya agar (TSA) medium for bacterial culture. Bacteria (E. coli ATCC 25922 and S. aureus ATCC 25923) from stocks were allowed to grow at 37 °C for 18–20 hours to give logarithmic-phase cultures. Antibacterial activity of our synthetic N-chloramines was conducted as followed. To 20 mL of bacterial suspension (106 to 107 colony forming units (CFU) mL−1) in a centrifuge tube was added 40 μL of N-chloramine (3–6) solutions (0.28 M stock solution, final 20 ppm [Cl+]) and timing of the exposure to the biocide was started immediately. After contact for determined intervals, 1.0 mL aliquots were withdrawn and mixed with an equal volume of 0.02 N sodium thiosulfate in phosphate buffer saline (PBS, 0.05 M, pH 7.0). The active chloramine was then quenched and the bacterial suspension was serially diluted. 100 μL of each diluted bacterial suspension was placed onto nutrient agar plates in triplicate. The same procedure was also applied to precursors of N-chloramines (11–14). After being incubated at 37 °C for 24 h, viable bacterial colonies on the plates were counted, and the bacterial reduction was then reported as followed:
Percentage reduction (%) = (A − B)/A × 100 |
where A is the number of bacteria retrieved from controls (colony-forming units, CFU mL−1), and B is the number of bacteria retrieved from N-chloramines or its precursors (CFU mL−1).
3 Results and discussion
3.1 Chemistry synthesis
In this study, we aimed to covalently combine the N-chloramine unit and the QP salt unit as shown in Scheme 1. Starting from commercial DMH as the raw material, DMH-bromide 7–10 was prepared via N-alkylation reaction on the imide site of DMH ring structure according to our previous procedures.5,22 Large excess dibromoalkane was herein used to minimize the bis-alkylated byproduct and the residual dibromoalkane was readily recycled either on chromatography or by distillation under reduced pressure. To produce phosphonium salts via quaternization reaction, triphenyl phosphine was initially adopted due to its commercial availability and relative stability. By reacting with as-prepared DMH-bromide 7, the corresponding phosphonium salt (Br− form) was successfully obtained (NMR data not shown) as white solid, but bears very poor solubility in water which is quite disadvantageous to the subsequent anion exchange procedure and the final model antibacterial test in aqueous solutions. As such, our attention was turned to tributyl phosphine, another commercial alternative which is quite sensitive to oxygen. The followed quaternization reaction with excess DMH-bromides was then carried out under N2 atmosphere condition. After work up and chromatography of the reaction mixture, high yield of DMH-QP salts 11–14 (Br− form) was achieved with residual DMH-bromides recycled. The obtained phosphonium salt was proved to bear pretty good aqueous solubility and was then treated with anion exchange resin to replace the Br− with Cl−, in case that Br− probably could be oxidized during the subsequent chlorination process, thus making the model antibacterial tests more complicated.
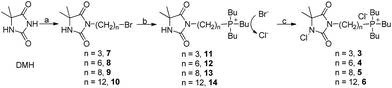 |
| Scheme 1 Synthesis route of QP N-chloramines. Reagents and conditions: (a) K2CO3, Br(CH2)nBr, acetone, reflux; (b) PBu3, CH3CN, N2, reflux; ion-exchange (Amberlite R IRA-900, Cl−) (c) t-BuOCl, H2O/t-BuOH, rt. | |
We next used synthetic t-butyl hypochlorite as the chlorination agent to produce QP N-chloramines 3–6. The reaction was conducted in dark at room temperature and the final products were obtained effortlessly after the solvent and excess t-butyl hypochlorite were removed under vacuum. It is worthwhile to note that we didn't adopted commercial bleach (NaClO) for this chlorination procedure because these QP N-chloramines, basically regarded as organic salts, also possess pretty good aqueous solubility and thus could not be readily purified from the reaction mixture which usually contains substantial amount of inorganic slats such as NaCl, NaOH and excess NaClO.
Taking the 1H NMR data of QP N-chloramine 5 and its precursor 13 for example, these two spectra were quite similar but some distinctive difference was still observed as shown in Fig. 2. After the chlorination reaction, signal of –CH3 of 13 shifted downfield from δ 1.31 to δ 1.41, and signal of –CH2– (adjacent to the imide group) of 13 also shifted downfield from δ 3.39 to δ 3.48 as well, probably attributed by the electron withdraw effect of the newly introduced Cl atom on DMH ring structure. Similar chemical shift difference was also observed for 13C NMR data analysis of compound 5 and compound 13 (Fig. S3†). The NMR chemical shift change together with the HRMS data clearly demonstrated the successful chlorination after being treated with t-butyl hypochlorite.
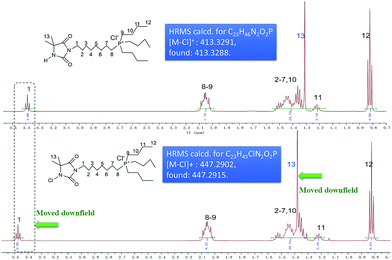 |
| Fig. 2 1H NMR spectra of QP N-chloramine 5 and its precursor 13. | |
3.2 Antibacterial performance
To examine the antibacterial activity of the synthetic QP N-chloramine 3, the ammonium counterpart 1 was prepared5 as a contrast. As shown in Table 1, the QA N-chloramine precursor 2, together with QP N-chloramine precursor 11, did not show any bactericidal activity, which is regarded as reasonable in that no long (usually C8–C18) alkyl chain was covalently tethered to these cationic centre.17,24,25 Within 5 min, QA N-chloramines 1 achieved 0.65 ± 0.04 and 0.09 ± 0.02 log bacterial reduction against E. coli and S. aureus respectively, whereas QP N-chloramine 3 displayed 1.10 ± 0 and 1.84 ± 0.01 log reduction accordingly. The observed bacterial reduction difference indicated that the presence of phosphonium positive charge moiety in biocide 3 contributes to a even faster N-chloramine–microorganism contact killing as compared to counterpart 1 which contains a QA positive charge unit instead.
Table 1 Bactericidal results of QA N-chloramine 1 and QP N-chloramine 3
Bacteria |
Synthetic compoundsc |
Contact time (min) |
5 |
10 |
Percent reduction/% |
Log reduction/log |
Percent reduction/% |
Log reduction/log |
The inoculum concentration: 2.9 × 106 CFU mL−1. The inoculum concentration: 6.8 × 106 CFU mL−1. The final [Cl+] concentration: 20 ppm for N-chloramines and 0 ppm for precursors. |
E. colia |
2 |
0 |
0 |
0 |
0 |
1 |
77.0 ± 1.7 |
0.65 ± 0.04 |
100 |
6.46 |
11 |
0 |
0 |
0 |
0 |
3 |
92.0 ± 0.0 |
1.10 ± 0.00 |
100 |
6.46 |
S. aureusb |
2 |
0 |
0 |
0 |
0 |
1 |
19.1 ± 4.4 |
0.09 ± 0.02 |
73.5 ± 2.9 |
0.58 ± 0.05 |
11 |
0 |
0 |
0 |
0 |
3 |
85.2 ± 0.1 |
1.84 ± 0.01 |
100 |
6.83 |
The obtained antibacterial data encouraged us to consider the possible action mode for the enhanced biocidal activity of QP N-chloramine 3. It's known that low molecular cationic disinfectants, either QP salts or QA salts, usually exert robust biocidal activity which relies on the tethered long alkyl chain (C8–C18) on the cation centre.24 Once the disinfectant is arrested on the negatively charged bacterial cell, the long aliphatic chain is capable of penetrating cell membrane, leading to disruption of the cytoskeleton and leakage of the cellular contents. Since no long alkyl chain exists in both QP N-chloramine 3 and QA N-chloramine 1, the antibacterial efficacy disparity between these two N-chloramine biocides could be merely attributed to the presence of different cationic centre. QP N-chloramine 3 displayed even higher biocidal efficacy than QA counterpart 1, implying that the phosphonium moiety probably bears even stronger affinity toward the negatively charged bacterial cell,18,19 especially toward cell membrane protein receptors and phosphate groups of membrane phospholipids (Fig. 3) via electrostatic interactions. To further understand the antibacterial difference between QP N-chloramine 3 and ammonium counterparts 1, the butyl substituted QA N-chloramine analog 15 was also prepared (Scheme S1 in ESI†). As a QA salt, compound 15 contains the same N-alkyl substituent and thus may be a more desirable contrast for antibacterial evaluation of 1 and 3. Not surprisingly, both 1 and 15 displayed the same level but inferior antibacterial activity (data not shown) as compared to that of QP N-chloramine 3, also suggesting that the faster killing mode of N-chloramine 3 was probably achieved by the stronger affinity of phosphonium moiety18,19 towards bacteria cell. However, the pinpoint chemical structure and molecule size of phosphonium moiety of 3 and ammonium moiety of 1 are not entirely identical due to the different atomic radius and different electronegativity of central atom (P vs. N), and the bactericidal mode based on prerequisite bacterium–biocide contact is essentially a complicated biochemical and biological process.2 Taken together, a preliminary conclusion could be at least made that the stronger electrostatic affinity of QP N-chloramine 3 (as depicted in Fig. 3) leads to the faster contact mode and the facilitated active Cl+ transfer probably to –SH (cysteine) and –NH2 (lysine) groups in protein receptors,26 resulting in higher bactericidal efficacy compared to QA N-chloramine 1.
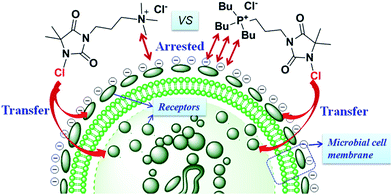 |
| Fig. 3 Proposed contact killing mode of QA N-chloramine 1 and QP N-chloramine 3. | |
We next moved to investigate the linker (–(CH2)n–) effect on bactericidal activity of QP N-chloramines. As shown in Table 2, no antibacterial activity was observed for QP N-chloramine precursors 11–13. It is also considered as reasonable because these compounds posses no long alkyl chains that could effectively penetrate bacteria cell membranes, thus exerting little antibacterial activity within our predetermined contact time frame.17,24 In contrast, another precursor 14 achieved 1.17 ± 0.15 and 0.96 ± 0.11 bacteria log reduction (within 5 min) for E. coli and S. aureus respectively, acting as a conventional QP salt biocide and thus displaying pretty good antibacterial activity. Obviously, the QP N-chloramine precursor 14, which carries a long alkyl chain terminated with a DMH group (–(CH2)12–DMH), probably exhibited similar antibacterial activity with conventional QP salts tethered with C12–C16 long aliphatic chain.24,25 It means that the long chain of –(CH2)12–DMH was also capable of inactivating microbial cell by penetrating cytoplasmic membrane. However, it is worthwhile to note that, for the purpose of simplifying the antibacterial system, no lecithin was added in our current study to quench long alkyl chains of QP salts. The contact killing time frame for QP salt 14 is thus not the determined interval of 5 min or 10 min, but the whole bacterial culturing process (around 20 h), suggesting slower killing kinetics than N-chloramines.
Table 2 Bactericidal results of synthetic QP N-chloramines 3–6
Bacteria |
QP N-chloramines (precursors)c |
Contact time (min) |
5 |
10 |
Percent reduction/% |
Log reduction/log |
Percent reduction/% |
Log reduction/log |
The inoculum concentration: 1.6 × 106 CFU mL−1. The inoculum concentration: 2.0 × 106 CFU mL−1. The final [Cl+] concentration: 20 ppm for N-chloramines and 0 ppm for precursors. |
E. colia |
11 |
0 |
0 |
0 |
0 |
3 |
95.9 ± 1.2 |
1.39 ± 0.01 |
100 |
6.22 |
12 |
0 |
0 |
0 |
0 |
4 |
92.1 ± 0. 6 |
1.11 ± 0.04 |
100 |
6.22 |
13 |
0 |
0 |
0 |
0 |
5 |
89.3 ± 1.2 |
0.97 ± 0.05 |
100 |
6.22 |
14 |
92.5 ± 2.4 |
1.17 ± 0.15 |
95.5 ± 0. 2 |
1.35 ± 0.01 |
6 |
99.3 ± 0.1 |
2.16 ± 0.02 |
100 |
6.22 |
S. aureusb |
11 |
0 |
0 |
0 |
0 |
3 |
99.1 ± 0.0 |
2.04 ± 0.00 |
100 |
7.30 |
12 |
0 |
0 |
0 |
0 |
4 |
81.0 ± 0.0 |
0.72 ± 0.00 |
100 |
7.30 |
13 |
0 |
0 |
0 |
0 |
5 |
76.0 ± 1.0 |
0.62 ± 0.02 |
100 |
7.30 |
14 |
88.8 ± 1.8 |
0.96 ± 0.11 |
93.8 ± 0.3 |
1.20 ± 0.02 |
6 |
100 |
7.30 |
100 |
7.30 |
An interesting antibacterial trend for QP N-chloramines was observed that the bacteria reduction declined with the methylene linker increased from –(CH2)3– (biocide 3) to –(CH2)8– (biocide 5), while the highest log reduction (2.17 for E. coli and 7.30 for S. aureus within 5 min) was achieved for biocide 6 with a linker of –(CH2)12– introduced. The data could be attributed that the QP N-chloramine biocide becomes more hydrophobic with the linker extended from –(CH2)3– to –(CH2)8–, probably providing slower diffusion and unfavored penetration into microbial cell wall. Such a finding is consistent with our previous study that decreased antibacterial activity was observed if a hexyl group (C6) was introduced to cationic centre of QA N-chloramines 1 to replace one methyl group.6 Even though the N-chloramine 6 is the most hydrophobic molecule, it could be essentially regarded as a conventional QP salt tethered with a long but DMH structure terminated chain (–(CH2)12–DMH-Cl), which probably produce similar bipolar dimmer as the long chained QA salt behaves27 and consequently provide additional contact killing contribution by effective penetration across microbial cell membrane. Among all the synthetic QP N-chloramines the biocide 6 exhibited the best antibacterial capacity, implying the synergistic action between the N-chloramine moiety and QP salt moiety. Ning et al.6 also reported the enhanced antibacterial activity of dodecyl/tetradecyl chained analogs of QA N-chloramines 1, and thoroughly discussed the synergistic effect between the N-chloramine unit and the long chained QA salt unit. Furthermore, compared with the QP salt moiety, the N-chloramine moiety is believed to play a predominant role during the contact killing process, but the modified long chain of biocide 6 undoubtedly provides an indispensable contribution. More antibacterial tests against drug-resistant strains and more chemical synthesis of QP N-chloramine 3 analogs for detailed synergistic effect investigation are under consideration in our group.
Another interesting data, as shown in Table 2, was also observed that total kill (at the contact interval of 10 min) was achieved either against E. coli or S. aureus for QP N-chloramines 3–6, which indicated that the bactericidal efficacy of these synthetic biocides could probably be underestimated without adequate contact time. This finding also suggests that the killing kinetic of QP N-chloramines may be altered by the linker length (–(CH2)n–) whereas the potential antibacterial capability was not basically compromised giving appropriate antibacterial duration. In addition, according to previous report, the “cut-off” effect28 for the QP N-chloramines would probably be observed if the methylene linker increases to –(CH2)14– or even longer. Preparation of such derivatives with longer connecting chains together with systematic antibacterial tests are also undertaken in this lab to investigate possible “cut-off” effect.
4 Conclusion
In conclusion, we successfully synthesized a new type of “composite” biocide with an QP positive charge unit and a DMH based N-chloramine unit combined covalently. Preliminary antibacterial tests indicated that the synthetic QP N-chloramine 3 exhibits drastically higher biocidal activity as compared to QA counterpart 1. The effect of methylene linker length in QP N-chloramines on bactericidal activity was also investigated to find that biocide 6 exhibited the highest biocidal activity, suggesting the synergistic effect between the QP moiety and N-chloramine moiety when a linker of –(CH2)12– was introduced to create the linear biocide molecule. These as-prepared QP N-chloramines are believed to be good candidates for battling infectious bacteria, especially for controlling biofilms29 that readily breed chronic infections. Furthermore, design and chemical synthesis of QP N-chloramine analogs that could readily be immobilized on polymeric substrates to prepare non-leaching antibacterial materials is undertaken in our lab, and such data will be delivered in due course.
Acknowledgements
The present work is supported by the Fundamental Research Funds for the Central Universities (DUT14RC(3)081), the Project Sponsored by the Scientific Research Foundation for the Returned Overseas Chinese Scholars, State Education Ministry (China), and the Chinese National Natural Science Foundation (21501018). The authors are also grateful for bacteria strain gift from Mrs Ning Anhong from Dalian Medical University.
References
- F. Hui and C. Debiemme-Chouvy, Biomacromolecules, 2013, 14, 585 CrossRef CAS PubMed.
- W. Gottardi, D. Debabov and M. Nagi, Antimicrob. Agents Chemother., 2013, 57, 1107 CrossRef CAS PubMed.
- A. Akdag, S. Okur, M. L. McKee and S. D. Worley, J. Chem. Theory Comput., 2006, 2, 879 CrossRef CAS PubMed.
- S. Lauten, H. Sarvis, W. Wheatley, D. Williams, E. Mora and S. Worley, Appl. Environ. Microbiol., 1992, 58, 1240 CAS.
- L. Li, T. Pu, G. Zhanel, N. Zhao, W. Ens and S. Liu, Adv. Healthcare Mater., 2012, 1, 609 CrossRef CAS PubMed.
- C. Ning, L. Li, S. Logsetty, S. Ghanbar, M. Guo, W. Ens and S. Liu, RSC Adv., 2015, 5, 93877 RSC.
- S. Liu and G. Sun, Ind. Eng. Chem. Res., 2009, 48, 613 CrossRef CAS.
- Y. Liu, K. Ma, R. Li and X. Ren, Cellulose, 2013, 20, 3123 CrossRef CAS.
- Z. Jie, X. Yan, L. Zhao, S. D. Worley and J. Liang, RSC Adv., 2014, 4, 6048 RSC.
- Y. Y. Sun and G. Sun, J. Appl. Polym. Sci., 2001, 81, 1517 CrossRef CAS.
- M. Natan, O. Gutman, R. Lavi, S. Margel and E. Banin, ACS Nano, 2015, 9, 1175 CrossRef CAS PubMed.
- G. Sun, W. B. Wheatley and S. D. Worley, Ind. Eng. Chem. Res., 1994, 33, 168 CrossRef CAS.
- C. Li, J. Hou, Z. Huang, T. Zhao, L. Xiao, G. Gao, C. Harnoode and A. Dong, Colloids Surf., B, 2015, 126, 106 CrossRef CAS PubMed.
- B. Demir, I. Cerkez, S. D. Worley, R. M. Broughton and T. S. Huang, ACS Appl. Mater. Interfaces, 2015, 7, 1752 CAS.
- Y. Liu, J. Li, X. Cheng, X. Ren and T. S. Huang, J. Mater. Chem. B, 2015, 3, 1446 RSC.
- Z. Z. Kang, B. Zhang, Y. C. Jiao, Q. Z. He and J. Liang, Cellulose, 2013, 20, 885 CrossRef CAS.
- A. Kanazawa, T. Ikeda and T. Endo, Antimicrob. Agents Chemother., 1994, 38, 945 CrossRef CAS PubMed.
- A. Muñoz-Bonilla and M. Fernández-García, Prog. Polym. Sci., 2012, 37, 281 CrossRef.
- Y. Xue and H. Xiao, J. Biomed. Mater. Res., Part A, 2016, 104, 747 CrossRef CAS PubMed.
- Y. Xue, Y. Pan, H. Xiao and Y. Zhao, RSC Adv., 2014, 4, 46887 RSC.
- L. C. Allen, J. Am. Chem. Soc., 1989, 111, 9003 CrossRef CAS.
- L. Li, N. Zhao and S. Liu, Polymer, 2012, 53, 67 CrossRef CAS.
- M. J. Mintz and C. Walling, Org. Synth., 1969, 49, 9 CrossRef CAS.
- P. Gilbert and A. Al-Taae, Lett. Appl. Microbiol., 1985, 1, 101 CrossRef CAS.
- P. Gilbert and L. E. Moore, J. Appl. Microbiol., 2005, 99, 703 CrossRef CAS PubMed.
- S. P. Denyer and G. Stewart, Int. Biodeterior. Biodegrad., 1998, 41, 261 CrossRef CAS.
- W. Gottardi and M. Nagl, J. Antimicrob. Chemother., 2005, 55, 475 CrossRef CAS PubMed.
- L. Caillier, E. Taffin-Givenchy, R. Levy, Y. Vandenberghe, S. Géribaldi and F. Guittard, Eur. J. Med. Chem., 2009, 44, 3201 CrossRef CAS PubMed.
- D. Karadag, O. E. Koroglu and B. Ozkaya, Process Biochem., 2015, 50, 262 CrossRef CAS.
Footnote |
† Electronic supplementary information (ESI) available. See DOI: 10.1039/c6ra24954j |
|
This journal is © The Royal Society of Chemistry 2017 |
Click here to see how this site uses Cookies. View our privacy policy here.