DOI:
10.1039/C7RA01087G
(Paper)
RSC Adv., 2017,
7, 10469-10478
Continuous flow synthesis of indoles by Pd-catalyzed deoxygenation of 2-nitrostilbenes with carbon monoxide†
Received
24th January 2017
, Accepted 1st February 2017
First published on 7th February 2017
Abstract
The palladium-catalyzed deoxygenation of o-vinylnitrobenzenes employing carbon monoxide as a terminal reductant produces indoles in a continuous flow environment. The reaction proceeds with catalyst loadings of 1 to 2 mol% Pd(OAc)2 in the presence of suitable ligands/additives and generates carbon dioxide as the only stoichiometric side-product. The reductive cyclization proceeds in a clean fashion with high initial reaction rates in the pressurized flow reactor, ultimately leading to deposition of catalytically inactive palladium(0) inside the channels of the flow device, allowing for an efficient catalyst recovery. A variety of o-vinylnitrobenzenes (o-nitrostilbenes and -styrenes) were converted to the corresponding indoles within 15 to 30 min at a reaction temperature of 140 °C to furnish products in good to excellent yields (10–20 bar CO pressure). Mechanistic aspects and the scope of the transformation are discussed.
Introduction
Indoles are among the most abundant heterocycles in nature and a vastly diverse array of natural and synthetic compounds contain the indole scaffold. Even though first methods for the generation of indoles were discovered well over a century ago, the ubiquity and structural diversity of biologically active indoles has continued to drive research towards their synthesis and functionalization.1 In particular, the development of regioselective methods for the construction of indoles with complex substitution patterns has remained an active area of research.1 In the 1960s, Cadogan and Sundberg reported the generation of indoles by reductive cyclization of o-nitrostyrenes (cf. Scheme 1).2 For the Cadogan–Sundberg indole synthesis, the nitroaromatic compound is thereby typically heated in boiling triethyl phosphite to accomplish a deoxygenative cyclization.2 This approach is appealing for the construction of highly functionalized indoles, since the reaction is inherently regioselective and the respective starting materials can be assembled in a convergent manner by highly developed, robust transformations. On the other hand, the extreme reaction conditions (reaction temperatures in excess of 150 °C) and the generation of large amounts of phosphorus waste renders this transformation increasingly unacceptable in contemporary organic synthesis. Transition metal catalyzed alternatives employing carbon monoxide as stoichiometric reducing agent were developed in the 1990s (Scheme 1).3 In particular, palladium-based systems have proven to be effective for this transformation. Watanabe and co-workers demonstrated a procedure for the reductive cyclization of 2-ethenyl-1-nitrobenzenes employing PdCl2(PhCN)2 as catalyst and triphenylphosphine as the ligand.4 A stoichiometric amount of tin dichloride and a CO pressure of 20 bar was required to yield the corresponding indoles within a reaction time of 16 h at 100 °C.4 Söderberg and co-workers accomplished the indolization with 6 mol% Pd(OAc)2 and 24 mol% triphenylphospine as ligand using MeCN as solvent.5 Typically, the reaction was completed after reaction times of 15 h at 70 °C under a CO pressure of 4 bar to furnish the indoles in fair to excellent yields (40 to 100%).5 More recent work has demonstrated the great potential of this transformation for the construction of functionalized indoles.6–8 For instance, a thorough study by Davies and co-workers revealed that the reaction proceeds under comparatively mild conditions and with high functional group tolerance.8 With catalyst loadings as low as 1 mol%, a variety of o-nitrostyrenes were converted to the respective indoles within reaction times of 16 h at 80 °C.8 Alternative procedures using molybdenum hexacarbonyl [Mo(CO)6] as a convenient but expensive source of CO have been developed.9
 |
| Scheme 1 Reductive cyclization of o-vinylnitrobenzenes. | |
Even though the reductive cyclization of o-nitrostyrene derivatives with CO as stoichiometric reductant provides an appealing approach for the synthesis of indoles, the necessity to employ a highly toxic gas under elevated pressure and temperature has severely limited the practicality of this transformation. Continuous flow microreactors represent an excellent platform for multiphase reactions.10–12 Since mass transfer between the phases is directly proportional to the interfacial area per unit volume, increasing interfacial area is crucial for achieving high reaction rates and to minimize unwanted side reactions in the homogeneous phase. Using dedicated continuous flow micromixers, specific interfacial areas orders of magnitude larger than in conventional stirred tank reactors can be established.10–12 Importantly, large, well-defined specific interfacial areas can be maintained up to high production volumes. In particular, gas–liquid reactions benefit tremendously from the unique characteristics of microreactors.12,13 Gases can be dosed into the flow system with precise stoichiometry using mass flow controllers and intense mixing of the gaseous and liquid phase can be achieved. Furthermore, high pressure operation increases the concentration of the gas in the liquid phase (Henry's law). It is not surprising, therefore, that continuous flow reactions with gaseous carbon monoxide as reagent have found quite considerable usage.13,14 Indeed, in several cases it was reported that carbonylation reactions in microreactors performed considerably cleaner compared to batch reactions under otherwise identical conditions.13
Herein we present an intensified continuous flow protocol for the reductive cyclization of o-vinylnitrobenzenes of type 1 (Scheme 1). The reactions proceeded with low catalyst loadings within residence times of only 15 to 30 minutes at 140 °C and CO pressures of 10–20 bar. The protocol was successfully applied for the generation of various 2-aryl-substituted indoles. The products were isolated with excellent purities, generally without the need for chromatography.
Results & discussion
The initial flow reactor setup consisted of an HPLC pump for introducing the liquid feed (Scheme 2; see also Fig. S1 in the ESI†). CO gas was fed from a gas cylinder into the system via a mass flow controller. The liquid and gaseous streams were combined in a simple stainless steel T-mixer at room temperature. The T-mixer was connected to the residence tube reactor via a fluoropolymer tubing (PFA, 1/16′′ o.d., 0.8 mm i.d.). The PFA tubing allowed visual observation of the flow profile. The residence time reactor was a 20 mL stainless steel coil heated on an aluminum heating block (Uniqsis FlowSyn). The processed reaction mixture finally exited the system through a short cooling loop in a water bath (1 mL stainless steel, 1/16′′ o.d., 1 mm i.d.) and an adjustable back-pressure regulator (0 to 25 bar). Pressure sensors were integrated into the T-mixer and directly after the pump (PS1 and PS2; Scheme 2). The evolution of carbon dioxide during the reaction could be conveniently monitored by in-line FTIR analysis (Mettler Toledo ReactIR with silicon probe).15 The strongly IR active asymmetrical stretch band of CO2 in the region from 2300 to 2400 cm−1 was the dominant feature in the IR-spectrum (see Fig. 1). The weaker stretch band of CO was detectable in the region of 2100 and 2200 cm−1.15
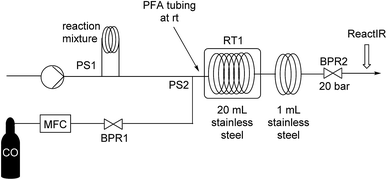 |
| Scheme 2 Experimental setup for continuous flow deoxygenations with carbon monoxide. | |
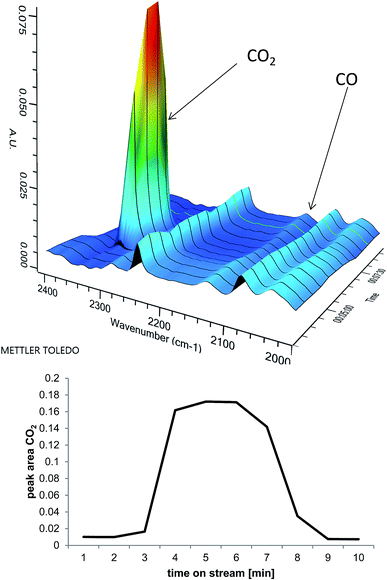 |
| Fig. 1 Top: 3-D surface image showing for the strongly IR active asymmetrical stretch band of CO2 in the region from 2300 to 2400 cm−1, and the weaker CO absorption in the region of 2100 to 2200 cm−1. Bottom: evolution of CO2 during a reaction. | |
Initial experiments were performed with methyl 2-nitrocinnamate (1a) as model substrate under conditions close to those reported by Davies and co-workers (Table 1).8 For these reactions, 0.9 mmol of the substrate, Pd(OAc)2 (2 mol%) and 1,10-phenanthroline ligand (phen) were dissolved in the respective solvent (3 mL). The solutions were loaded into the sample loop of the 6-port injection valve. When the reaction was started, the injection valve was switched and the reaction mixture was carried into the mixer, where it was combined with carbon monoxide. The liquid and the gaseous feed were pumped at flow rates of respectively 0.5 and 5 mL min−1 for the initial reactions. This corresponded to 1.4 equiv. of CO and thus less than required by stoichiometry (2 equiv.). The palladium(II) acetate was reduced to palladium(0) immediately upon contact with CO and the Pd(0) partly deposited on the PFA tubing which connected the T-mixer and the stainless steel residence coil (Scheme 2).16 Furthermore, palladium(0) particles were observed in the collected reaction mixtures. Even though considerable amounts of palladium were lost from the mixture by precipitation on the tubing, reasonable conversions and remarkably clean reactions were obtained (Table 1). The only side product detectable in the reaction mixture in appreciable amounts showed an m/z of 191, and is probably N-hydroxyindole 3a (Table 1). Conversions of methyl 2-nitrocinnamate (1a) between 80 and 90% were obtained in all tested solvents after a residence time of ∼30 min at 120 °C (Table 1). DMF and MeCN stabilize the palladium(0) better than the other tested solvents and less Pd-particles were found in the processed reaction mixtures. Only traces of N-hydroxyindole 3a were formed in MeCN as solvent. In contrast, significant amounts of N-hydroxyindole 3a were produced in less polar solvents, such as dioxane and EtOAc. Interestingly, the corresponding reaction with 2-nitrocinnamic acid did not provide the expected indole-2-carboxylic acid as product. Instead, compounds derived from nitro reduction and from decarboxylation with subsequent reductive cyclization were formed (Scheme S1 in the ESI†).
Table 1 Reductive cyclization of methyl 2-nitrocinnamate (1a) in various solvents at 120 °C (Scheme 2)a
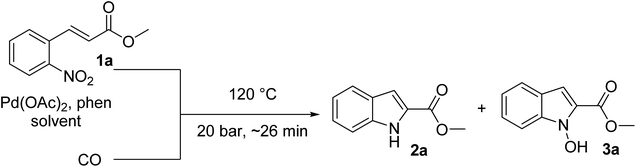
|
|
Solvent |
1ab (%) |
2ab (%) |
3ab(%) |
Othersb,c (%) |
Conditions: 0.9 mmol substrate 1a, 2 mol% Pd(OAc)2 and 4 mol% 1,10-phenanthroline in 3 mL solvent. Flow rate gas/liquid: 5.0/0.5 mL min−1 (∼1.4 equiv. of CO); ∼20 bar pressure; residence time ∼30 min (Scheme 2). HPLC peak area integration at 215 nm. Unidentified compounds. |
1 |
MeCN |
14 |
85 |
1 |
1 |
2 |
DMF |
9 |
83 |
7 |
0 |
3 |
Dioxane |
8 |
64 |
28 |
0 |
4 |
EtOAc |
20 |
45 |
35 |
0 |
5 |
Toluene |
1a not soluble |
6 |
i-PrOH |
1a not soluble |
Formation of N-hydroxyindoles has previously been reported for reductive cyclizations involving CO and has been taken as evidence for a reaction mechanism involving partial deoxygenation of nitrostyrene to a nitrosostyrene intermediate (mechanism A in Scheme 3).8 Spontaneous intramolecular electrocyclization of the nitrosostyrene, followed by [1,5]-hydrogen shift and isomerization yields the N-hydroxyindole 3.17 A subsequent palladium-catalyzed reduction of the N-hydroxyindole 3 with CO as terminal reductant forms indole 2 as the final product (Scheme 3).8 In contrast, earlier mechanistic investigations by Watanabe, Cenini, and Söderberg have suggested the formation of a metal nitrene as intermediate (IV) by exhaustive deoxygenation of the nitrostyrene 1 (mechanism B in Scheme 3).3,4,7 Cyclization followed by reductive elimination directly generates the indole 2 without a N-hydroxyindole intermediate.7 As suggested by Söderberg, both mechanisms may operate depending on the specific reaction conditions.7b This is supported by kinetic data from our laboratory, acquired in various solvents and at various temperatures. The latter cycle (B) predominates in MeCN as solvent, while hydroxyindole 3a slowly accumulates in EtOAc or toluene (for additional experiments, see the ESI†).
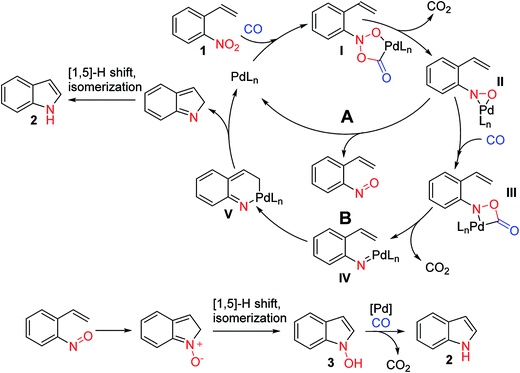 |
| Scheme 3 Putative mechanisms for palladium-catalyzed indolization of o-nitrostyrene (coordination of Pd to the double bond of the o-nitrostyrene has been omitted for clarity).7b | |
For subsequent experiments the catalyst loading was further reduced to 1 mol%. At this catalyst loading, the conversion increased significantly with increasing 1,10-phenanthroline loading. Probably, the phenanthroline ligand stabilizes the Pd(0) and prevents deposition of Pd black on the tubing wall (Table 2).
Table 2 Influence of 1,10-phenanthroline ligand loadinga
|
phen (%) |
1ab (%) |
2ab (%) |
Othersb,c (%) |
Conditions: 0.9 mmol substrate 1a, 1 mol% Pd(OAc)2, 1,10-phenanthroline in 3 mL MeCN. Flow rate gas/liquid: 5.0/0.5 mL min−1 (∼1.4 equiv. CO); ∼20 bar pressure; 120 °C; residence time ∼30 min (Scheme 2). HPLC peak area integration at 215 nm. Unidentified compounds. |
1 |
1% |
82 |
18 |
0 |
2 |
2% |
54 |
42 |
4 |
3 |
4% |
32 |
65 |
3 |
4 |
8% |
27 |
67 |
4 |
As expected, better conversions were obtained with increased stoichiometry of CO. However, more than 2 equiv. of CO did not increase the conversion further (Table S2 in the ESI†). In addition, temperature and reaction time had surprisingly little effect on the reaction (see Table 3). In particular, decreasing the residence time did not decrease the conversion as strongly as expected. Indeed, at a reaction temperature of 140 °C, the conversion increased with increasing flow rate (entries 1 to 4 in Table 3). We hypothesized that at higher flow rates less of the palladium precipitates on the initial PFA tubing at room temperature (cf. Scheme 2) and more palladium is therefore available in the stainless steel tube at 140 °C. Therefore, all subsequent reactions were performed with a heated static mixer, to allow the combination of the gaseous CO feed with the liquid reaction mixture directly at elevated temperatures (Uniqsis glass static mixer; 1.8 mL mixing volume). With the static mixer heated to 140 °C, the conversion increased to >90% (entry 6 in Table 3). The stainless steel residence coil was then removed, still providing >90% conversion after a residence time of ∼3 min at 160 °C (entry 8 in Table 3). The reaction was remarkably clean with methyl indole-2-carboxylate (3b) as the virtually only product. Even with a catalyst loading of only 1 mol%, palladium(0) immediately formed upon contact with CO and precipitated on the channels of the static mixer. Indeed, after only a few experiments, a palladium film covered the channel of the static mixer over most of its length (Fig. S4 in the ESI†). Notably, the palladium(0) on the mixer showed no catalytic activity for reductive cyclization (entry 10 in Table 3). Decreasing the amount of Pd(OAc)2 below 1 mol% decreased the conversions significantly (entry 9 in Table 3).
Table 3 Continuous flow reductive cyclization of 1a with and without a static mixera
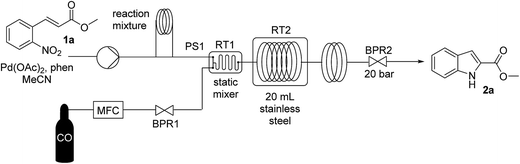
|
|
Pd(OAc)2/phen (mol%) |
Flow rate liquid (mL min−1) |
Flow rate CO (mL min−1) |
Temp RT1b (°C) |
Temp RT2b (°C) |
rtc (min) |
1ad (%) |
2ad (%) |
Othersd,e (%) |
Conditions: 0.9 mmol substrate 1a, Pd(OAc)2 and 1,10-phenanthroline in 3 mL MeCN; ∼2.2 equiv. of CO; ∼20 bar pressure. Temp RT1/RT2 = temperature of static mixer/stainless steel residence tube. rt = residence time (see Experimental section for details). HPLC peak area integration at 215 nm. Unidentified compounds. |
1 |
1/4 |
0.5 |
8 |
— |
140 |
22 |
39 |
61 |
0 |
2 |
1/4 |
1 |
16 |
— |
140 |
11 |
28 |
72 |
0 |
3 |
1/4 |
2 |
32 |
— |
140 |
5 |
24 |
76 |
0 |
4 |
1/4 |
3 |
48 |
— |
140 |
3 |
21 |
79 |
0 |
5 |
1/4 |
0.5 |
8 |
110 |
140 |
20 |
19 |
80 |
1 |
6 |
1/4 |
0.5 |
8 |
140 |
140 |
23 |
7 |
92 |
1 |
7 |
1/4 |
0.5 |
8 |
140 |
— |
3 |
34 |
65 |
1 |
8 |
1/4 |
0.5 |
8 |
160 |
— |
3 |
7 |
92 |
1 |
9 |
0.5/2 |
0.5 |
8 |
160 |
— |
3 |
56 |
44 |
0 |
10 |
0/4 |
0.5 |
8 |
160 |
— |
3 |
100 |
0 |
0 |
Davies and co-workers obtained efficient cyclization at low catalyst loading with 3,4,7,8-tetramethyl-1,10-phenanthroline (tm-phen) as ligand in DMF as solvent.8 Under our conditions, reactions with phenanthroline provided slightly better results than reactions with tm-phen (entry 1 and 2 in Table 4). Furthermore, despite the similar structure of phen and bipyridyl, the conversion decreased to <5% using the latter ligand (entry 3 and 4 in Table 4). Likewise, essentially no reaction was observed using 4,5-diazafluoren-9-one (daf) as ligand (entry 5 in Table 4). The palladium-catalyzed reductive cyclization is often performed using phosphines as ligands.5,6 Surprisingly, methyl 2-nitrocinnamate (1a) exhibited only low reactivity in the presence of various phosphine ligands under the present reaction conditions (conversion <10%; entry 7 in Tables 4 and S3 in the ESI†). Better results were obtained with mixtures of phenanthroline and phosphines (entry 8 to 11 in Table 4). Palladium(0) in combination with mixtures of phen and bidentate phosphine ligands was identified as an efficient catalyst system by Söderberg.7 However, in our hands, significant amounts of the N-hydroxyindole 3a were formed using these conditions.
Table 4 Continuous flow reductive cyclization of 3b in the presence of various nitrogen ligandsa
As shown in Table 4, best results were obtained with 1,10-phenanthroline as ligand (entry 1 in Table 4). With 1 mol% Pd(OAc)2 and 4 mol% ligand, conversions of ∼70% were achieved after a residence time of ∼3 min at 140 °C. Increasing the residence time above ∼3 min did not increase the conversion appreciably (Fig. 2a). Furthermore, increasing the temperature did not drive the reaction to completion (Fig. 2b). Gratifyingly, the addition of tributylamine further stabilized palladium. The reaction proceeded to >90% conversion within a residence time of 15 min at 140 °C even with reduced amounts of phenanthroline (Fig. 2). The reaction remained very clean and only the expected indole 2a was detected by HPLC-UV/Vis analysis. Upon concentration of the processed mixture to about 50% of its original volume, the pure indole 2a precipitated from the solution and was isolated by filtration in 81% yield.
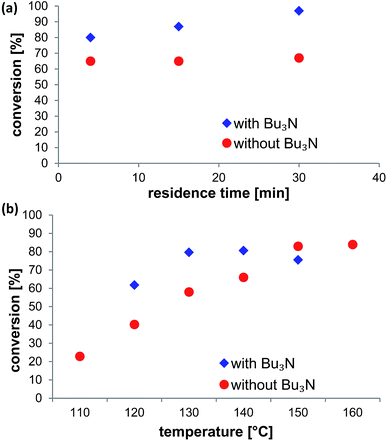 |
| Fig. 2 HPLC conversions after reactions with various residence times (140 °C; (a)) and temperature (∼3 min residence time; (b)) using Pd(OAc)2/phen (1 mol%/4 mol%) or Pd(OAc)2/phen/Bu3N (1 mol%/2 mol%/20 mol%) as catalyst system. | |
Palladium phenanthroline complexes have been demonstrated to be among the most active catalysts for the reductive carbonylation of nitrobenzenes to isocyanates.18 However, nitroaromatic compounds did not react under the reaction conditions employed for the reductive cyclization (see Table S4 in the ESI†). Neither nitrobenzenes with electron-withdrawing nor with electron-donating substituents exhibited any reactivity. Notably, ethyl 4-nitrocinnamate was recovered unchanged after subjecting it to the reaction conditions (Scheme 4). As already observed by Söderberg, a close proximity of the double bond to the nitro group seems to be crucial for the reduction to occur.5 Indeed, the presence of nitrobenzene apparently interferes with the reductive cyclization and nitrostilbene 1b did not cyclize in the presence of 9% nitrobenzene (see Table S5 in the ESI†). A similar observation was reported by Söderberg and co-workers.6b
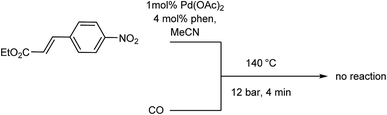 |
| Scheme 4 Unsuccessful attempts for the reduction of nitro arenes. | |
With optimized conditions for the reductive cyclization in hand, the scope was briefly investigated using a variety of substituted o-nitrostilbenes. The corresponding o-nitrostilbenes were easily prepared employing sealed vessel microwave heating using an aqueous/CHCl3-biphasic Wittig reaction (40 min at 100 °C, see the ESI†).19 The nitrostilbenes were isolated as E/Z mixtures after column chromatography in good to excellent yields. The E/Z mixture of 1j was further separated by column chromatography to give pure E-1j and Z-1j in 23 and 51% yield, respectively (see the ESI†). The reductive cyclization of stilbenes 1 was performed in the flow system shown in Table 3 using the glass static mixer and a subsequent 20 mL stainless steel tube reactor (Table 5). The amount of catalyst was increased to 2 mol% for these reactions. No further optimization was attempted as it has previously been reported that the reaction is largely independent of the substitution pattern and the electronic properties of the substituents on the aromatic rings.5,6e,7 In contrast to these previous reports, the conversion obtained for the continuous flow reactions using our experimental conditions depended strongly on the electron density of the stilbene. For instance, while reactions proceeded to high conversions with stilbenes bearing an electron withdrawing CF3-moity (1b to 1e), conversions <16% were obtained for stilbenes 1k to 1m with the strongly electron donating NMe2 group on the aromatic ring containing the nitro group (Table 5). Reprocessing of the mixture did not increase the conversion appreciably. Furthermore, increasing reaction temperature did not have a noticeable effect. With the cyano-derivative, (Z)-4-(2-nitrostyryl)benzonitrile Z-1j, the conversion increased to 57%. After a second pass through the reactor, a conversion of 95% was obtained and the product was isolated in 74% yield (Table 5). A clear trend is not easily discernible from the experiments shown in Table 5, and both inherent reaction rate and stabilization of palladium(0) by the stilbene are likely to play a decisive role. The intermediate homogeneous Pd(0) species have the tendency to aggregate to clusters, which ultimately irreversibly precipitate from the mixture in the form of catalytically inactive Pd black. Since π-complexation and stabilization of low-valent palladium by electron-poor alkenes is generally stronger, the active species is available for more catalytic cycles and the reaction proceeds to higher conversions in these cases. Reactions with substrates 1f to 1i proceeded to conversions ranging from 95% for the cyano substituted stilbene 1f to 14% for the fluoro derivative 1i (Table 5). Again, a second pass through the reactor did not drive the reaction to higher conversions. ICPMS analysis revealed that more than 90% of the palladium was already lost from the mixture after stilbene 1f and 1g were processed through the static mixer (no residence tube; ∼3 min residence time; see Table S6 in the ESI†). These results suggest that the palladium-catalyzed indolization is inherently fast, but the catalyst is quickly lost from the solution. In contrast, almost 50% of palladium was still present in the processed reaction mixture containing methyl 2-nitrocinnamate (1a) as substrate. With no stabilizing alkene in the reaction mixture, 97% of the palladium was lost according to ICPMS analysis. Importantly, the palladium could be essentially quantitatively recovered from the static mixer by washing the reactor with aqua regia (Table S6 in the ESI†).
Table 5 Continuous flow reductive cyclization of o-nitrostilbenes 1 with CO as terminal reductanta
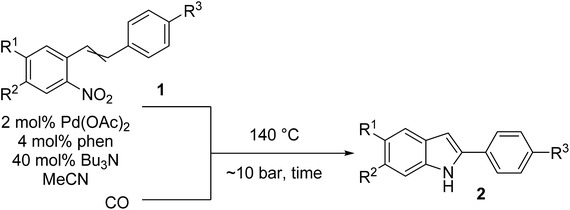
|
|
R1 |
R2 |
R3 |
First passb (rt = 15 min) |
Second passb (rt = 30 min) |
Yieldc (%) |
Conditions: see Experimental section. HPLC peak area integration. Isolated yields of pure compounds. Purity of compounds was determined by 1H NMR, HPLC and GC-MS analysis. n.d. = not determined. |
1b |
H |
CF3 |
CN |
57 |
89 |
80 |
1c |
H |
98 |
— |
90 |
1d |
tBu |
57 |
95 |
85 |
1e |
F |
90 |
— |
83 |
1f |
H |
H |
CN |
95 |
— |
90 |
1g |
H |
81 |
81 |
70 |
1h |
tBu |
37 |
39 |
n.d. |
1i |
F |
14 |
16 |
n.d. |
Z-1j |
H |
NMe2 |
CN |
57 |
95 |
74 |
1k |
H |
16 |
25 |
n.d. |
1l |
tBu |
13 |
14 |
n.d. |
1m |
F |
6 |
14 |
n.d. |
1n |
Cl |
H |
CN |
41 |
— |
40 |
1o |
H |
51 |
58 |
45 |
1p |
F |
87 |
— |
80 |
The stereochemistry of the alkene had little effect on the reaction and the indolization of both E-1j and Z-1j proceeded with essentially identical reaction rates (Table S7 in the ESI†). Slightly faster reactions were usually observed for the E isomer when mixtures of the E/Z-stilbenes were processed (Table S7 in the ESI†). Even though full conversion was not obtained for all stilbenes under the present reaction conditions, the reactions were very clean and only the expected indole was observed by HPLC-UV/Vis analysis of the crude reaction mixtures. Indole 1n immediately crystallized from the mixture after the continuous flow reaction and was isolated by simple filtration. Indole 1b was isolated by column chromatography. For the other indoles, the solvent was evaporated and the products were finally recrystallized from petroleum ether (see Experimental section for details).
The reaction was finally scaled up to 60 mL (12 mmol substrate) in the same reactor using methyl 2-nitrocinnamate (1a) as substrate and 1 mol% catalyst loading (Scheme 5). For this reaction the injection loop was removed and the HPLC pump was replaced by a high-pressure syringe pump. At a reaction time of 140 °C and a residence time of 15 min, conversions of 95% were afforded with 2-nitrocinnamate (1a) as substrate. The product crystalized from the mixture upon standing to furnish the pure indole 2a in 84% product yield. ICPMS analysis revealed that the isolated indole 2a contained 45 ppm of palladium. Recrystallization of the indole reduced the amount to 16 ppm without reducing product yield appreciably.20
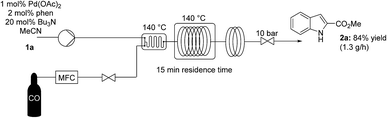 |
| Scheme 5 Palladium-catalyzed reductive cyclization of methyl 2-nitrocinnamate (1a). | |
Conclusion
We have presented a continuous flow reductive cyclization of various o-vinylnitrobenzenes with carbon monoxide as terminal reductant. The reaction was performed with catalyst loadings of 1 to 2 mol% Pd(OAc)2 using 1,10-phenanthroline/tributylamine as ligand system. The carbon monoxide reduced palladium(II) to palladium(0) nearly instantly and palladium quickly deposited on the channels of the flow system. Thus, for less reactive o-nitrostilbenes the reaction came to a halt before reasonable conversions were obtained. Nevertheless, in the pressurized flow reactor, reactions proceeded to high conversions for a variety of stilbenes within reaction times of 15 to 30 min. In general, only the indole was detected in the crude reaction mixtures and the products were isolated in high purity without the need for column chromatography.
The reductive cyclization of o-nitrostyrenes provides a general and inherently regioselective approach to indoles. The reaction proceeds under neutral conditions and tolerates a broad range of functionality. The respective starting materials can be assembled in a convergent manner by well-developed, robust transformations. Furthermore, using CO as the stoichiometric reductant and palladium as catalyst, CO2 is the only stoichiometric by-product. The continuous flow indolization reported herein proceeded with reaction speeds 1 to 2 orders of magnitude faster than those reported for batch processes.
Experimental section
General experimental conditions
1H-NMR spectra were recorded on a Bruker 300 MHz instrument. 13C-NMR spectra were recorded on the same instrument at 75 MHz. Chemical shifts (δ) are expressed in ppm downfield from TMS as internal standard. The letters s, d, t, q, and m are used to indicate singlet, doublet, triplet, quadruplet, and multiplet. High-resolution mass spectra were recorded on a FT-ICR-MS instrument using electrospray ionization (ESI) in positive mode. Low resolution mass spectra were obtained on a LC-MS instrument using electrospray ionization (ESI) in positive or negative mode (Shimadzu LCMS-2020). GC-MS spectra were recorded using a Thermo Focus GC coupled with a Thermo DSQ II (EI, 70 eV). A HP5-MS column (30 m × 0.250 mm × 0.025 μm) was used with helium as carrier gas (1 mL min−1 constant flow). The injector temperature was set to 280 °C. After 1 min at 50 °C the temperature was increased in 25 °C min−1 steps up to 300 °C and kept at 300 °C for 4 minutes. Analytical HPLC (Shimadzu LC20) analysis was carried out on a C18 reversed-phase (RP) analytical column (150 × 4.6 mm, particle size 5 μm) at 25 °C using a mobile phase A (water/acetonitrile 90
:
10 (v/v) + 0.1% TFA) and B (MeCN + 0.1% TFA) at a flow rate of 1.0 mL min−1. The following gradient was applied: linear increase from solution 30% B to 100% B in 8 min, hold at 100% solution B for 2 min. Melting points were determined on a Stuart™ SMP3 melting point apparatus. Reagents and solvents were purchased from commercial sources and were used without further purification.
Caution: Carbon monoxide is a highly poisonous, colourless, odourless and tasteless gas. Reactions have to be performed in an efficient fume hood. Excess carbon monoxide should be directly vented into the exhaust duct of the fume hood. Carbon monoxide detectors must be used inside and outside the fume hood at all times!
General procedure for indole synthesis (Table 5)
The indole synthesis was performed in the flow setup shown in Table 3. The reactor consisted of an HPLC pump for introducing the liquid feed (Uniqsis FlowSyn). Carbon monoxide gas from a gas cylinder (N18, 98% CO) was fed into the system via a calibrated mass flow controller (Bronkhorst). The liquid and gaseous streams were combined in a glass static mixer at 140 °C (GSM 2 mL, Uniqsis). The mixer was connected to the residence tube reactor via a fluoropolymer tubing (PFA, 1/16′′ o.d., 0.8 mm i.d.). The residence coil reactor (stainless steel, 1/6′′ o.d., 1.0 mm i.d., 20 mL) was heated on a heating block to 140 °C (Uniqsis FlowSyn). The reaction mixture exited the system through a short cooling loop and an adjustable back-pressure regulator (Swagelok KCB1H0A2A5P60000, 0–26 bar). The reaction mixtures consisted of 0.9 mmol of stilbene, 2 mol% Pd(OAc)2, 4 mol% of phenanthroline and 40 mol% of tributylamine in 3 mL of MeCN (HPLC grade) as solvent. The mixtures were loaded into a 5 mL injection loop and injected from the loop into the reactor at flow rates of the liquid stream of 0.5 mL min−1 and a flow rate of the CO stream of 8.0 mL min−1. The total residence time was 15 min (determined by a stopwatch). Some starting materials were reprocessed under the same reaction conditions (see Table 5). Indole 1n immediately crystallized from the mixture after the continuous flow reaction and was isolated by simple filtration. Indole 1b was isolated by column chromatography on silica gel using ethyl acetate and petroleum ether as eluent. For the other indoles, the solvent was evaporated. The remaining material dissolved in hot boiling petroleum ether and palladium was removed by filtration. After cooling to −10 °C, the obtained crystals were filtered off, washed with cold chloroform and dried in a drying oven at 50 °C.
Methyl 1H-indole-2-carboxylate (2a). Colorless crystals, mp 150–151 °C (lit.21 mp 151–152 °C), 81% yield (127 mg, 0.73 mmol); 1H NMR (300 MHz, DMSO-d6) δ 11.94 (brs, 1H), 7.66 (dd, J = 8.0, 0.7 Hz, 1H), 7.46 (dd, J = 8.3, 0.9 Hz, 1H), 7.29–7.24 (m, 1H), 7.17–7.16 (m, 1H), 7.11–7.05 (m, 1H), 3.88 (s, 3H). 13C NMR (75 MHz, DMSO-d6) δ 162.2, 137.8, 127.5, 127.2, 125.1, 122.5, 120.6, 113.0, 108.2, 52.3. MS (EI): m/z: 175 [M]+.
4-(6-(Trifluoromethyl)-1H-indol-2-yl)benzonitrile (2b). White crystals, mp 201–202 °C, 80% yield (205 mg, 0.71 mmol); 1H NMR (300 MHz, acetone-d6) δ 11.18 (brs, 1H), 8.00–7.92 (m, 2H), 7.79–7.72 (m, 2H), 7.68 (d, J = 8.4 Hz, 1H), 7.65 (d, J = 0.7 Hz, 1H), 7.22 (dd, J = 8.4, 1.2 Hz, 1H), 7.13–7.07 (m, 1H). 13C NMR (75 MHz, acetone-d6) δ 139.0, 136.7, 136.0, 132.9, 131.4, 126.0, 125.4 (q, J = 270.6 Hz), 124.0 (q, J = 31.8 Hz), 121.5, 118.3, 116.6 (q, J = 3.5 Hz), 111.1, 108.9 (q, J = 5.1 Hz), 101.7; 19F NMR (282 MHz, acetone-d6) δ −61.20 (s), MS (EI): m/z: 286 [M]+, HRMS (ESI): calc. for C16H10F3N2+ 285.0645 [M + H]+, found 285.0643.
2-Phenyl-6-(trifluoromethyl)-1H-indole (2c). White crystals, mp 181–182 °C (lit.22 mp 181–182 °C), 90% yield (211 mg, 0.81 mmol); 1H NMR (300 MHz, CDCl3) δ 8.56 (brs, 1H), 7.77–7.68 (m, 4H), 7.54–7.47 (m, 2H), 7.44–7.36 (m, 2H), 6.90–6.89 (m, 1H). 13C NMR (75 MHz, CDCl3) δ 140.6, 135.6, 131.6, 129.2, 128.5, 125.4, 125.2 (q, J = 271.5 Hz), 124.2 (q, J = 31.9 Hz), 120.9, 117.0 (q, J = 3.5 Hz), 108.4 (q, J = 4.1 Hz), 100.0. MS (EI): m/z: 260 [M]+. 19F NMR (282 MHz, CDCl3) δ −60.93.
2-(4-(tert-Butyl)phenyl)-6-(trifluoromethyl)-1H-indole (2d). White crystals, mp 107–108 °C, 85% yield (242 mg, 0.76 mmol); 1H NMR (300 MHz, CDCl3) δ 8.68 (brs, 1H), 7.73–7.68 (m, 2H), 7.65 (d, J = 8.5 Hz, 2H), 7.52 (d, J = 8.5 Hz, 2H), 7.37 (d, J = 9.2 Hz, 1H), 6.86 (m, 1H), 1.39 (s, 9H). 13C NMR (75 MHz, CDCl3) δ 151.7, 140.7, 135.5, 131.7, 128.8, 126.1, 125.2, 125.2 (q, J = 271.4 Hz), 123.9 (q, J = 31.8 Hz), 120.7, 116.8, 108.3, 99.5, 34.7, 31.2. 19F NMR (282 MHz, CDCl3) δ −60.87. MS (EI): m/z: 317 [M]+, HRMS (ESI): calc. for C19H19F3N+ 316.1319 [M + H]+, found 316.1316.
2-(4-Fluorophenyl)-6-(trifluoromethyl)-1H-indole (2e). White crystals, mp 121–122 °C, 83% yield (208 mg, 0.75 mmol); 1H NMR (300 MHz, acetone-d6) δ 10.97 (brs, 1H), 7.83–7.76 (m, 2H), 7.63–7.59 (m, 2H), 7.21–7.09 (m, 3H), 6.85 (d, J = 1.3 Hz, 1H). 13C NMR (75 MHz, CDCl3) δ 162.8 (d, J = 248.8 Hz), 140.0, 135.6, 131.5, 127.9 (d, J = 3.3 Hz), 127.2 (d, J = 8.2 Hz), 125.1 (q, J = 271.5 Hz), 124.2 (q, J = 32.0 Hz), 120.9, 117.1 (q, J = 3.4 Hz), 116.24 (d, J = 21.9 Hz), 108.4 (q, J = 4.0 Hz), 100.0. 19F NMR (282 MHz, acetone-d6) δ −60.94 (3H), −114.91 (1H). MS (EI): m/z: 279 [M]+.
4-(1H-Indol-2-yl)benzonitrile (2f). White crystals, mp 190–192 °C (lit.23 mp 190–191 °C), 90% yield (176 mg, 0.81 mmol); 1H NMR (300 MHz, DMSO-d6) δ 11.76 (brs, 1H), 8.07–8.05 (m, 2H), 7.93–7.90 (m, 2H), 7.58 (d, J = 7.9 Hz, 1H), 7.44 (dd, J = 8.1, 0.8 Hz, 1H), 7.19–7.14 (m, 2H), 7.06–7.01 (m, 1H). 13C NMR (75 MHz, DMSO-d6) δ 138.1, 137.0, 136.0, 133.3, 128.8, 125.8, 123.2, 121.1, 120.3, 119.5, 112.1, 109.6, 102.0. MS (EI): m/z: 218 [M]+.
2-Phenyl-1H-indole (2g). White crystals, mp 190–191 °C (lit.22 mp 186–187 °C), 70% yield (121 mg, 0.63 mmol), 1H NMR (300 MHz, acetone-d6) δ 10.5 (brs, 1H), 7.75–7.69 (m, 2H), 7.43 (d, J = 7.9 Hz, 1H), 7.34–7.25 (m, 3H), 7.20–7.13 (m, 1H), 7.00–6.93 (m, 1H), 6.88 (td, J = 7.5, 1.1 Hz, 1H), 6.77–6.75 (m, 1H). 13C NMR (75 MHz, acetone-d6) δ 137.9, 137.5, 132.7, 129.3, 128.9, 127.4, 125.0, 121.8, 120.2, 119.6, 111.1, 99.1. MS (EI): m/z: 193 [M]+.
4-(6-(Dimethylamino)-1H-indol-2-yl)benzonitrile (2j). Yellow needles, mp 220–221 °C, 74% yield (174 mg, 0.67 mmol); 1H NMR (300 MHz, acetone-d6) δ 10.29 (brs, 1H), 7.82–7.77 (m, 2H), 7.64–7.59 (m, 2H), 7.29 (d, J = 8.7 Hz, 1H), 6.86–6.82 (m, 1H), 6.64–6.58 (m, 1H), 6.56–6.55 (m, 1H), 2.81 (s, 6H). 13C NMR (75 MHz, acetone-d6) δ 148.8, 140.0, 137.3, 133.4, 132.6, 124.4, 121.2, 121.2, 118.8, 109.9, 108.8, 102.1, 93.8, 40.6. MS (pos-ESI): m/z: 260 [M + H]+, HRMS (ESI): calc. for C17H16N3+ 260.1193 [M + H]+, found 260.1191.
4-(5-Chloro-1H-indol-2-yl)benzonitrile (2n). White crystals, mp 198–200 °C, 40% yield (90 mg, 0.36 mmol); 1H NMR (300 MHz, acetone-d6) δ 10.93 (brs, 1H), 7.94–7.89 (m, 2H), 7.75–7.70 (m, 2H), 7.50 (d, J = 2.0 Hz, 1H), 7.32 (d, J = 8.7 Hz, 1H), 7.02 (dd, J = 8.7, 2.0 Hz, 1H), 6.97 (s, 1H). 13C NMR (75 MHz, acetone-d6) δ 137.5, 136.4, 136.3, 132.8, 130.1, 125.7, 125.2, 123.0, 119.9, 118.4, 112.9, 110.7, 101.3. MS (EI): m/z: 252 [M]+.
5-Chloro-2-phenyl-1H-indole (2o). White crystals, mp 196–197 °C (lit.24 mp 195–196 °C), 45% yield (91 mg, 0.40 mmol); 1H NMR (300 MHz, acetone-d6) δ 10.73 (brs, 1H), 7.76–7.70 (m, 2H), 7.44 (d, J = 2.0 Hz, 1H), 7.37–7.18 (m, 4H), 6.96 (dd, J = 8.6, 2.1 Hz, 1H), 6.76 (d, J = 2.2 Hz, 1H). 13C NMR (75 MHz, acetone-d6) δ 139.7, 135.9, 132.1, 130.4, 129.0, 127.8, 125.2, 124.8, 121.7, 119.4, 112.5, 98.7. MS (EI): m/z: 227[M]+.
5-Chloro-2-(4-fluorophenyl)-1H-indole (2p). White crystals, mp 165–166 °C (lit.25 mp 167–168 °C), 80% yield (176 mg, 0.72 mmol); 1H NMR (300 MHz, acetone-d6) δ 10.72 (brs, 1H), 7.80–7.73 (m, 2H), 7.44 (d, J = 2.0 Hz, 1H), 7.28 (d, J = 8.6 Hz, 1H), 7.16–7.07 (m, 2H), 6.96 (dd, J = 8.6, 2.1 Hz, 1H), 6.73 (s, 1H). 13C NMR (75 MHz, acetone-d6) δ 162.4 (d, J = 245.7 Hz), 138.8, 135.9, 130.4, 128.7, 127.3 (d, J = 8.2 Hz), 124.8, 121.8, 119.3, 115.8 (d, J = 21.9 Hz), 112.5 (d, J = 3.8 Hz), 98.7. 19F NMR (282 MHz, acetone-d6) δ −115.57. MS (EI): m/z: 244 [M]+.
Acknowledgements
Funding from NAWI Graz and Research Center Pharmaceutical Engineering (RCPE) for the ReactIR system is gratefully acknowledged. We thank Prof. W. Goessler (Graz University) for performing ICPMS analysis.
Notes and references
- For reviews on recent advances in the field of indole synthesis, see:
(a) D. F. Taber and P. K. Tirunahari, Tetrahedron, 2011, 67, 7195–7210 CrossRef CAS PubMed;
(b) G. R. Humphrey and J. T. Kuethe, Chem. Rev., 2006, 106, 2875–2911 CrossRef CAS PubMed.
-
(a) R. J. Sundberg and T. Yamazaki, J. Org. Chem., 1967, 32, 290–294 CrossRef CAS;
(b) R. J. Sundberg, J. Org. Chem., 1965, 30, 3604–3610 CrossRef CAS;
(c) J. I. G. Cadogan, Synthesis, 1969, 1, 11–17 CrossRef CAS;
(d) J. I. G. Cadogan, Organo-phosphorus Reagents Org. Synth., 1979, 269–294 CAS;
(e) For a recent example in synthesis, see: A. S. Cotterill, C. J. Moody and J. R. A. Roffey, Tetrahedron, 1995, 51, 7223–7230 CrossRef CAS.
- For early reports, see:
(a) C. Crotti, S. Cenini, B. Rindone, S. Tollari and F. Demartinc, J. Chem. Soc., Chem. Commun., 1986, 784–786 RSC;
(b) C. Crotti, S. Cenini, R. Todeschini and S. Tollari, J. Chem. Soc., Faraday Trans., 1991, 87, 2811–2820 RSC;
(c) M. Pizzotti, S. Cenini, P. Psaro and S. Costanzi, J. Mol. Catal., 1990, 63, 299–304 CrossRef CAS;
(d) C. Crotti, S. Cenini, F. Ragaini, F. Porta and S. Tollari, J. Mol. Catal., 1992, 72, 283–298 CrossRef CAS.
-
(a) M. Akazome, T. Kondo and Y. Watanabe, Chem. Lett., 1992, 769–772 CrossRef CAS;
(b) M. Akazome, T. Kondo and Y. Watanabe, J. Org. Chem., 1994, 59, 3375–3380 CrossRef CAS.
- B. C. Söderberg and J. A. Shriver, J. Org. Chem., 1997, 62, 5838–5845 CrossRef.
- For further examples using Pd(OAc)2/ligand as catalyst, see:
(a) B. C. Söderberg, A. C. Chisnell, S. N. O'Neil and J. A. Shriver, J. Org. Chem., 1999, 64, 9731–9734 CrossRef;
(b) B. C. G. Söderberg, S. R. Banini, M. R. Turner, A. R. Minter and A. K. Arrington, Synthesis, 2008, 6, 903–912 CrossRef;
(c) B. C. G. Söderberg, J. W. Hubbard, S. R. Rector and S. N. O'Neil, Tetrahedron, 2005, 61, 3637–3649 CrossRef;
(d) B. C. Söderberg, S. R. Rector and S. N. O'Neil, Tetrahedron Lett., 1999, 40, 3657–3660 CrossRef;
(e) T. L. Scott and B. C. G. Söderberg, Tetrahedron, 2003, 59, 6323–6332 CrossRef CAS;
(f) N. H. Ansari, C. A. Dacko, N. G. Akhmedov and B. C. G. Söderberg, J. Org. Chem., 2016, 81, 9337–9349 CrossRef CAS PubMed.
-
(a) T. L. Scott and B. C. G. Söderberg, Tetrahedron Lett., 2002, 43, 1621–1624 CrossRef CAS;
(b) R. W. Clawson Jr, R. E. Deavers, N. G. Akhmedov and B. C. G. Söderberg, Tetrahedron, 2006, 62, 10829–10834 CrossRef.
- I. W. Davies, J. H. Smitrovich, R. Sidler, C. Qu, V. Gresham and C. Bazaral, Tetrahedron, 2005, 61, 6425–6437 CrossRef CAS.
-
(a) F. Zhou, D.-S. Wang and T. G. Driver, Adv. Synth. Catal., 2015, 357, 3463–3468 CrossRef CAS;
(b) N. Jana, F. Zhou and T. G. Driver, J. Am. Chem. Soc., 2015, 137, 6738–6741 CrossRef CAS PubMed.
- For extensive treatises on microreactor and continuous flow technology, see:
(a) Flow Chemistry, ed. F. Darvas, V. Hessel and G. Dorman, De Gruyter, Berlin, 2014 Search PubMed;
(b) Microreactors in Preparative Chemistry, ed. W. Reschetilowski, Wiley-VCH, Weinheim, 2013 Search PubMed;
(c) Microreactors in Organic Synthesis and Catalysis, ed. T. Wirth, Wiley-VCH, Weinheim, 2nd edn, 2013 Search PubMed;
(d) V. Hessel, J. C. Schouten, A. Renken, Y. Wang, and J.-i. Yoshida, Handbook of Micro Reactors, Wiley-VCH, Weinheim, 2009 Search PubMed;
(e) Chemical Reactions and Processes under Flow Conditions, ed. S. V. Luis and E. Garcia-Verdugo, RSC Green Chemistry, 2010 Search PubMed.
- For recent reviews on continuous flow chemistry, see:
(a) C. Wiles and P. Watts, Green Chem., 2014, 16, 55–62 RSC;
(b) S. V. Ley, D. E. Fitzpatrick, R. J. Ingham and R. M. Myers, Angew. Chem., Int. Ed., 2015, 54, 3449–3464 CrossRef CAS PubMed;
(c) B. Gutmann, D. Cantillo and C. O. Kappe, Angew. Chem., Int. Ed., 2015, 54, 6688–6728 CrossRef CAS PubMed;
(d) V. Hessel, D. Kralisch, N. Kockmann, T. Noël and Q. Wang, ChemSusChem, 2013, 6, 746–789 CrossRef CAS PubMed;
(e) J. C. Pastre, D. L. Browne and S. V. Ley, Chem. Soc. Rev., 2013, 42, 8849–8869 RSC;
(f) D. T. McQuade and P. H. Seeberger, J. Org. Chem., 2013, 78, 6384–6389 CrossRef CAS PubMed;
(g) M. Movsisyan, E. I. P. Delbeke, J. K. E. T. Berton, C. Battilocchio, S. V. Ley and C. V. Stevens, Chem. Soc. Rev., 2016, 45, 4892–4928 RSC.
- For recent reviews on continuous flow gas–liquid reactions, see:
(a) C. J. Mallia and I. R. Baxendale, Org. Process Res. Dev., 2016, 20, 327–360 CrossRef CAS;
(b) H. P. Gemoets, Y. Su, M. Shang, V. Hessel, R. Luque and T. Noël, Chem. Soc. Rev., 2016, 45, 83–117 RSC;
(c) B. Pieber and C. O. Kappe, Top. Organomet. Chem., 2016, 57, 97–136 CrossRef.
- For recent examples of reactions with CO gas in tubular flow reactors or microreactors, see:
(a) Md. T. Rahman, T. Fukuyama, N. Kamata, M. Sato and I. Ryu, Chem. Commun., 2006, 2236–2238 RSC;
(b) E. R. Murphy, J. R. Martinelli, N. Zaborenko, S. L. Buchwald and K. F. Jensen, Angew. Chem., Int. Ed., 2007, 46, 1734–1737 CrossRef CAS PubMed;
(c) X. Gong, P. W. Miller, A. D. Gee, N. J. Long, A. J. Mello and R. Vilar, Chem.–Eur. J., 2012, 18, 2768–2772 CrossRef CAS PubMed;
(d) P. W. Miller, N. J. Long, A. J. de Mello, R. Vilar, J. Passchier and A. Gee, Chem. Commun., 2006, 546–548 RSC;
(e) P. W. Miller, N. J. Long, A. J. de Mello, R. Vilar, H. Audrain, D. Bender, J. Passchier and A. Gee, Angew. Chem., Int. Ed., 2007, 46, 2875–2878 CrossRef CAS PubMed;
(f) P. W. Miller, L. E. Jennings, A. J. deMello, A. D. Gee, N. J. Long and R. Vilar, Adv. Synth. Catal., 2009, 351, 3260–3268 CrossRef CAS.
- For examples of reactions with CO using “tube-in-tube” reactors as gas addition tools, see:
(a) C. J. Mallia, G. C. Walter and I. R. Baxendale, Beilstein J. Org. Chem., 2016, 12, 1503–1511 CrossRef CAS PubMed;
(b) M. A. Mercadante and N. E. Leadbeater, Org. Biomol. Chem., 2011, 9, 6575–6578 RSC;
(c) C. Brancour, T. Fukuyama, Y. Mukai, T. Skrydstrup and I. Ryu, Org. Lett., 2013, 15, 2794–2797 CrossRef CAS PubMed;
(d) T. Fukuyama, Y. Mukai and I. Ryu, Beilstein J. Org. Chem., 2011, 7, 1288–1293 CrossRef CAS PubMed;
(e) U. Gross, P. Koos, M. O'Brien, A. Polyzos and S. V. Ley, Eur. J. Org. Chem., 2014, 6418–6430 CrossRef CAS.
- Ley and co-workers previously used the CO band for the in-line determination of CO concentration in a continuous flow system: P. Koos, U. Gross, A. Polyzos, M. O'Brien, I. Baxendale and S. V. Ley, Org. Biomol. Chem., 2011, 9, 6903–6908 CAS.
- Reduction of Pd(OAc)2 with CO was previously used in Heck reactions to generate Pd(0):
(a) F. A. Siqueira, J. G. Taylor and C. R. D. Correia, Tetrahedron Lett., 2010, 51, 2102–2105 CrossRef CAS;
(b) A. H. L. Machado, M. A. de Sousa, D. C. S. Patto, L. F. S. Azevedo, F. I. Bombonato and C. R. D. Correia, Tetrahedron Lett., 2009, 50, 1222–1225 CrossRef CAS.
- I. W. Davies, V. A. Guner and K. N. Houk, Org. Lett., 2004, 6, 743–746 CrossRef CAS PubMed.
- E. Ragaini, S. Cenini and F. Demartin, J. Organomet. Chem., 1999, 586, 190–195 CrossRef , and references therein.
- E. Sinkovec and M. Krajnc, Org. Process Res. Dev., 2011, 15, 817–823 CrossRef CAS , and references therein.
- The limit for the total amount of platinoid-group metals is 5 mg kg−1 (for dosages of up to 10 g per day). Guideline on the specification limits for residues of metal catalysts from the European Agency for the Evaluation of Medicinal Products (EMEA), 2007 (http://www.emea.europa.eu).
- L. Angelo, O. Rosaria, R. Giovanni, S. Giovanni and U. Nicola, Heterocycles, 1988, 27, 1365–1376 CrossRef.
- W. Huifeng, L. Yaming, J. Linlin, Z. Rong, J. Kun, Z. Defeng and D. Chunying, Org. Biomol. Chem., 2011, 9, 4983–4986 Search PubMed.
- A. M. Gary, C. Belgin and E. K. Lauren, J. Org. Chem., 2009, 74, 973–980 CrossRef PubMed.
- J. H. William, A. P. Vincent and U. Yasuyuki, J. Org. Chem., 1981, 46, 4511–4515 CrossRef.
- F. Da Settimo, F. Simorini, S. Taliani, C. La Motta, A. M. Marini, S. Salerno, M. Bellandi, E. Novellino, G. Greco, B. Cosimelli, E. Da Pozzo, B. S. Costa, M. Nicola and M. M. Claudia, J. Med. Chem., 2008, 51, 5798–5806 CrossRef CAS PubMed.
Footnote |
† Electronic supplementary information (ESI) available: Further experimental results and optimization data; Wittig synthesis of o-nitrostilbenes and characterization data; ICPMS analysis of the processed reaction mixture; 1H-NMR and 13C-NMR spectra. See DOI: 10.1039/c7ra01087g |
|
This journal is © The Royal Society of Chemistry 2017 |
Click here to see how this site uses Cookies. View our privacy policy here.