DOI:
10.1039/C6RA27339D
(Paper)
RSC Adv., 2017,
7, 9833-9839
A fluorescent chemosensor for Sn2+ and Cu2+ based on a carbazole-containing diarylethene†
Received
25th November 2016
, Accepted 13th January 2017
First published on 2nd February 2017
Abstract
A novel diarylethene with a carbazole moiety was developed for the highly selective and sensitive detection of Sn2+ and Cu2+ in methanol. Its fluorescence was enhanced with a 76 nm blue shift and selective fluorescence quenching occurred upon the addition of Sn2+ and Cu2+, respectively, over a wide range of tested cations. The compound formed complexes with Sn2+ and Cu2+ in a 1
:
1 stoichiometry and has the detection limits of 1.9 μM and 1.2 μM, respectively. Moreover, the compound can be applied to detect Sn2+ and Cu2+ in natural water samples with high accuracy.
Introduction
Fluorescent sensor design is an active field of supramolecular chemistry due to potential practical advantages in biomedical, analytical, and environmental chemistry,1 and in the construction of optical and electronic signaling channels towards targeted molecular or ionic species.2–4 In the last few decades, significant efforts have been made to develop novel fluorescent chemosensors, capable of distinguishing and sensing biologically and environmentally significant cations and anions.5,6 Among these, the detection of heavy and transition metal ions is significant due to these ions playing crucial roles in living systems and also simultaneously having tremendous detrimental effects when exceeding the normal permissible limits.7
Sn2+, an essential trace mineral for humans, is involved in growth factors and cancer prevention.8 Deficiency of tin may result in poor growth and hearing loss, whereas excess tin accumulation can abhorrently affect respiratory and digestive systems.9,10 Recent reports have revealed that Sn2+ can be readily taken up by human white blood cells and cause DNA damage.11 Thus, it is of great importance to establish a method for the determination of Sn2+ in the environmental and biological systems. Similarly, Cu2+ is also of significant importance as it acts as a catalytic cofactor for a variety of metalloenzymes12 and is an essential trace element for many biological processes and systems.13,14 The over-accumulation of Cu2+ in humans is responsible for many neurodegenerative diseases such as Menkes syndrome,15,16 Wilson's disease,17 Alzheimer's disease, and prion disease.18 Therefore, enormous attempts have been dedicated to the development of Cu2+ fluorescent sensors since they facilitate sensitive, easy, and rapid detection.19
As one of the most promising photoresponsive materials, diarylethenes have been well-recognized for their remarkable fatigue resistance, excellent thermal stability, and rapid response.20 Upon alternating the irradiation between UV and visible light, a reversible transformation between the open-ring and closed-ring isomers occurred, accompanied by many obvious changes in the physical properties such as absorption spectra, fluorescence, refractive indices, electronic conduction, oxidation-reduction potentials, and so on. It is very convenient to construct a molecular switch using diarylethenes. Diarylethene derivatives that exhibit good fluorescence performance have become star molecules due to their fluorescence signalling, high detection sensitivity, and simplicity.21,22 However, most diarylethenes show weak or no fluorescence.23 Thus, linking a suitable fluorescent chromophore into the diarylethene structure offers new possibilities for developing sensors.24 To date, many fluorescent sensors for Zn2+,25,26 Cu2+,27,28 Fe3+,29 Al3+,30,31 and Hg2+
32,33 based on diarylethene derivatives have been widely studied. However, there are relatively few reports on the fluorescent sensors for Sn2+.34,35
Carbazole possesses a rigid fused-ring structure and its N atom has a lone pair of electrons forming n–π conjugate with benzene rings. It has been widely investigated as a donor due to its potential value for solar energy storage and efficient luminescence.36 However, the carbazole moiety has rarely been used in diarylethene derivatives.37,38
Herein, a novel carbazole-containing diarylethene 1-(2-methyl-5-phenyl-3-thienyl)-2-[2-methyl-5-(3-amine-9-ethyl-carbazolyl)-3-thienyl]perfluorocyclopentene (1o) was successfully synthesized. 1o showed dual channel fluorescence signals (‘turn-on’ and ‘turn-off’) for the selective detection of Sn2+ and Cu2+ in methanol. To the best of our knowledge, this is a new chemosensor for multi-cations (Sn2+ and Cu2+) with discrimination in the fluorescence response. Considering the open- and closed-ring isomeric states, the photochromism and ion sensing of the six states was studied to clarify the mechanism of recognition, as shown in Scheme 1.
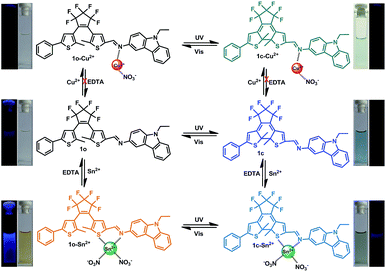 |
| Scheme 1 Photochromism and ion sensing process of 1o. | |
Experimental
Materials and chemicals
Reactions were monitored by analytical thin-layer chromatography on the plates coated with 0.25 mm silica gel 60 F254 (Qingdao Haiyang Chemical). Flash column chromatography employed silica gel (32–63 μm, Qingdao Haiyang Chemical) and Al2O3 (37–74 μm, J&K). Melting points were measured using a WRS-1B melting point apparatus and are uncorrected. Infrared spectra were obtained using a Bruker Vertex-70 spectrometer. NMR spectra were obtained using a Bruker AV-400 spectrometer with teramethylsilane (TMS) as the internal reference and CDCl3 and DMSO-d6 as solvents. Elemental analysis was carried out using a PE CHN 2400 analyzer. Absorption spectra were obtained using an Agilent 8453 UV/Vis spectrometer. Photoirradiation was carried out using an SHG-200 UV lamp, a CX-21 ultraviolet fluorescence analysis cabinet, and a BMH-250 visible lamp. Light of appropriate wavelengths was isolated by light filters.
Reagents
Chemical reagents were purchased from either Alfa or TCI and used without further purification. Solvents were purchased from Beijing Chemical Works. Anhydrous solvents were of spectro-grade and purified by distillation prior to use. All solution-phase reactions were performed under an atmosphere of dry argon or nitrogen.
Synthesis
The synthesis of 1o started with the preparation of the intermediates 2
39 and 3.40 3 was lithiated and coupled with 2 followed by hydrolysis to give 4, which was further coupled with 9-ethyl-9H-carbazol-3-amine to afford 1o. The synthetic route is shown in Scheme 2.
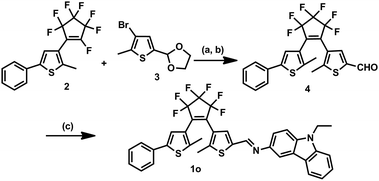 |
| Scheme 2 Synthesis of diarylethene 1o. Reagents and conditions: (a) n-BuLi, THF, 195 K; (b) p-TsOH, pyridine, acetone/H2O, reflux; and (c) 9-ethyl-9H-carbazol-3-amine, MeOH, reflux. | |
Synthesis of 4
To a stirred THF solution (50 mL) of 3 (2.04 g, 10 mmol), 2.5 mol L−1 n-BuLi/hexane solution (4.40 mL, 11 mmol) was slowly added at 195 K under a nitrogen atmosphere and the mixture was stirred for 30 min. 2 (2.93 g, 8 mmol) was added and the mixture was further stirred for 2 h at this temperature. The reaction was allowed to warm to room temperature and quenched by the addition of water. The product was extracted with diethyl ether and evaporated in vacuo. It did not require further purification and was used for the next step reaction. The crude product was dissolved in acetone/water (v/v = 4/1) solution (50 mL) with pyridine (0.79 g, 10 mmol) and p-toluenesulfonic acid (2.85 g, 15 mmol). The resulting mixture was refluxed for 6 h and then cooled down to room temperature. The mixture was then sequentially washed with aqueous NaHCO3 and water. After being extracted with ether, the organic layer was dried over MgSO4, filtered, and concentrated. The crude product was purified by column chromatography on silica gel using petroleum ether/ethyl acetate (v/v = 4/1) as the eluent to give 4 (2.12 g) in 45.0% yield. 1H NMR (CDCl3, 400 MHz): δ (ppm) 1.86 (s, 3H, –CH3), 1.98 (s, 3H, –CH3), 7.16 (s, 1H, thiophene-H), 7.30–7.32 (m, 1H, phenyl-H), 7.44–7.46 (m, 2H, phenyl-H), 7.47 (d, 2H, phenyl-H, J = 8.0 Hz), 7.70 (s, 1H, thiophene-H), 9.78 (s, 1H, –CHO); 13C NMR (DMSO-d6, 100 MHz): δ (ppm) 14.6, 14.7, 123.6, 124.6, 124.8, 125.7, 128.0, 128.4, 129.6, 130.0, 130.6, 133.3, 140.1, 141.5, 183.5.
Synthesis of 1o
4 (0.236 g, 0.5 mmol) and 9-ethyl-9H-carbazol-3-amine (0.105 g, 0.5 mmol) were dissolved in methanol (10 mL). The reaction mixture was refluxed for 6 h, and then cooled down to room temperature. The raw product was condensed and purified by recrystallization with methanol to obtain 0.40 g 1o as a yellow solid in 59% yield. M.p. 378–388 K; 1H NMR (DMSO-d6, 400 MHz): δ (ppm) 1.38 (t, 3H, –CH3, J = 8.0 Hz), 2.06 (s, 3H, –CH3), 2.08 (s, 3H, –CH3), 4.47–4.54 (m, 2H, –CH2), 7.26 (s, 1H, thiophene-H), 7.40 (s, 1H, thiophene-H), 7.48–7.52 (m, 3H, benzene-H), 7.56 (d, 2H, J = 8.0 Hz, benzene-H), 7.66–7.72 (m, 4H, benzene-H), 7.77 (s, 1H, benzene-H), 8.24 (d, 2H, J = 8.0 Hz, benzene-H), 9.02 (s, 1H, –CH
N); 13C NMR (CDCl3, 100 MHz): δ (ppm) 13.3, 14.0, 14.5, 37.23, 108.2, 108.3, 112.3, 118.5, 119.4, 120.1, 121.8, 125.2, 125.2, 125.5, 127.5, 128.5, 130.0, 140.1, 148.7; HRMS (ESI+) m/z: 665.1510 [M + H]+, (C36H26F6N2S2 requires 664.1442); anal. calcd. for C36H26F6N2S2 (%), calcd: C, 70.48; H, 3.91; N, 4.22; found: C, 70.50; H, 3.92; N, 4.21.
Preparation of the metal ions
The solutions of metal ions (0.1 mol L−1) were prepared by the dissolution of their respective metal nitrates in distilled water, except for K+, Hg2+, Mn2+, and Ba2+ (where the counter ion was chloride). Distilled-deionized water was used throughout the experiment.
Procedures for metal-ion sensing
Stock solutions of the metal ions (0.1 mol L−1) were prepared in deionized water. A stock solution of 1o (1.0 × 10−3 mol L−1) was prepared in CH3OH, and then diluted to 2.0 × 10−5 mol L−1. Titration experiments were performed by placing 2 mL of 1o in a quartz cuvette of 1 cm optical path length, and then incrementally adding the Sn2+ stock solution by means of a micro-pipette. Spectra were obtained 3 s after the addition. Test samples for the selectivity experiments were prepared by adding appropriate amounts of metal ion stock solutions to 2 mL of 1o. In competition experiments, Sn2+ or Cu2+ was added to the solution containing 1o and the other metal ions of interest. For fluorescence measurements, excitation was provided at 310 nm, and emission was obtained from 350 to 615 nm.
Determination of cyclization/cycloreversion quantum yields
Quantum yield was measured using 1,2-bis(2-methyl-5-phenyl-3-thienyl)perfluorocyclopentene41 as reference for the cyclization and cycloreversion reactions. The absorbance of the sample and the reference at the irradiation wavelength (297 nm) were adjusted to be the same for the cyclization quantum yield measurement. The reaction rate of the sample and reference were measured under the same conditions and were compared. For the cyclization quantum yield measurement, absorbance (A) at the absorption maximum of the closed-ring isomer was plotted against time. For the cycloreversion quantum yield measurement, −log
A at the absorption of the irradiated wavelength was plotted against time. The measurement was carried out five times, and the value was determined by averaging.
Determination of the stoichiometry of the 1o–Sn2+/Cu2+ complexes
According to the method for continuous variation, a series of solutions of 1o and Sn2+/Cu2+ at ratios of 1
:
9, 2
:
8, 3
:
7, 4
:
6, 5
:
5, 6
:
4, 7
:
3, 8
:
2, and 9
:
1 were prepared, and the fluorescence spectra were obtained. When plotting the fluorescence intensity against [M2+]/([M2+] + [1o]), a feature point was obtained at 0.5 on the abscissa, indicating the 1
:
1 stoichiometry of the 1o–Sn2+/Cu2+ complexes.
Determination of the association constant (Ka)
According to the fluorescence titration, the apparent association constant (Ka) was determined using the following equation: F − F0 = ΔF = [M2+](Fmax − F0)/(Ka + [M2+]), where F is the observed fluorescence, Fmax is the saturated fluorescence for the 1o–Sn2+ or 1o–Cu2+ complex, and F0 is the fluorescence for the free 1o. When plotting the reciprocal of ΔF against the reciprocal of concentration of Sn2+ or Cu2+, a linear relation equation was obtained: Y = A + BX. Ka was calculated from A/B.
Determination of the limit of detection (LOD)
The detection limit was calculated based on the fluorescence titration. To determine the S/N ratio, the emission intensity of 1o without any metal ions was measured 15 times and the standard deviation of the blank measurements was determined. Three-independent duplication measurements of emission intensity were performed in the presence of metal ions and each average value of the intensities was plotted as a concentration of metal ions for determining the slope. The detection limit was then calculated using the equation: detection limit = 3σ/A, where σ is the standard deviation of the blank measurements and A is the slope of intensity plotted versus sample concentration.
Real sample analysis
The water sample was filtered through a filter paper to remove some large-size impurities. The solutions of Sn2+/Cu2+ (0.01 mol L−1) were prepared by the dissolution of Sn(NO3)2/Cu(NO3)2 in distilled water (standard solutions) and river water (sample solutions), respectively. The intensity for each of the four standard solutions was determined at 477/401 nm for the fluorescence spectrum. The results were obtained and the working curve was prepared. The intensity of the sample solutions was determined to find out the concentration of Sn2+/Cu2+ by the working curve method.
Results and discussion
The photochromic behavior of 1o was examined in methanol (2.0 × 10−5 mol L−1) at room temperature (Fig. 1A). The absorption maximum of the open-ring form was observed at 287 nm due to the π → π* transition.42 Upon irradiation with 297 nm light, the absorption at 287 nm decreased, and a new absorption band centered at 622 nm emerged due to the formation of the closed-ring isomer 1c, accompanied with a color change from colorless to blue. The blue color could be bleached to colorless upon irradiation with visible light (λ > 500 nm), and the absorption spectrum returned to the initial state of 1o. The cyclization and cycloreversion quantum yields were 0.22 and 0.028, respectively. Moreover, the compound showed good stability at room temperature. No decomposition was detected when the powder was exposed to air for more than six months. After 50 repeat cycles (Fig. S1†), the degradation for 1c was only 7.6%. The fluorescence property of 1o was measured in methanol (2.0 × 10−5 mol L−1) at room temperature (Fig. 1B). When excited at 310 nm, the fluorescent emission peak of 1o appeared at 477 nm. Upon irradiation with 297 nm light, the emission intensity decreased due to the formation of weakly fluorescent closed-ring isomers of 1c.43 The back-irradiation with appropriate visible light (λ > 500 nm) regenerated the open-ring isomer of 1o, and the emission intensity returned to the original state. When the photostationary state was achieved, the emission intensity of 1 was quenched to ca. 40%.
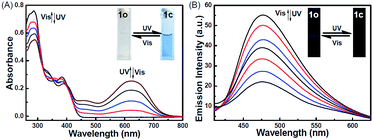 |
| Fig. 1 Absorption spectral and color changes in the absorption and fluorescence of 1o upon alternating irradiation between UV and visible light in methanol: (A) absorption and color changes (2.0 × 10−5 mol L−1) and (B) fluorescence and color changes (2.0 × 10−5 mol L−1), excited at 310 nm. | |
The fluorometric responses of 1o towards metal ions was studied in methanol. The fluorescence of 1o was not significantly influenced in the presence of metal ions such as Zn2+, Co2+, Mn2+, Hg2+, Pb2+, Cd2+, K+, Ca2+, Mg2+, Ba2+, Sr2+, and Ni2+. However, the emission intensity of 1o was selectively altered upon the addition of Sn2+/Cu2+. As shown in Fig. 2, a clear fluorescence enhancement of 1o with a 76 nm blue shift was observed with Sn2+, whereas a reverse response, i.e. fluorescence quenching, was observed upon the addition of Cu2+. The enhancement with Sn2+ has been explained based on the inhibition of C
N isomerization due to chelate formation.44 Moreover, the blue shift indicated that the possible intramolecular charge transfer occurred in 1o on interaction with Sn2+. In contrast, the fluorescence quenching by Cu2+ was most likely due to an energy transfer process occurring from 1o to the open-shell d-orbitals and paramagnetic Cu2+, which caused a faster and more efficient nonradiative decay of the excited states of 1o. Moreover, Cr3+, Al3+, and Fe3+ exerted a slight quenching effect with a 76 nm blue shift. However, compared with the strong fluorescence quenching by Cu2+, their disturbances were quite minor.
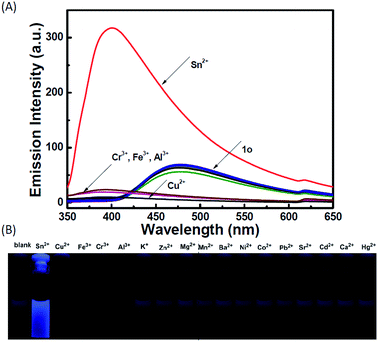 |
| Fig. 2 Changes in the fluorescence of 1o induced by the addition of various metal ions (12 equiv.) in methanol (2.0 × 10−5 mol L−1): (A) emission spectral changes and (B) images demonstrating changes in the fluorescence. | |
To determine the binding stoichiometry between 1o and Sn2+/Cu2+, the continuous variation method was used. Fig. 3 displays the Job's plot of the fluorescence intensity of 1o and the intensity of the system with the molar fraction of the host {[1o]/([1o] + [Sn2+/Cu2+])} for a series of solutions, in which the total concentration of 1o and Sn2+ or Cu2+ was constant with the molar fraction of 1o continuously varying. The inflection point was observed when the molar fraction reached 0.5, which indicates a 1
:
1 binding stoichiometry between 1o and Sn2+/Cu2+. The formation of the 1o–Sn2+/Cu2+ complex was confirmed by ESI mass spectra (Fig. S2†). The free 1o displayed a characteristic peak at 665.1 m/z. When excess amounts of Sn2+ were added to 1o, a new peak at 907.5 m/z emerged that was assigned to 1o–Sn2+ (the calculated [1o + Sn2+ + 2NO3− − H] value was 908.0). When excess amounts of Cu2+ were added to 1o, a new peak at 788.1 m/z emerged that was assigned to 1o–Cu2+ (the calculated [1o + Cu2+ + NO3− − H]+ value was 789.1). The complex formation was further confirmed by IR (Fig. S3†) and 1H NMR (Fig. S4†) spectra, respectively. In the IR spectra, the 1669 cm−1 v(C
N) peak of 1o shifted to 1599 cm−1 due to the reaction with Sn2+ and that of 1o shifted to 1674 cm−1 due to the formation of a bond between –C
N and Cu2+. The new peak at 1380/1388 cm−1 indicated that NO3− was introduced into 1o–Cu2+ and 1o–Sn2+, respectively.45 In the 1H NMR spectra of 1o–Sn2+, the proton (–CH
N) signal at 9.03 ppm showed up-field shifts upon the addition of Sn2+. This indicated that Sn2+ binds to the imine moiety. However, the proton signals could not be detected in the presence of the paramagnetic Cu2+. The possible complex formation is shown in Scheme 1.
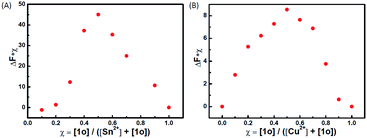 |
| Fig. 3 Job's plot showing the 1 : 1 complex: (A) 1o with Sn2+, and (B) 1o with Cu2+. | |
The fluorescence spectral titrations of 1o (2.0 × 10−5 mol L−1) were performed with incremental addition of Sn2+/Cu2+ to obtain a better idea of the binding constants and binding stoichiometry (Fig. 4). When excited at 310 nm, the fluorescence intensity of 1o at 477 nm was enhanced more than 4.5-fold with a 76 nm blue shift for Sn2+ and quenched more than 5-fold with Cu2+. The association constant Ka was graphically evaluated by plotting F0/(F − F0) against 1/[M2+], as shown in Fig. 5. The fluorescence titration data were linearly fitted according to the Benesi–Hilderbrand equation,46,47 which indicated 1
:
1 binding stoichiometry. The Ka values were obtained from the slope and intercept of the line and the calculated binding constants were 4.04 × 103 L mol−1 and 3.40 × 104 L mol−1 for Sn2+ and Cu2+, respectively. The standard deviation for the three repeated measurements of Ka was 0.88% for Sn2+ and 1.48% for Cu2+. Under optical detection, according to the reported method,48 the detection limit of 1o as a fluorescent sensor for Sn2+/Cu2+ was determined from the plot of fluorescence intensity as a function of the concentration of Sn2+/Cu2+ (Fig. S5†). 1o was found to have the detection limits of 1.9 × 10−6 and 1.2 × 10−6 mol L−1, respectively, which is reasonable for the detection of micromolar concentrations of Sn2+/Cu2+.
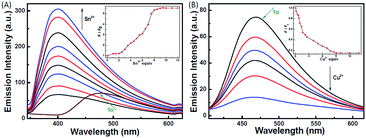 |
| Fig. 4 Changes in the fluorescence of 1o induced by the successive addition of Sn2+ and Cu2+ in methanol (2.0 × 10−5 mol L−1): (A) Sn2+ and (B) Cu2+. Inset: fluorescence intensity at 477/401 nm for 1o as a function of the concentration of Sn2+ and Cu2+, respectively. | |
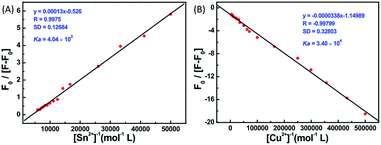 |
| Fig. 5 The Benesi–Hildebrand plots based on the 1 : 1 complexes: (A) 1o–Sn2+ and (B) 1o–Cu2+. | |
To check the interference of other metal ions in the detection of Sn2+ and Cu2+, the interference studies were carried out by monitoring the change in the emission profile of 1o–Sn2+/Cu2+ in the presence of other interfering cations (Zn2+, Al3+, Cr3+, Co2+, Mn2+, Hg2+, Pb2+, Cd2+, K+, Ca2+, Fe3+, Mg2+, Ba2+, Sr2+, and Ni2+). As shown in Fig. 6, the fluorescence profile of 1o with Sn2+ was not affected by the coexistence of other interfering metal ions except Cu2+. In the presence of Cu2+, the fluorescence intensity decreased almost 5-fold, which may be ascribed to the high association constant of 1o for Cu2+. Even in the presence of Sn2+, the fluorescence intensity obviously decreased upon the addition of Cu2+. These studies show that no other metal ions are interfering in the detection of Sn2+ except Cu2+ and the specific response for Cu2+ is not disturbed by other competing metal ions.
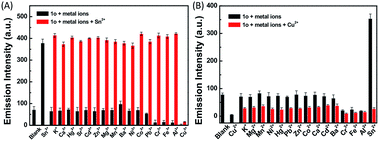 |
| Fig. 6 Emission intensity change upon the addition of different interfering metal ions in methanol (2.0 × 10−5 mol L−1): (A) 1o + Sn2+ and (B) 1o + Cu2+. Bars represent the emission intensity at 477/401 nm. Black bars represent the addition of 10 equiv. of various metal ions to the solution of 1o and red bars represent the addition of Sn2+/Cu2+ (20 equiv.) to the abovementioned solution. | |
To understand the reversibility of 1o towards Sn2+/Cu2+, a reversibility experiment was carried out using disodium salt of ethylene diamine tetraacetate (Na2EDTA), which has a strong binding ability towards metal ions. As shown in Fig. 7, upon the addition of 12 equiv. of EDTA to 1o containing Sn2+, the emission intensity immediately decreased. Further addition of Sn2+ could restore the fluorescent state. The cycle (Sn2+–EDTA) can be carried out four times. This regeneration indicates that 1o can be reused with proper treatment, whereas the fluorescence could not be regained by further addition of excess EDTA, indicating irreversible sensing for Cu2+.
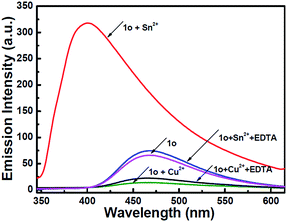 |
| Fig. 7 Reversible binding of 1o–Sn2+/Cu2+ with EDTA. Fluorescence of 1o, 1o in the presence of Sn2+/Cu2+, respectively, and (c) probe in the presence of Sn2+/Cu2+ upon the addition of excess amount of EDTA. | |
The effect of pH on the fluorescence intensity of 1o was investigated in the absence and presence of Sn2+/Cu2+. As shown in Fig. S6,† no obvious fluorescence intensity change of 1o was observed between pH 2.4 and 11.5, suggesting that the compound is stable over a wide range. In the pH range of 4.0–11.5, a marked fluorescence enhancement was observed upon the addition of Sn2+. In the presence of Cu2+, the quenched fluorescence was almost unaffected over a wide range, from pH 2.4 to 12.9. Therefore, 1o can detect Sn2+ and Cu2+ over a wide pH range with a high selectivity and specificity.
Moreover, 1o–Sn2+ exhibited a notable fluorescent switch by photoirradiation, as shown in Fig. 8. The emission intensity of the complex 1o–Sn2+ notably decreased upon irradiation with 297 nm light due to the formation of the closed-ring isomer of 1c–Sn2+. When arriving at the photostationary state, the emission intensity was quenched ca. 52%. The back irradiation with appropriate visible light regenerated the open-ring isomer 1o–Sn2+ and recovered its original emission intensity.
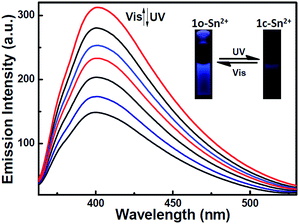 |
| Fig. 8 Fluorescence spectral and color change of 1o–Sn2+ upon alternating irradiation between UV and visible light in methanol (2.0 × 10−5 mol L−1) excited at 310 nm. | |
To demonstrate the real application of 1o, Sn2+/Cu2+ in real water samples from the Ganjiang River of Nanchang City were determined according to the reported methods.49,50 Different amounts of Sn2+/Cu2+ were spiked into the real water sample. The sample was filtered through a 0.2 mm membrane. Tables 1 and 2 show the results obtained using the fluorescent sensor 1o with appropriate added amounts of Sn2+/Cu2+. The recovery for both Sn2+ and Cu2+ was over 90%. Therefore, 1o, which can detect Sn2+/Cu2+ in real water samples with high accuracy, has practical value.
Table 1 Detection of Sn2+ in natural water samples
Sample |
Sn2+ added (nM) |
Sn2+ determined (nM) |
Recovery (%) |
1 |
120 |
127.1 |
105.9 |
2 |
130 |
141.2 |
108.6 |
3 |
140 |
151.2 |
108.0 |
4 |
150 |
166.5 |
111.0 |
Table 2 Detection of Cu2+ in natural water samples
Sample |
Cu2+ added (nM) |
Cu2+ determined (nM) |
Recovery (%) |
1 |
40 |
44.3 |
111 |
2 |
60 |
58.0 |
96.6 |
3 |
80 |
73.7 |
92.2 |
4 |
100 |
94.7 |
94.7 |
Conclusions
A novel diarylethene with a carbazole unit, which shows high sensitivity and selectivity for Sn2+ and Cu2+ in methanol over a wide range of metal ions tested, was designed and synthesized. The sensing of Sn2+ and Cu2+ was not affected in the presence of other interfering cations. The 1
:
1 stoichiometry complexes were confirmed from Job's plot with the detection limits were 1.9 μM for Sn2+ and 1.2 μM for Cu2+. Moreover, the compound showed high accuracy for Sn2+ and Cu2+ in natural sample testing. The results indicate that diarylethene derivatives can be used for practical applications in sensory research.
Acknowledgements
The authors are grateful for the financial support received from the National Natural Science Foundation of China (21363009, 21362013, 51373072), the Science Funds of Natural Science Foundation of Jiangxi Province (20142BAB203005, 20132BAB203005), the Young Scientist Training Program of the Jiangxi Province (20153BCB23008), and the JXSTNU Sci-Tech innovation team (2015CXTD002), the Project of the Science Funds of Jiangxi Education Office (GJJ160774).
Notes and references
- T. Q. Duong and J. S. Kim, Chem. Rev., 2010, 110, 6280–6301 CrossRef PubMed.
- J. M. Lehn, Supramolecular Chemistry—Conceptand Perspective, VCH, Weinheim, 1995 Search PubMed.
- M. Chhatwal, A. Kumar, V. Singh, R. D. Gupta and S. K. Awasthi, Coord. Chem. Rev., 2015, 292, 30–55 CrossRef CAS.
- G. R. C. Hamilton, S. K. Sahoo, S. Kamila, N. Singh, N. Kaur, B. W. Hyland and J. F. Callan, Chem. Soc. Rev., 2015, 44, 4115–4132 RSC.
- L. Basabedesmonts, D. N. Reinhoudt, M. Cregocalama, S. Sahoo, J. Marek and N. Singh, Chem. Informationsdienst, 2007, 36, 993–1017 RSC.
- A. Kuwar, R. Patil, A. Singh and Y. T. Yang, J. Mater. Chem. C, 2014, 3, 453–460 RSC.
- M. Vendrell, D. Zhai, J. C. Er and Y. T. Yang, Chem. Rev., 2012, 112, 4391–4420 CrossRef CAS PubMed.
- H. Rüdel, Ecotoxicol. Environ. Saf., 2003, 56, 180–189 CrossRef.
- N. Cardarelli, Thymus, 1990, 15, 223–231 CAS.
- L. R. Sherman, J. Masters, R. Peterson and S. Levine, J. Anal. Toxicol., 1986, 10, 6–9 CrossRef CAS PubMed.
- C. M. Viau, J. M. Cardone, T. N. Guecheva, M. L. Yoneama, J. F. Dias, F. G. Sousa, C. Pungartnik, M. Brendel and J. A. Henriques, Arch. Toxicol., 2009, 83, 769–775 CrossRef CAS PubMed.
- B. E. Kim, T. Nevitt and D. J. Thiele, Nat. Chem. Biol., 2008, 4, 176–185 CrossRef CAS PubMed.
- J. Y. Jung, M. Kang, J. Chun, J. Lee, J. Kim, Y. Kim, S. J. Kim, C. Lee and J. Soon, Chem. Commun., 2013, 49, 176–178 RSC.
- V. K. Gupta, M. R. Ganjali and P. Nayak, Anal. Chem., 2011, 41, 282–313 CAS.
- S. Lutsenko, A. Gupta, J. L. Burkhead and V. Zuzel, Arch. Biochem. Biophys., 2008, 476, 22–32 CrossRef CAS PubMed.
- M. Didonato and B. Sarkar, Biochim. Biophys. Acta, 1997, 1360, 3–16 CrossRef CAS.
- E. Gaggelli, H. Kozlowski, D. Valensin and G. Valensin, Chem. Rev., 2006, 106, 1995–2004 CrossRef CAS PubMed.
- H. Kozlowski, M. Luczkowski, M. Remelli and D. Valensin, Coord. Chem. Rev., 2012, 256, 2129–2141 CrossRef CAS.
- E. L. Que, D. W. Domaille and C. J. Chang, Chem. Rev., 2008, 108, 1517–1549 CrossRef CAS PubMed.
- M. Irie, Chem. Rev., 2000, 100, 1685–1716 CrossRef CAS PubMed.
- X. Q. Chen, T. Pradhan, F. Wang, J. S. Kim and J. Yoon, Chem. Rev., 2012, 112, 1910–1956 CrossRef CAS PubMed.
- K. P. Carter, A. M. Young and A. E. Palmer, Chem. Rev., 2014, 114, 4564–4601 CrossRef CAS PubMed.
- M. Irie, T. Fukaminato, K. Matsuda and S. Kobatake, Chem. Rev., 2014, 114, 12174–12277 CrossRef CAS PubMed.
- S. Z. Pu, Q. Sun, C. B. Fan, R. J. Wang and G. Liu, J. Mater. Chem. C, 2016, 4, 3075–3093 RSC.
- X. J. Piao, Y. Zou, J. C. Wu, C. Y Li and T. Yi, Org. Lett., 2009, 11, 3818–3821 CrossRef CAS PubMed.
- C. C. Zhang, S. Z. Pu, Z. Y. Sun, C. B. Fan and G. Liu, J. Phys. Chem. B, 2015, 119, 4673–4682 CrossRef CAS PubMed.
- Q. Zou, X. Li, J. J. Zhang, J. Zhou, B. Sun and H. Tian, Chem. Commun., 2012, 48, 2095–2097 RSC.
- C. H. Zheng, G. Liu, S. Z. Pu and B. Chen, Tetrahedron Lett., 2013, 54, 5791–5794 CrossRef CAS.
- S. Y. Huang, Z. Y. Li, S. S. Li, J. Yin and S. H. Liu, Dyes Pigm., 2012, 92, 961–966 CrossRef CAS.
- J. Q. Ren and H. Tian, Sensors, 2007, 7, 3166–3178 CrossRef CAS.
- S. Z. Pu, C. C. Zhang, C. B. Fan and G. Liu, Dyes Pigm., 2016, 129, 24–33 CrossRef CAS.
- L. Xu, S. Wang, Y. N. Lv, Y. A. Son and D. Cao, Spectrochim. Acta, Part A, 2014, 128, 567–574 CrossRef CAS PubMed.
- T. Fukaminato, T. Sasaki, T. Kawai and M. Irie, J. Am. Chem. Soc., 2004, 126, 14843–14849 CrossRef CAS PubMed.
- S. S. Wei, C. H. Zheng, G. Liu, B. Chen and S. Z. Pu, J. Photochem. Photobiol., A, 2015, 307–308, 48–53 CrossRef CAS.
- S. Z. Pu, Y. M. Xue, C. H. Zheng, W. W. Geng, S. Q. Cui and G. Liu, Tetrahedron, 2014, 70, 9070–9076 CrossRef CAS.
- Z. R. Grabowski, K. Rotkiewicz and W. Rettig, Chem. Rev., 2003, 103, 3899–4031 CrossRef PubMed.
- Y. Xing, H. Lin, F. Wang and P. Lu, Sens. Actuators, B, 2006, 114, 28–31 CrossRef CAS.
- Z. J. Zhao, Y. J. Xing, Z. X. Wang and P. Lu, Org. Lett., 2007, 9, 547–550 CrossRef CAS PubMed.
- A. Peters, C. Vitols, R. McDonald and N. R. Branda, Org. Lett., 2003, 5, 1183–1186 CrossRef CAS PubMed.
- S. L. Gilat, S. H. Kawai and J. M. Lehn, J. Chem. Soc., Chem. Commun., 1993, 1439–1442 RSC.
- M. Irie, T. Lifka, A. S. Kobatake and S. Kobatake, J. Am. Chem. Soc., 2000, 122, 4871–4876 CrossRef CAS.
- Z. X. Li, L. Y. Liao, W. Sun, C. H. Xu, C. Zhang, C. J. Fang and C. H. Yan, J. Phys. Chem. C, 2008, 112, 5190–5196 CAS.
- T. Kawai, M. S. Kim, T. Sasaki and M. Irie, Opt. Mater., 2003, 21, 275–278 CrossRef CAS.
- B. N. Ahamed and P. Ghosh, Dalton Trans., 2011, 40, 6411–6419 RSC.
- C. W. Frank and L. B. Rogers, Inorg. Chem., 2002, 5, 1543–1548 Search PubMed.
- H. A. Benesi and J. H. Hildebrand, J. Am. Chem. Soc., 1949, 71, 2703–2707 CrossRef CAS.
- M. Barra, C. Bohne and J. C. Scaiano, J. Am. Chem. Soc., 1990, 112, 8075–8079 CrossRef CAS.
- D. L. Massart, B. G. Vandeginste, L. M. C. Buydens, S. De Jong, P. J. Lewi and J. S. Verbeke, Handbook of Chemometrics and Qualimetrics: Part A, Eselvier, Amsterdan, Netherlands, 1997, ch. 13 Search PubMed.
- Y. T. Chen, Y. S. Mi, Q. F Xie, J. N. Xiang, H. L. Fan, X. B. Luo and S. R. Xia, Anal. Methods, 2013, 5, 4818–4823 RSC.
- X. Wu, J. Chen and J. X. Zhao, Analyst, 2013, 138, 5281–5287 RSC.
Footnote |
† Electronic supplementary information (ESI) available. See DOI: 10.1039/c6ra27339d |
|
This journal is © The Royal Society of Chemistry 2017 |
Click here to see how this site uses Cookies. View our privacy policy here.