DOI:
10.1039/C7RA01353A
(Paper)
RSC Adv., 2017,
7, 13879-13885
Enhancement of CO2 permeability of poly(vinyl ether)s having oxyethylene chains by the sequence control of crosslinking sites
Received
2nd February 2017
, Accepted 17th February 2017
First published on 1st March 2017
Abstract
Sequential living cationic copolymerizations of vinyl ethers having oxyethylene side chains (MOEO2VE and MOEO3VE) with vinyl ether having a crosslinkable group (VEEM) provided the ABA-typed triblock copolymers poly(VEEM)-b-poly(MOEO2VE)-b-poly(VEEM)s and poly(VEEM)-b-poly(MOEO3VE)-b-poly(VEEM)s. All triblock copolymers are sticky liquids at room temperature, and the Tgs were −77 to −73 °C. Heating the triblock copolymers afforded membranes by thermal crosslinking via mathacrylate groups at the outer segments in the polymers. All the membranes showed high CO2 permselectivity (PCO2/PN2 = 40–51) due to the high CO2 solubility selectivity (SCO2/SN2 = 44–61). The CO2 permeability of the triblock copolymers was higher than that of the random copolymers with the same composition ratios. This indicates that the inner segment (MOEO2VE and MOEO3VE) effectively enhanced the gas diffusivity in the polymer matrix because the crosslinking points are present only in the outer segments.
Introduction
Gas separation using polymer membranes becomes important due to the low costs and energy consumption compared with traditional separation techniques, such as adsorption and absorption.1–3 For CO2 separation from mixed gases, a variety of polymers containing oxyethylene chains were studied because oxyethylene chains have highly favorable interactions with CO2 molecules and preferentially dissolve CO2 molecules.4 For example, poly(ethylene oxide) exhibits high CO2 permselectivity (PCO2/PN2 = 50), but the CO2 permeability is low (PCO2 = 12 barrer).5 Gas molecules generally go through amorphous regions in polymer matrices. The high crystallinity of poly(ethylene oxide) interferes with the diffusion of gases in the membrane. To enhance the gas permeability, an introduction of short oxyethylene chains to the rigid polymers and a crosslinking between short oxyethylene chains are investigated.6–12 For instance, the crosslinked membranes prepared from low-molecular-weight poly(ethylene glycol) diacrylate show high CO2 permeability and high CO2/N2 selectivity (PCO2 = 100–150 barrer, PCO2/PN2 = 45–65) [6–10]. The PCO2 and PCO2/PN2 values of poly(ether imide)s bearing amorphous poly(ethylene oxide) are 100–150 barrer and 45–65, respectively.11
We have been studying the membranes of poly(vinyl ether)s for CO2 separation.13–17 Among them, poly(vinyl ether)s having oxyethylene chains are wholly amorphous and have high content of oxyethylene units, and therefore they exhibit high gas permeability and high CO2 permselectivity. However, the homopolymers of vinyl ethers having oxyethylene chains are sticky liquid, whose Tg's are −50 to −20 °C. The copolymerization with other vinyl ethers such as 2-admantyl vinyl ether and vinyloxy ethoxy ethyl methacrylate [VEEM] is needed for the preparation of polymer membranes.14–17 For example, the copolymers of vinyl ether having oxyethylene chain with VEEM are heated in bulk to afford the membranes of crosslinked polymers by the reaction of methacrylate side groups. We reported that the gas permeability increased as the composition rate of VEEM in the copolymer decreased because the lack of crosslinking point enhanced the flexibility of polymer chains.15,17 The crosslinking of polymer chains is necessary to obtain membranes, but it decreases the gas permeability.
In the present study, we synthesized the triblock copolymers, poly(VEEM)-b-poly(MOEO2VE)-b-poly(VEEM) and poly(VEEM)-b-poly(MOEO3VE)-b-poly(VEEM), with various composition ratios (Scheme 1). The triblock copolymers have the crosslinkable groups only at the outer sides in the polymer chains, and therefore the sequence of poly(MOEO2VE) or poly(MOEO3VE) is predicted to make the polymer chains flexible compared to the random copolymer with the same composition. In consequence, the triblock copolymer may show high CO2 permeability.
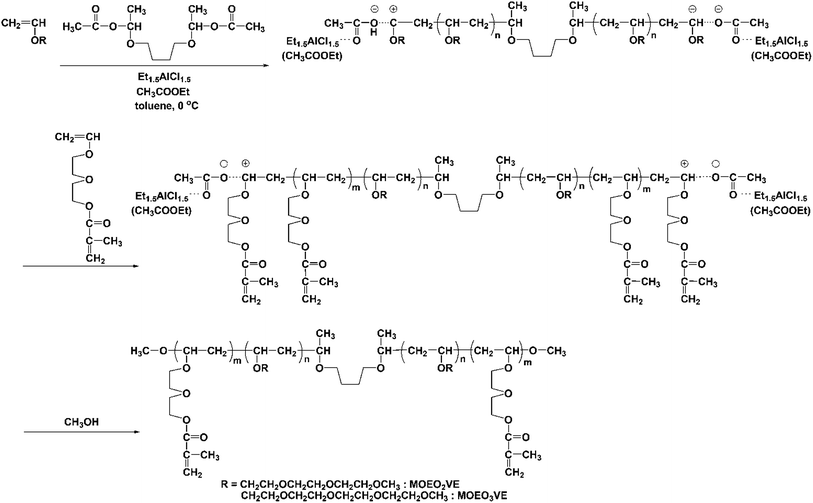 |
| Scheme 1 Synthesis of triblock copolymers, poly(VEEM)-b-poly(MOEO2VE)-b-poly(VEEM) and poly(VEEM)-b-poly(MOEO3VE)-b-poly(VEEM). | |
Experimental
Materials
Materials 2-[2-(2-methoxyethoxy)ethoxy]ethyl vinyl ether (MOEO2VE) was supplied by Maruzen Petrochemical Co. Ltd. 2-{2-[2-(2-Methoxyethoxy)ethoxy]ethoxy}ethyl vinyl ether (MOEO3VE) was synthesized by the reaction of 2-[2-(2-methoxyethoxy)ethoxy]ethyl methanesulfonate (CH3OCH2CH2OCH2CH2OCH2CH2OSO2CH3) with sodium salt of 2-hydroxyethyl vinyl ether (CH2
CH–O–CH2CH2–OH), which was supplied by Nippon Carbide Industries Co. Inc. The detailed procedure was reported in our previous work.15 2-(2-Vinyloxyethoxy)ethyl methacrylate (VEEM) was supplied by Nippon Shokubai Co. Ltd. and distilled over tert-butylcatechol under reduced pressure. 1,4-Bis(1-acetoxyethoxy)butane [CH3CH(OCOCH3)OCH2CH2CH2CH2OCH(OCOCH3)CH3: BAEB] was synthesized by the treatment of 1,4-butanediol divinyl ether with acetic acid at 60 °C and purified by distillation under reduced pressure three times over calcium hydride. Et1.5AlCl1.5 (1.82 M solution in toluene) was commercially obtained from Sigma-Aldrich Co. Ltd. and used without further purification. Toluene for polymerization was distilled twice over calcium hydride.
Polymerization
The polymerizations were carried out under a dry nitrogen atmosphere in a glass flask equipped with a three-way stopcock, which was baked at ca. 400 °C on a heat gun just before the reaction. The toluene solution of cationogen (BAEB) and Lewis acid (Et1.5AlCl1.5) were prepared in the separate tubes. The solution of BAEB was added to a MOEO2VE or MOEO3VE solution containing internal standard (tetrahydronaphthalene) for gas chromatography. The solution of Et1.5AlCl1.5 was added to the mixture at 0 °C. MOEO2VE or MOEO3VE was first polymerized, and then VEEM (neat) was added to the reaction mixture under the same conditions. Polymerization was terminated by adding excess amount of methanol (2.0 mL) containing a small amount of aqueous ammonia. Monomer conversion was determined from its residual concentration measured by gas chromatography. To work-up the polymer, the reaction mixture was diluted with CH2Cl2 and washed with 0.1 mol L−1 hydrochloric acid and 10 wt% aqueous sodium chloride in this order. The organic solution was concentrated by rotary evaporator and the resultant polymer was isolated by precipitation into a large excess of hexane.
Fabrication of composite membrane
Composite membranes were prepared using a porous Teflon film (Advantech). The pore size, porosity, and thickness are 10 μm, 80%, and 50 μm, respectively. The porous Teflon film was put on a Petri dish, and the toluene-solution of uncrosslinked polymer obtained by the polymerization was poured into the dish. The solvent was slowly evaporated at room temperature under atmospheric pressure for a few days. After evaporation of the solvent, the Petri dish was placed in an oven at 110 °C for 15 min. This heating led the crosslinking between polymer side chains. The formed membrane of crosslinking polymer with Teflon film was cooled to room temperature and peeled from the dish. The membrane was dried at room temperature under reduced pressure for 24 h.
Measurements
The molecular weight distributions (MWDs) of polymers were measured by gel permeation chromatography (GPC) in chloroform at 40 °C at 1.0 mL min−1 flow rate on a Shimadzu LC-10AD chromatograph equipped with three polystyrene gel columns (Shodex K-807L, K-805L, and K-804L) and a Shimadzu RID-6A refractive index detector. The number-average molecular weight (Mn) and polydispersity ratio (Mw/Mn) were calculated from chromatograms based on a polystyrene calibration. 1H NMR (500 MHz) spectra were recorded on Jeol ECX-500 instrument in CDCl3 at room temperature. Scanning electron microscopy (SEM) was measured with HITACHI-HITEC S-3400N. Gas permeability coefficients were measured with a Tsukubarikaseiki K-315-N gas permeability apparatus at 25 °C under 1 atm upstream pressure using dry gases. The permeability coefficient (P) expressed in barrer unit (1 barrer = 10−10 cm3(STP) cm cm−2 s−1 cmHg−1) was calculated from the slope of the steady-state line. The diffusivity coefficient (D) was determined by the time lag method using the following equation:here, l is the membrane thickness and θ is the time lag, which is given by the intercept of the asymptotic line of time-pressure carve to the time axis. The solubility coefficient (S) was calculated by using the following equation:
Results and discussion
Polymerization
In our present study, the random copolymerization of MOEO3VE with VEEM proceeded in the living manner using isobutyl vinyl ether-acetic acid adduct (CH3CH(OiBu)OCOCH3) as an initiator, Et1.5AlCl1.5 as a Lewis acid catalyst, and CH3COOEt as an added base in toluene at 0 °C.17,18 In the present study, MOEO2VE was first polymerized using BAEB as a bifunctional initiator, Et1.5AlCl1.5, and CH3COOEt in toluene at 0 °C to synthesize the triblock copolymer. Then, VEEM was added to the reaction mixture and polymerized. Fig. 1 shows the GPC traces of the products obtained by the polymerization with the different monomer feed ratios. As shown in Fig. 1, all the MWD curves of the products showed the formation of the triblock copolymers, poly(VEEM)-b-poly(MOEO2VE)-b-poly(VEEM), by the sequential polymerization described above. The peaks of the homopolymers shifted to the high-molecular-weight region by adding the second monomer, VEEM, with relatively narrow molecular weight distribution. No homopolymers peaks were observed in the MWD curves of the triblock copolymers, but other peaks appeared in higher-molecular-weight region (Mn > 1 × 106) especially in the polymerization with high feed ratio of VEEM. These products seem to be formed by the intermolecular reaction through mathacrylate units of VEEMs, but all the triblock copolymers were totally soluble in polymerization solvents. Fig. 2 shows the GPC curves of the products obtained by the sequential polymerizations of MOEO3VE and VEEM at various monomer feed ratios. Similarly to the case of MOEO2VE and VEEM, the sequential polymerizations produced the triblock copolymers, poly(VEEM)-b-poly(MOEO3VE)-b-poly(VEEM), with relatively narrow molecular weight distributions. High-molecular-weight product formed by the intermolecular reaction was present in the polymerization with 2
:
1 monomer feed ratio. All the copolymers were soluble in toluene, chloroform, tetrahydrofuran, dichloromethane, etc. They did not dissolve in hexane and water.
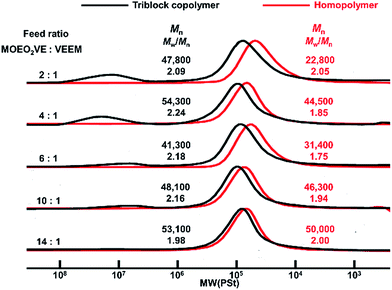 |
| Fig. 1 GPC traces of the products: synthesis of poly(VEEM)-b-poly(MOEO2VE)-b-poly(VEEM) with BAEB/Et1.5AlCl1.5/CH3COOEt in toluene at 0 °C for 24 h: [monomer]0,total = 1.2 M, [BAEB]0 = 1.0 mM, [Et1.5AlCl1.5]0 = 25 mM, [CH3COOEt]0 = 1.0 M. | |
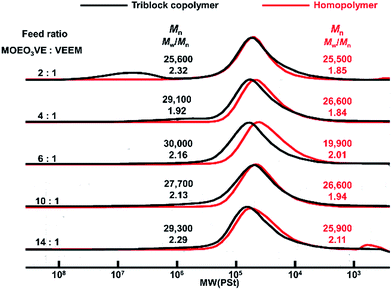 |
| Fig. 2 GPC traces of the products: synthesis of poly(VEEM)-b-poly(MOEO3VE)-b-poly(VEEM) with BAEB/Et1.5AlCl1.5/CH3COOEt in toluene at 0 °C for 24 h: [monomer]0,total = 1.2 M, [BAEB]0 = 1.0 mM, [Et1.5AlCl1.5]0 = 25 mM, [CH3COOEt]0 = 1.0 M. | |
Fig. 3 shows 1H NMR spectra of poly(VEEM)-b-poly(MOEO2VE)-b-poly(VEEM) and poly(VEEM)-b-poly(MOEO3VE)-b-poly(VEEM) obtained by the sequential polymerizations at the 2
:
1 monomer feed ratio. The peaks assignable to methacrylate group were observed at 6.12 and 5.58 ppm, and the intensity ratio of the signal at 6.12 ppm for methacrylate group to the signal at 4.27 ppm for the methylene adjacent to the ester oxygen was 1 to 2, which suggests that methacrylate groups of VEEM hardly react during cationic polymerization although the products obtained by the intermolecular reaction were observed in GPC chromatograms. The copolymer compositions were determined by the peak intensity ratio of the signal for the methylene adjacent to the ester oxygen to the signals for the main chain methine and the pendant methylene and methyl group adjacent to the ether oxygen. For the poly(VEEM)-b-poly(MOEO2VE)-b-poly(VEEM), the peak intensity of the signal for the methylene (f) was compared with the peak intensity of the signals for the main chain methine (a, i), pendant methylene (c–e, k–p), and methyl group (q). For the poly(VEEM)-b-poly(MOEO3VE)-b-poly(VEEM), the peak intensity of the signal for the methylene (f) was compared with the peak intensity of the signals for the main chain methine (a, i), pendant methylene (c–e, k–r), and methyl group (s). The composition ratios calculated from 1H NMR spectra corresponded well to the monomer feed ratios.
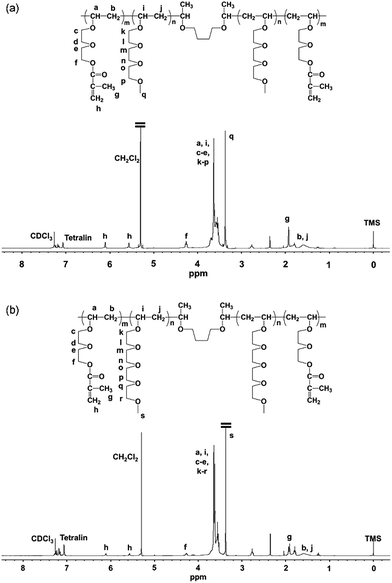 |
| Fig. 3 1H NMR spectra of (a) poly(VEEM)-b-poly(MOEO2VE)-b-poly(VEEM) (feed ratio 2 : 1) and (b) poly(VEEM)-b-poly(MOEO3VE)-b-poly(VEEM) (feed ratio 2 : 1). | |
Thermal crosslinking and thermal property
Fig. 4(a), (c), (e) and (g) shows the IR spectra of the triblock copolymers obtained by the polymerization. Fig. 4(b), (d), (f) and (h) shows the IR spectra of the triblock copolymers heated at 110 °C in bulk. The absorptions at 1620 cm−1 assigned to the stretching of C
C double bonds and at 1710 cm−1 assigned to the stretching of C
O carbonyl bonds were observed in all the spectra. However, the peak intensity of the C
C double bonds was decreased compared to the peak intensity of the absorption assigned to the stretching of C–O–C at 1080 cm−1 after heating. The conversion of C
C double bonds was calculated from the peak intensity at 1620 cm−1 on the basis of the peak at 1080 cm−1 attributed to C–O–C. The conversion of C
C double bonds calculated from the spectra (a) and (b) for poly(VEEM)-b-poly(MOEO2VE)-b-poly(VEEM) was approximately 50%. Those for the other triblock copolymers were in the range of 40–60%. The reaction between methacrylate groups in bulk did not proceed completely but reached around 50% conversion, whose result was the same as the case of the random copolymers, poly(MOEO3VE)-ran-poly(VEEM), reported previously.17
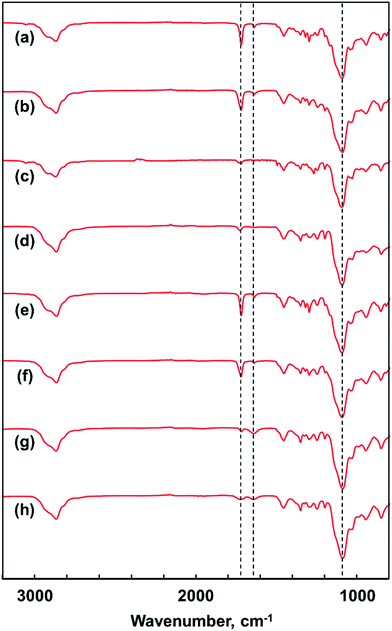 |
| Fig. 4 IR spectra (a) before and (b) after heating for poly(VEEM)-b-poly(MOEO2VE)-b-poly(VEEM) (composition ratio 2 : 1), (c) before and (d) after heating for poly(VEEM)-b-poly(MOEO2VE)-b-poly(VEEM) (composition ratio 14 : 1), (e) before and (f) after heating for poly(VEEM)-b-poly(MOEO3VE)-b-poly(VEEM) (composition ratio 2 : 1), (g) before and (h) after heating for poly(VEEM)-b-poly(MOEO3VE)-b-poly(VEEM) (composition ratio 14 : 1). | |
Fig. 5 shows the DSC thermograms of poly(VEEM)-b-poly(MOEO2VE)-b-poly(VEEM) and poly(VEEM)-b-poly(MOEO3VE)-b-poly(VEEM). The prepared five poly(VEEM)-b-poly(MOEO2VE)-b-poly(VEEM)s exhibited Tgs of −77 to −74 °C, which were very close to Tg of −73 °C of the homopolymer, poly(MOEO2VE).16 The Tgs of poly(VEEM)-b-poly(MOEO3VE)-b-poly(VEEM)s were −75 to −73 °C, which were also similar to Tg of −72 °C of the homopolymer, poly(MOEO3VE).16 In both types of triblock copolymers, the Tgs were independent on the composition ratio of MOEO2VE/MOEO3VE and VEEM. The low Tg is advantage for gas separation membrane because the flexible polymer chains enhances the gas diffusivity in the polymer matrix.
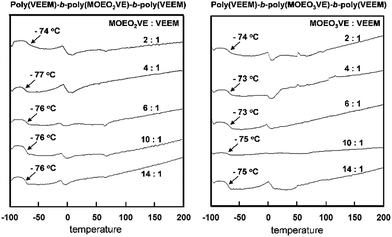 |
| Fig. 5 DSC thermograms of poly(VEEM)-b-poly(MOEO2VE)-b-poly(VEEM)s and poly(VEEM)-b-poly(MOEO3VE)-b-poly(VEEM)s. | |
Gas permeability of crosslinked membranes
Fig. 6 shows SEM images of the cross-section of porous PTFE film (a and b) and the representative composite membranes (c–f). PTFE film had a filament structure and a lot of pores were observed in Fig. 6(a) and (b). On the other hand, no pores were observed in Fig. 6(c)–(f). This indicates that the copolymers penetrated into the pores of PTFE film and all the composite membranes were non-porous membranes. Since the pore size of PTFE film is as large as 10 μm, poly(vinyl ether) can infiltrate into the pores. The composite membranes prepared in this study were smooth in surface and uniform in sickness.
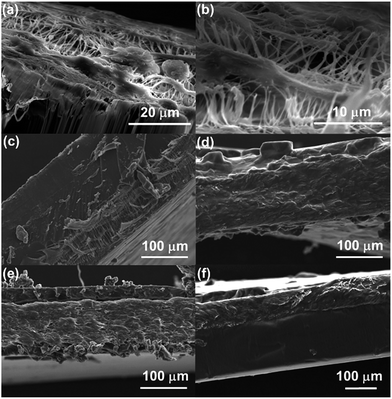 |
| Fig. 6 SEM images of the cross-section of (a) (b) porous PTFE film, (c) poly(VEEM)-b-poly(MOEO2VE)-b-poly(VEEM) (composition ratio 2 : 1), (d) poly(VEEM)-b-poly(MOEO2VE)-b-poly(VEEM) (composition ratio 14 : 1), (e) poly(VEEM)-b-poly(MOEO3VE)-b-poly(VEEM) (composition ratio 2 : 1), (f) poly(VEEM)-b-poly(MOEO3VE)-b-poly(VEEM) (composition ratio 14 : 1). | |
The gas permeability of the composite membranes was examined at 25 °C. Table 1 summarizes the nitrogen, oxygen, carbon dioxide permeability coefficients (PN2, PO2, PCO2) and separation factors (PO2/PN2, PCO2/PN2), along with the data for random copolymers of poly(MOEO3VE)-ran-poly(VEEM) for comparison. The gas permeability coefficients increased with the decreasing composition ratio of VEEM in the copolymers. For instance, the PCO2 values of poly(VEEM)-b-poly(MOEO2VE)-b-poly(VEEM) increased from 230 barrer to 420 barrer. The CO2 values of poly(VEEM)-b-poly(MOEO3VE)-b-poly(VEEM) increased from 280 barrer to 530 barrer. The low composition rates of VEEM means that the triblock copolymers had low crosslinking points. The lack of crosslinking points increases the polymer chain mobility. In the same composition ratio, the PCO2 values of poly(VEEM)-b-poly(MOEO3VE)-b-poly(VEEM) were higher than those of poly(VEEM)-b-poly(MOEO2VE)-b-poly(VEEM). From these results, it was found that the gas permeability increases with decreasing the composition ratio of VEEM and increasing the length of oxyethylene side chain. This tendency is the same as the case of random copolymers we reported in the previous study.15,17 Interestingly, the gas permeability of poly(VEEM)-b-poly(MOEO3VE)-b-poly(VEEM) was higher than that of random copolymer, poly(MOEO3VE)-ran-poly(VEEM), with the same composition ratios, indicating that the monomer sequence in the copolymer is essential to the gas permeability. The random copolymers are crosslinked randomly in the main chains, whereas the triblock copolymers are crosslinked at outer segments in the main chains. The images of the crosslinked structures are illustrated in Fig. 7. The main chains of the triblock copolymers may become more flexible than the random copolymers, which would result in higher gas permeability. The O2 separation factors (PO2/PN2) of the membranes were 2.3 to 2.9, which are ordinary values. On the other hand, the CO2 permselectivity of all the copolymers was very high, and the separation factors (PCO2/PN2) were 41 to 51.
Table 1 Gas permeability, diffusion, and solubility coefficients (Pa, Db, Sc) of membranes at 25 °C
Composition |
PN2 |
PO2 |
PCO2 |
PO2/PN2 |
PCO2/PN2 |
DN2 |
DCO2 |
SN2 |
SCO2 |
DCO2/DN2 |
SCO2/SN2 |
In the unit of barrer (1 barrer = 1 × 10−10 cm3(STP) cm cm−2 s−1 cmHg−1). In the units of 1 × 10−7 cm2 s−1. In the units of 1 × 10−3 cm3(STP) cm−3 cmHg−1. |
Poly(VEEM)-b-poly(MOEO2VE)-b-poly(VEEM) |
2 : 1 |
4.5 |
13 |
230 |
2.9 |
51 |
8.4 |
7.3 |
0.53 |
31 |
0.87 |
58 |
4 : 1 |
7.5 |
18 |
310 |
2.4 |
41 |
14 |
11 |
0.54 |
30 |
0.79 |
56 |
6 : 1 |
7.6 |
18 |
340 |
2.3 |
45 |
12 |
11 |
0.63 |
32 |
0.91 |
51 |
10 : 1 |
8.4 |
20 |
370 |
2.4 |
45 |
14 |
12 |
0.59 |
33 |
0.85 |
56 |
14 : 1 |
9.3 |
22 |
420 |
2.4 |
45 |
16 |
14 |
0.60 |
31 |
0.88 |
52 |
![[thin space (1/6-em)]](https://www.rsc.org/images/entities/char_2009.gif) |
Poly(VEEM)-b-poly(MOEO3VE)-b-poly(VEEM) |
2 : 1 |
6.6 |
17 |
280 |
2.6 |
42 |
12 |
11 |
0.48 |
26 |
0.92 |
54 |
4 : 1 |
8.1 |
21 |
340 |
2.6 |
43 |
16 |
14 |
0.49 |
25 |
0.88 |
51 |
6 : 1 |
9.4 |
24 |
430 |
2.6 |
46 |
17 |
15 |
0.57 |
28 |
0.88 |
49 |
10 : 1 |
12 |
29 |
500 |
2.4 |
42 |
21 |
19 |
0.58 |
26 |
0.90 |
45 |
14 : 1 |
12 |
30 |
530 |
2.5 |
44 |
21 |
20 |
0.57 |
27 |
0.95 |
47 |
![[thin space (1/6-em)]](https://www.rsc.org/images/entities/char_2009.gif) |
Poly(MOEO3VE)-ran-poly(VEEM) |
2 : 1 |
3.3 |
8.9 |
140 |
2.7 |
42 |
11 |
7.4 |
0.30 |
19 |
0.85 |
51 |
4 : 1 |
4.9 |
12 |
220 |
2.4 |
45 |
10 |
8.7 |
0.49 |
25 |
0.93 |
55 |
6 : 1 |
6.7 |
17 |
300 |
2.5 |
45 |
18 |
13 |
0.37 |
23 |
0.86 |
55 |
10 : 1 |
7.9 |
19 |
360 |
2.4 |
46 |
21 |
15 |
0.38 |
24 |
0.88 |
52 |
14 : 1 |
9.2 |
23 |
420 |
2.5 |
46 |
18 |
16 |
0.51 |
26 |
0.89 |
57 |
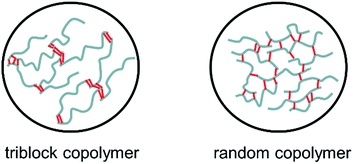 |
| Fig. 7 Possible crosslinked structures of triblock-copolymer and random-copolymer. | |
Gas permeability of non-porous polymer membranes can be divided into the diffusion and solution terms theoretically in the case of rubbery polymers. To inspect the gas permeability of the composite membranes in detail, the gas diffusion and solubility coefficients were estimated by time-lag method. Table 1 lists the diffusion and solubility coefficients (D and S) of the triblock and random copolymers. The SCO2 values of poly(VEEM)-b-poly(MOEO2VE)-b-poly(VEEM) and poly(VEEM)-b-poly(MOEO3VE)-b-poly(VEEM) were 25 to 33 cm3(STP) cm−3 cmHg−1, and the SCO2/SN2 values were as large as 45 to 58. The high solubility selectivity for CO2 caused the high CO2 permselectivity of the triblock copolymers. The D values increased with the decreasing composition ratio of VEEM in the same manner as the gas permeability coefficients. The DCO2 value of poly(VEEM)-b-poly(MOEO2VE)-b-poly(VEEM) (composition ratio 14
:
1) was 14 × 10−7 cm2 s−1, which is twice as large as that of poly(VEEM)-b-poly(MOEO2VE)-b-poly(VEEM) (composition ratio 2
:
1). Similarly, the D value of poly(VEEM)-b-poly(MOEO3VE)-b-poly(VEEM) (composition ratio 14
:
1) was twice as large as that of poly(VEEM)-b-poly(MOEO3VE)-b-poly(VEEM) (composition ratio 2
:
1). The increment of gas permeability coefficient (P) caused by the decrease of VEEM ratio is originated mainly from the increase of gas diffusivity in the polymer matrix. The crosslinking points in polymer matrix restrict a motion of polymer chains, which lowers the gas diffusion in polymer matrix. For CO2 permeation, poly(VEEM)-b-poly(MOEO3VE)-b-poly(VEEM) showed slightly high diffusivity and solubility compared to the random copolymer, poly(MOEO3VE)-ran-poly(VEEM). Therefore, the increment of flexibility of oxyethylene segment by block copolymerization enhanced not only the gas diffusivity but also the gas solubility in the polymer matrix.
The gas permeability–selectivity tradeoff plots for CO2/N2 gas pair of the present copolymers are shown in Fig. 8. The CO2 permeability increased continuously with the decrease of the composition ratios of VEEM, and the CO2 permselectivity of all the copolymers maintained high revel around 50. The triblock copolymers obviously showed higher permeability than the random copolymers. Notably, the data of poly(VEEM)-b-poly(MOEO3VE)-b-poly(VEEM) (composition ratio 14
:
1) was very close to the 2008 Robeson's upper bound.19
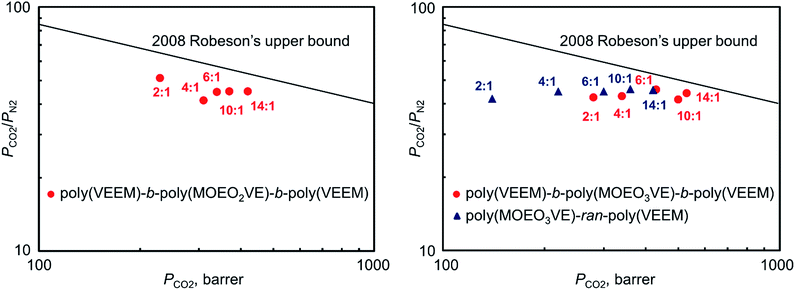 |
| Fig. 8 Permeability–selectivity upper bound plots of poly(VEEM)-b-poly(MOEO2VE)-b-poly(VEEM)s, poly(VEEM)-b-poly(MOEO3VE)-b-poly(VEEM)s, and poly(MOEO3VE)-ran-poly(VEEM)s for CO2/N2 gas pair. | |
Conclusions
Highly CO2-permeable and -permselective triblock copolymers were synthesized by the sequential cationic polymerizations of MOEO2VE/MOEO3VE and VEEM. The non-porous composite membranes with PTFE film were obtained by solution-cast followed by thermal crosslinking. The gas permeability increased with increasing the oxyethylene side chain length and decreasing the VEEM contents because the flexible polymer chains enhanced the gas diffusivity in polymer matrix. Noteworthy, the monomer sequence is dependent on the gas permeability. Triblock copolymers exhibited high gas permeability than the random copolymers even they have the same composition ratios. The triblock copolymers have the crosslinking only at the end segments, which makes the polymer chains more flexible than the random copolymers. These findings would contribute to the membrane field.
Acknowledgements
We are grateful to Maruzen Petrochemical Co. Ltd for supplying MOEO2VE. We are also grateful to Nippon Shokubai Co. Ltd. for supplying VEEM. We are also grateful to Nippon Carbide Industries for supplying 2-hydroxyethyl vinyl ether and 2-(2-hydroxyethoxy)ethyl vinyl ether.
Notes and references
- R. W. Baker and B. T. Low, Macromolecules, 2014, 47, 6999–7013 CrossRef CAS.
- Y. Huang, T. C. Merkel and R. W. Baker, J. Membr. Sci., 2014, 463, 33–40 CrossRef CAS.
- T. C. Merkel, H. Lin, X. Wei and R. W. Baker, J. Membr. Sci., 2010, 359, 126–139 CrossRef CAS.
- H. Lin and B. D. Freeman, J. Mol. Struct., 2005, 739, 57–74 CrossRef CAS.
- H. Lin and B. D. Freeman, J. Membr. Sci., 2004, 239, 105–117 CrossRef CAS.
- H. Lin, T. Kai, B. D. Freeman, S. Kalakkunnath and D. S. Kalika, Macromolecules, 2005, 38, 8381–8393 CrossRef CAS.
- H. Lin and B. D. Freeman, Macromolecules, 2005, 38, 8394–8407 CrossRef CAS.
- S. Kalakkunnath, D. S. Kalika, H. Lin and B. D. Freeman, Macromolecules, 2005, 38, 9679–9687 CrossRef CAS.
- H. Lin and B. D. Freeman, Macromolecules, 2006, 39, 3568–3580 CrossRef CAS.
- H. Lin, E. Van Wagner, J. S. Swinnea, B. D. Freeman, S. J. Pas, J. Hill, S. Kalakkunnath and D. S. Kalika, J. Membr. Sci., 2006, 276, 145–161 CrossRef CAS.
- K. Okamoto, M. Fujii, S. Okamyo, H. Suzuki, K. Tanaka and H. Kita, Macromolecules, 1995, 28, 6950–6956 CrossRef CAS.
- M. Yoshino, K. Ito, H. Kita and K. Okamoto, J. Polym. Sci., Part B: Polym. Phys., 2000, 38, 1707–1715 CrossRef CAS.
- T. Sakaguchi, Y. Koide and T. Hashimoto, J. Appl. Polym. Sci., 2009, 114, 2339–2343 CrossRef CAS.
- T. Sakaguchi, M. Ohashi, K. Shimada and T. Hashimoto, Polymer, 2012, 53, 1659–1664 CrossRef CAS.
- T. Sakaguchi, F. Katsura, A. Iwase and T. Hashimoto, Polymer, 2014, 55, 1459–1466 CrossRef CAS.
- T. Sakaguchi, R. Okunaga, S. Irie, M. Urushisaki and T. Hashimoto, Polym. Bull., 2016 DOI:10.1007/s00289-1820-2.
- T. Sakaguchi, S. Yamazaki and T. Hashimoto, Polymer, 2017, 112, 278–287 CrossRef CAS .
- S. Aoshima, H. Oda and E. Kobayashi, J. Polym. Sci., Part A: Polym. Chem., 1992, 30, 2407–2413 CrossRef CAS.
- L. M. Robeson, J. Membr. Sci., 2008, 320, 390–400 CrossRef CAS.
|
This journal is © The Royal Society of Chemistry 2017 |
Click here to see how this site uses Cookies. View our privacy policy here.