DOI:
10.1039/C7RA02453C
(Paper)
RSC Adv., 2017,
7, 20667-20676
Anaerobic lipopeptide biosurfactant production by an engineered bacterial strain for in situ microbial enhanced oil recovery
Received
27th February 2017
, Accepted 3rd April 2017
First published on 10th April 2017
Abstract
Bacillus mojavensis JF-2 produces water-soluble lipopeptide under aerobic conditions, while Pseudomonas stutzeri DQ1 grows rapidly under anaerobic conditions. These bacteria were used to construct an engineered strain for anaerobic lipopeptide production by protoplast fusion for potential use in microbially enhanced oil recovery (MEOR). The resulting fusant strain FA-2 produced lipopeptide (382 mg l−1) anaerobically at temperatures up to 50 °C, across a pH range of 4.5–10.0, and at salt concentrations as high as 10% NaCl. Experimental results from a physical simulation core at 39 °C suggest that FA-2 has potential for use in MEOR.
1 Introduction
Lipopeptides are extraordinary biosurfactants that are well known for reducing surface tension and interfacial tension between oil and water, thus playing a crucial role in microbial enhanced oil recovery (MEOR).1,2 Lipopeptides also exhibit exceptional emulsifying, foaming, antiviral and anti-mycoplasma properties, and are widely used in many other areas. Biosurfactants have several advantages over chemical surfactants such as: lower toxicity, higher environmental compatibility, biodegradability, higher surface activity, lower critical micelle concentration and synthesis from renewable raw materials.3–5 However, most screened biosurfactant producing bacterial strains are aerobic organisms.6–9 As the underground environment of petroleum reservoirs is anaerobic,10,11 these organisms would not be effective for in situ microbial enhanced oil recovery.12–15 High temperatures, high salinity and low oxygen content in oil reservoirs are known to adversely impact microbial growth and their metabolites production.16–18 Considerable effort has been expended to isolate bacterial strains that can function under reservoir conditions for MEOR, but few strains have been isolated and characterized.
Though very few anaerobic biosurfactant-producing bacteria have been characterized, interest in anaerobic biosurfactant production has steadily increased given the potential use in enhanced oil recovery.12,13,19,20 In MEOR, both the above ground production and the underground production have been undertaken; however, above ground production is costly and complicated.21 The in situ application is more cost-effective, longer lasting, more simply implemented and has a wide range of applications, making it advantageous for MEOR.22 Obtaining microorganisms for anaerobic biosurfactant production that can tolerate oil reservoir conditions such as extremes of pH, temperatures and salinity is prerequisite for in situ surfactant production.15–18 In the absence of suitable native strains, molecular and genetic engineering tools have been used to modify microorganisms to improve their tolerance to harsh reservoir conditions that produce biosurfactants for MEOR.23,24
Protoplast fusion is a common technique for engineering bacteria and has been used to create new strains with desired properties by combining genomes from different organisms. Here, intergeneric protoplast fusion was performed between Bacillus mojavensis JF-2 and Pseudomonas stutzeri DQ-1. B. mojavensis JF-2,25,26 capable of producing lipopeptide with excellent properties, was isolated from oil-field injection brine, and its ability to produce biosurfactant has been characterized.27–31 Several previous works reported that JF-2 produced lipopeptide biosurfactants under anaerobic conditions;26–29,31 however, it has been described that this anaerobic lipopeptide production is limited, unstable, and requires the addition of deoxyribonucleosides or DNA supplements to the culture medium.29 Surfactant-producing capability of JF-2 will be lost when cultured repeatedly which causes problems for field trials and large-scale biosurfactant production.31 The JF-2 strain adopted in this study can only grow and produce lipopeptide aerobically which means the loss of anaerobic biosurfactant-producing ability. P. stutzeri DQ-1 grows rapidly under anaerobic conditions but does not produce biosurfactant. The current investigation was aimed at engineering a new strain with the combined characteristics of rapid anaerobic growth and stable lipopeptide production by protoplast fusion. The resulting strain, FA-2 possessed the desired characteristics and exhibited potential for MEOR under the harsh conditions typical of petroleum reservoirs.(‡)
2 Experimental
2.1 Strains and media
Bacillus mojavensis JF-2 (ATCC 39307), obtained from the American Type Culture Collection (Manassas, VA), and Pseudomonas stutzeri DQ-1, isolated from Daqing Oil Field (PetroChina Company Ltd., Heilongjiang Province, China), were used as parent strains for fusion experiments.
Bacteria were incubated in complete medium consisting of 10 g per liter of tryptone, 5 g of yeast extract, 5 g of beef extract, 5 g of sodium chloride and 10 g of glucose per liter. Lysozyme was dissolved in SMM buffer (0.5 M sucrose, 0.02 M MgCl2, 0.02 M maleic acid, pH 6.8) and kept at −20 °C.
Anaerobic fermentation medium (AFM) was used for anaerobic fermentation of the fusant. AFM was composed of sucrose (32 g per liter), NaCl (5 g per liter), KCl (1 g per liter), KH2PO4 (5.7 g per liter), K2HPO4 (5.25 g per liter), NaNO3 (3 g per liter), MgSO4 (0.25 g per liter), CaCl2 (0.16 g per liter), and yeast extract (2.7 g per liter). Anaerobic culture preparation was performed as modified by Javaheri31 by boiling the medium under a stream of oxygen-free nitrogen.
2.2 Protoplast preparation
The parent strains JF-2 and DQ-1 were incubated in 50 ml complete medium and cultivated at 40 °C and 37 °C respectively. When the optical density (600 nm) reached approximately 1.0, the cells were harvested by centrifugation at 3000 × g at 4 °C for 10 min and then washed twice with sterile normal saline solution (9 g per liter sodium chloride, pH 6.8). The cells were resuspended in 20 ml SMM buffer containing lysozyme (3 mg ml−1 for JF-2 and 6 mg ml−1 for DQ-1) and then shaken at 100 rpm and 37 °C to complete protoplast formation, 50 min enzymolysis for JF-2 and 80 min enzymolysis for DQ-1.32
2.3 Protoplast inactivation
JF-2 protoplast suspensions were transferred into sterile tubes and incubated at 65 °C in a water bath for 160 min to achieve complete inactivation in which the protoplasts cannot get regenerated. DQ-1 protoplast suspensions (3 ml) were transferred to a sterile Petri dish (6 cm diameter) and then placed under a preheated 30 W ultraviolet lamp at a vertical distance of 20 cm for 40 minute irradiation to inactivate DQ-1 completely. The inactivated DQ-1 protoplasts were maintained in the dark for 2 h to avoid photo reactivation repair. Inactivation was verified by lack of growth on regeneration medium.
2.4 Protoplast fusion and regeneration
Inactivated protoplast suspensions of the two parent strains were gently mixed in a 10 ml centrifuge tube and incubated at 30 °C. After 5 min, the tube was centrifuged at 2500 × g for 10 min and then resuspended in 0.5 ml SMM buffer. Fusogenic agent (1.5 ml, SMM buffer containing 40% PEG6000, w/v) was added with mild shaking for homogenization and then incubated at 30 °C for 5 min. The cells were collected by centrifugation (2500 × g, for 10 min) at 4 °C and then added in 2 ml SMM buffer. Protoplasts were diluted with SMM buffer, centrifuged at 2500 × g for 10 min, and diluted again. Serial dilutions of the protoplast suspension (0.1 ml) were applied on the regeneration blood agar and incubated at 37 °C in an anaerobic box (MGC AnaeroPack C-31, Titsubishi) for 4 to 7 days.
2.5 Screening of fusants
The single colonies that caused hemolytic circles were isolated from anaerobic box and then incubated into anaerobic media. Each strain was cultured in triplicate anaerobically at 37 °C for 48 h. The surface tension and the oil displacement activity of the culture broths were measured to demonstrate the ability of anaerobic biosurfactant production. A tension meter (BZY-1, Shanghai HENGPING Co. Ltd) was used to determine the surface tension of the cell-free supernatant. A single fusant strain designated FA-2 with the desired characteristics was isolated through this screening process.
2.6 Evaluation of fusant
B. mojavensis JF-2, P. stutzeri DQ1 and fusant FA-2 were inoculated on complete media respectively and cultured for 50 h at 37 °C, and then their colonial morphologies were compared. Scanning electronic microscope (SEM, FEI Company Quanta™250, USA) was employed to observe microscopic surface features of the bacterial cells.
The effects of temperature, salt concentration, and pH on anaerobic growth of the FA-2 were determined. The surface tension was performed to assess the surface activity of produced biosurfactant, and the biosurfactant activity was measured by the oil-spreading technique.33 The effect of temperature on the growth and surfactant production of FA-2 was examined at 20–60 °C. The anaerobic culture media supplemented with 1–25% NaCl were used to investigate the effect of salt concentration on the growth and surfactant production of FA-2. The pH was varied from 3.5 to 11 to determine the range and optimal pH for growth and surfactant production. The tests for temperature, salt, and pH were performed at 40 °C.
2.7 Optimization of carbon and nitrogen sources
The effect of various carbon sources on the anaerobic growth of FA-2 was assessed by growing the strain in MS medium (pH 7.2) supplemented with 0.5% (w/v) yeast extract, and 2% (w/v) of either glucose, glycerol, molasses, sucrose, sunflower seed oil, coconut oil, olive oil,34 soybean oil, liquid paraffin, or crude oil at 40 °C for 36 hours. Similarly, the effects of various nitrogen sources on growth was determined by growing the organism in the MS medium (pH 7.2) supplemented with 1% (w/v) of either yeast extract, tryptone, soy bean flour or corn steep powder. The MS medium supplemented with 0.5% NaNO3, KNO3, NH4NO3, NH4Cl, or (NH4)2SO4 were used to investigate the effect of inorganic nitrogen sources on anaerobic growth and surfactant production of FA-2. After inoculation, broths were centrifuged at 5000 × g for 10 minutes at 4 °C and the surface tension and the diameter of oil spreading circle of individual supernatants was measured to estimate the biosurfactant production.21
2.8 Growth of FA-2 under anaerobic conditions
Anaerobic cultures were prepared by boiling the medium followed by cooling under a stream of oxygen-free nitrogen. FA-2 cultures (logarithmic phase) were centrifuged to obtain cells and then washed thrice to remove the residual biosurfactant. The cells were resuspended and inoculated into a MD-500 laboratory fermenter (L.E. MARUBISHI, MD-500 bioreactor, Japan). The inoculation dose was 5%, v/v. The bioreactor, equipped with pH, DO, oxidation–reduction potential (ORP), and temperature probes, had a total volume of 10 l and was enclosed and only interconnected with a nitrogen gas bag to guarantee anaerobic conditions. Cultivation was performed at 37 °C using 6 l of media with the agitation speed of 120 rpm. The initial pH was approximately 7.5 and no pH adjustment was performed.
2.9 Biosurfactant extraction and analysis
Overnight seed cultures of FA-2 were inoculated (5%, v/v) into 3000 ml of medium E supplemented with 0.1% NaNO3 and 0.1% yeast extract under strictly anaerobic conditions,35 and then shaken at 100 rpm for 72 h. The culture broth was centrifuged (Himac Centrifuge SCR20BA, HITACHI) at 10
000 × g for 20 min at 4 °C to obtain a cell-free supernatant. The supernatant was treated with 6 mol l−1 HCl to adjust the pH to 2.0 and placed at 4 °C for overnight for acid precipitation. The precipitate was harvested by centrifugation at 10
000 × g for 30 min at 4 °C. The product was leached thrice with a 2
:
1 chloroform–methanol mixture. The organic phase was collected to concentrate biosurfactant using a rotary evaporator (RE-2000, Shanghai YARONG) at 50 °C after which the precipitates were redissolved and transferred to a lyophilizer. The mass of freeze-dried extract was measured to determine the yield of crude biosurfactant.
The biosurfactant was first identified by thin-layer chromatography (TLC), modified from Cooper.36 A sample of biosurfactant was dissolved in methanol and spotted on silica gel plates, which were eluted with chloroform/methanol (10
:
9, v/v). The ninhydrin reagent (composed of 0.3% ninhydrin and 3% glacial acetate acid in butanol) was evenly sprayed to the sheets. The sheets were put into 95 °C oven for drying. The lipopeptide was determined by appearance of a red spot on the plate.
Fourier transform infrared spectroscopy (FT-IR) analysis was employed to identify the molecular structure of the biosurfactant. The sample was dissolved in dichloromethane and then subjected to FT-IR (NICOLET380, Thermo Electron Corporation, USA). FT-IR spectra were collected between 400 and 4000 cm−1 wave numbers.
2.10 Liquid chromatography/tandem mass spectrometry analysis
The crude biosurfactant was further analyzed by liquid chromatography-mass spectrometry (LC-MS) as described previously.37 A TSQ Quantum Access Max Triple Quadrupole mass spectrometer (Thermo Scientific, San Jose, CA) equipped with a reversed-phase HPLC column (Waters Atlantis T3 Column, Waters Corporation, Dublin, Ireland, φ 2.1 × 150 mm) was used. Chromatographic analysis was carried out using a gradient elution program [acetonitrile
:
H2O (1% trifluoroacetic acid), 10%
:
90%, v/v (0–20 min); 60%
:
40%, v/v (20–30 min); 100%
:
0, v/v (30–50 min)] at a flow rate of 0.3 ml min−1. The absorbance was detected at 220 nm. All detection was in positive mode.
Further MS2 measurement on [M + H]+ ion of the metabolite was conducted on the LC-MS machine described above using the same program.
2.11 CMC, emulsification capacity, oil displacement, biosurfactant stability
The surface tension of a series of biosurfactant concentrations was measured for the determination of critical micelle concentration (CMC).38 A stock solution of the crude biosurfactant (1 g l−1) was prepared in distilled water and various dilutions were made to obtain the concentrations from 10 to 150 mg l−1. The CMC was determined from the plateau in the curve of surface tension to biosurfactant concentration.
Emulsification capacity for different hydrophobic substrates was determined by measuring the emulsification index (E24) according to Cooper and Goldenberg.39 For this determination, 4 ml of biosurfactant solution was blended with 6 ml of hydrocarbons, i.e., liquid paraffin, kerosene, crude oil, olive oil, n-heptane and xylene, and vigorously vortexed for 2 min. The mixtures were allowed to stand for 24 h at room temperature. The emulsification index (E24) was calculated using the following equation: E24 (%) = HEL/HS × 100, where, HEL is the height of the emulsion layer, and HS is the height of the total liquid column.
To measure oil displacement activity of the biosurfactant, twenty milliliters of distilled water was added to a Petri dish (90 mm of diameter), followed by the addition of 20 μl of crude oil to the surface of the water. Ten microliters of a culture was added to the center of the oil layer.40,41 The diameters of clear zones were measured for triplicate samples.
Biosurfactant stability at various pH ranging from pH 3–11, salt concentrations from 3–25% and temperatures from 20–121 °C were determined. Biosurfactant was exposed to each of these conditions for 3 h, and then the surface tension and E24 were measured at room temperature.
2.12 Physical simulation core flooding experiment
The effectiveness of fusant FA-2 for MEOR was tested in an artificial core at 39 °C simulating the oil reservoir conditions at Luliang, Xinjiang Oil Field. Each stainless-steel column was packed with an artificial core made of original rock from oil reservoir (inner diameter 3.2 cm, length 29.9 cm, pore volume 60.0 ml) with 373.3 cm d−1 permeability. Two columns were used for the experiment and the columns simulated the heterogeneity of the reservoir rock. The columns were saturated with oil-field injected water of Luliang after vacuum pumping and then flooded with crude oil of Luliang until residual brine was saturated. After aging at 39 °C for 24 h, the columns were flooded again with injected water until the water cut of produced liquid was higher than 98%. Then, 1 pore volume (PV) of anaerobic medium inoculated with 10% bacteria FA-2 was injected into the column (JZ-14 2), while the control column (JZ-14 20) was injected 1 PV of anaerobic medium, and both columns were incubated at 39 °C for 10 d. In the subsequent water flooding, the same injected water was used to flood the column. The flow velocity for the flooding was set at 1 ml min−1. The volume of oil released and the water cut in the produced water were measured. The enhanced oil recovery (EOR) (%) and water cut (%) were calculated as the description by Sun.23
3 Results and discussion
3.1 Screening of fusants
In this study, 356 fusant strains were obtained, and 18 colonies that produced hemolysis circles were isolated preliminarily and incubated in anaerobic medium in anaerobic pipes. The selected fusant strains were named FA-1–FA-18. FA-2 had the most effective biosurfactant-producing capability based on the largest diameter of the oil spreading assay and therefore was chosen for further study (Table 1).
Table 1 The surface tension, diameter of oil spreading circle of culture broths, and cell growth of recombinant strains under anaerobic conditions. All strains were grown in triplicate anaerobically. Triplicate cultures of each strain were analyzed and mean values were shown
Group |
Surface tension (mN m−1) |
Diameter of oil spreading circle (mm) |
OD600 |
CK |
60.9 ± 2.3 |
2 ± 0 |
0 ± 0 |
FA-1 |
49.1 ± 1.6 |
3 ± 1 |
1.7 ± 0.2 |
FA-2 |
26.6 ± 1.1 |
26 ± 3 |
1.6 ± 0.1 |
FA-3 |
33.2 ± 2.2 |
16 ± 5 |
1.2 ± 0.3 |
FA-4 |
50.9 ± 2.5 |
3 ± 1 |
0 ± 0 |
FA-5 |
29 ± 1.3 |
18 ± 2 |
1.2 ± 0.2 |
FA-6 |
31.3 ± 1.2 |
15 ± 4 |
1.4 ± 0.1 |
FA-7 |
39.8 ± 3.3 |
5 ± 1 |
1.3 ± 0.2 |
FA-8 |
41.3 ± 2.1 |
4 ± 1 |
0.3 ± 0.1 |
FA-9 |
39.2 ± 2.7 |
6 ± 2 |
0.4 ± 0.1 |
FA-10 |
41.1 ± 1.9 |
5 ± 1 |
0.6 ± 0.1 |
FA-11 |
36.8 ± 1.2 |
7 ± 2 |
0.9 ± 0.2 |
FA-12 |
29.6 ± 2.8 |
17 ± 3 |
1.1 ± 0.2 |
FA-13 |
30.3 ± 2.1 |
16 ± 6 |
0.9 ± 0.3 |
FA-14 |
27.8 ± 1.3 |
19 ± 1 |
1.3 ± 0.2 |
FA-15 |
39.9 ± 3.8 |
4 ± 2 |
1.1 ± 0.2 |
FA-16 |
45.4 ± 3.3 |
3 ± 0 |
0.7 ± 0.3 |
FA-17 |
38.5 ± 2.6 |
6 ± 2 |
0.8 ± 0.2 |
FA-18 |
30.2 ± 0.3 |
15 ± 3 |
1.2 ± 0.3 |
3.2 Comparison of fusant FA-2 to parent strains
The differences in colony morphology between parent strains and FA-2 were significant. The colony of B. mojavensis JF-2 was volcano-shaped and white in color, while that of FA-2 was flat, yellow-white, dry, and circular. The colonial diameters of parent strain JF-2 were 3–6 mm. In contrast, the colony of FA-2 was larger (12–15 mm). Scanning electron microscopy revealed that FA-2 was morphologically dissimilar to either of the parent strains (Fig. 1).
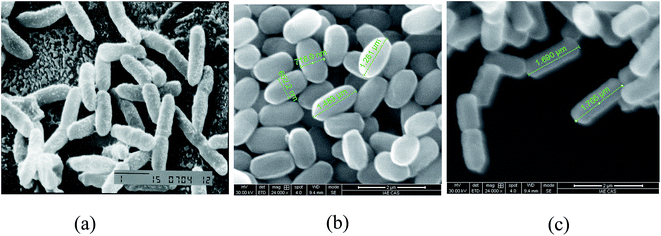 |
| Fig. 1 Scanning electron microscope images of P. stutzeri DQ-1 (a), B. mojavensis JF-2 (b) and fusant strain FA-2 (c). The cells were surveyed under 24 000× scale and the green bars show the length and width of each cell. | |
FA-2 grew well anaerobically exhibiting a maximum optical density of 1.4–1.8 (600 nm) while JF-2 grew poorly reaching a maximum OD of only 0–0.1. The hereditary stability of FA-2's anaerobic lipopeptide production was tested by subculturing the engineered strain. FA-2 exhibited rapid anaerobic growth and surfactant production after 10 successive subcultures, suggesting that FA-2 had high genetic stability. FA-2 had no loss of lipopeptide-production capacity even subcultured sequentially which demonstrated stable genetic characteristics and reliable potential in field trial.
3.3 The effects of temperature, salt concentration, and pH on anaerobic growth of FA-2
The optimal temperature for strain FA-2 to produce biosurfactant was 40 °C, same with the in situ temperature of Luliang. Within 25–50 °C, the surface tension of the culture broth was distinctly lowered, but lower temperature or higher temperature would cause decline in biosurfactant production (Fig. 2). FA-2 functioned well in a wide range of pH (4.5–10.0), but favored neutral and slightly alkaline environment. The diameter of the oil spreading circle and surface tension of culture broth was linearly correlated with the yield of biosurfactant.41 Biosurfactant production of FA-2 was inhibited by high salt concentration, dropping significantly when the NaCl concentration was over 10%.
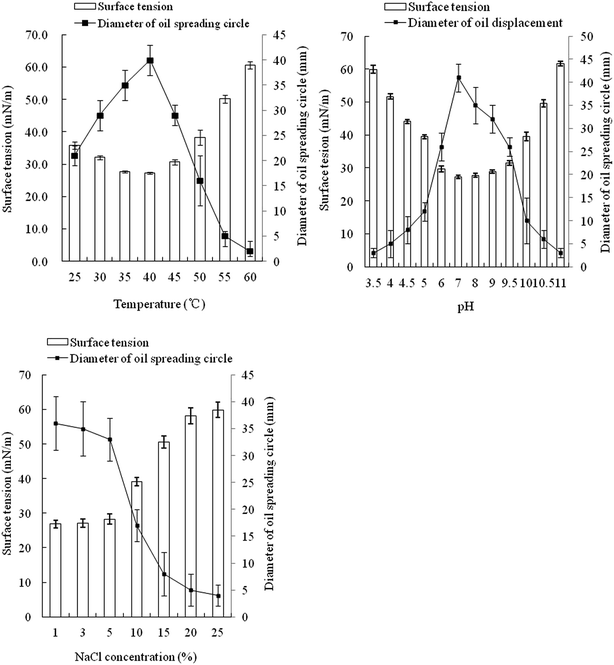 |
| Fig. 2 Effect of temperature, pH, and salinity on surface activity and biosurfactant production of the fusant FA-2 culture. Data points are the mean value of triplicate samples. Error bars show the corresponding standard deviation. | |
3.4 Optimization of carbon and nitrogen sources
Sucrose was the best carbon source tested with respect to surfactant yield efficiency, since it had the most significant reduction in surface tension (from 60.7 to 28.5 mN m−1) and the largest oil spreading circle (37 mm) (Fig. 3). The effect of glucose was slightly inferior to sucrose. Vegetable oil could also be utilized by FA-2 for growth and biosurfactant production. As complex C and N sources, corn steep powder and molasses were not well utilized by FA-2.
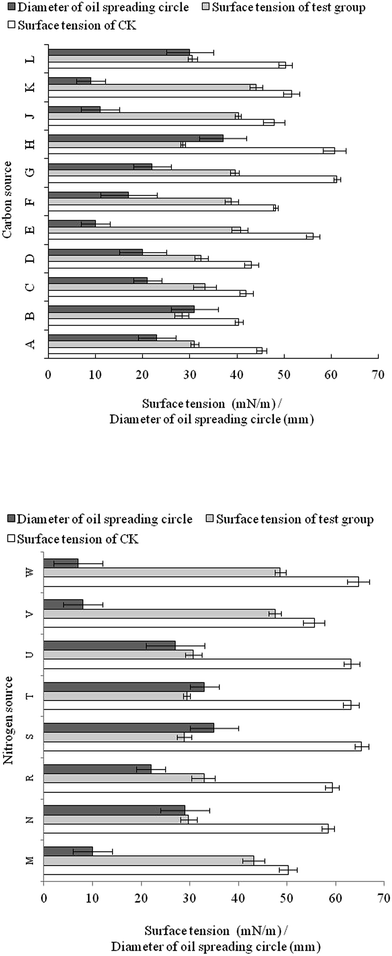 |
| Fig. 3 Effect of various carbon and nitrogen sources on biosurfactant production and surface activity of the fusant strain FA-2. Data points are the mean values of triplicate samples. Carbon sources, (A) sunflower seed oil; (B) coconut oil; (C) olive oil; (D) soybean oil; (E) liquid paraffin; (F) crude oil; (G) glycerol; (H) sucrose; (J) corn steep powder; (K) molasses; (L) glucose. Nitrogen sources, (M) corn steep powder; (N) yeast extract; (R) tryptone; (S) sodium nitrate; (T) ammonium nitrate; (U) potassium nitrate; (V) ammonium sulfate; (W) ammonium chloride. | |
For nitrogen source utilization, all the nitrates contributed to biosurfactant production as the surface tension of culture broth was below 30 mN m−1 and the diameter of oil spreading circle was over 27 mm. Yeast extract was also a suitable nitrogen source for biosurfactant production since the surface tension dropped to 29.7 mN m−1 and the diameter of oil spreading circle was 29 mm (Fig. 3).
3.5 Biosurfactant determination
The output of the crude biosurfactant was 382 mg l−1 which was considerable. Huge amount of bacterial strains was obtained with prominent biosurfactant production in previous studies. Wickerhamomyces anomalus PY189 produced 570 mg l−1 of biosurfactant under optimized conditions reducing the surface tension of the media from 42.5 mN m−1 to 36.5 mN m−1 after 7 days of cultivation.42 Raw glycerol was used as the sole carbon source for biosurfactant production by Bacillus subtilis LSFM-05, and the highest yield (1370 mg l−1) of biosurfactant was get at 60 h of incubation.43 Highest production of biosurfactant, 844, 755 and 2158 mg l−1 for Bacillus subtilis #309, #311 and #573 respectively, were yielded using sucrose as the carbon source.16 Maximal production of 1500 mg l−1 of surfactin was yielded by Bacillus subtilis ATCC 21332 with Mn2+ addition.44 The production of the biosurfactant in our study was lower than that in the studies mentioned above; however, the biosurfactant from FA-2 was obtained from anaerobic culture and was demonstrated with higher surface activity.
A sample spotted on TLC plates and stained with ninhydrin revealed red spots on silica plates indicating the material contained amino acids. The IR spectrum showed bands characteristic of peptide bonds at 3400 cm−1 (stretching mode of NH) and at 1651 cm−1 (CO–N bond stretching mode) (Fig. 4). The bands at 1436 cm−1 and 1258 cm−1 suggested the presence of aliphatic chains (–CH3–, –CH2–) (Fig. 4). These results imply the presence of a peptide-like moiety as well as aliphatic hydrocarbons in the biosurfactant.
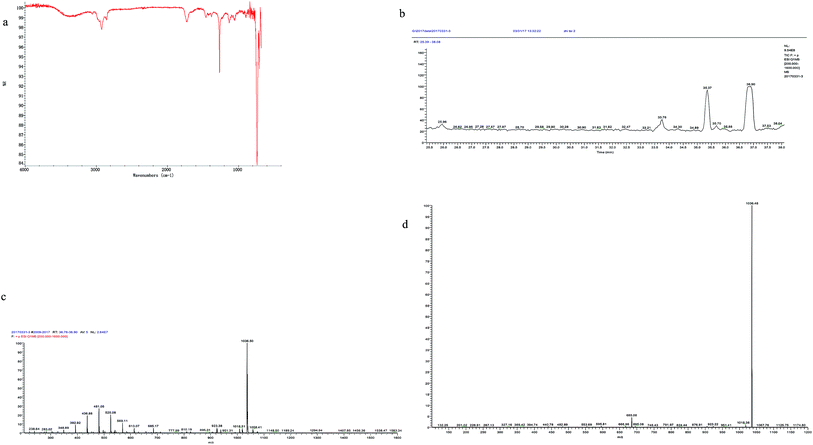 |
| Fig. 4 (a) FTIR spectrum of the crude biosurfactant recovered from cell-free supernatant of FA-2; (b) reversed phase high-performance liquid chromatography of purified biosurfactant; (c) tandem mass spectra of peak 35.37 in (b); (d) tandem mass spectra of peak 36.90 in (b). | |
The crude biosurfactant was analyzed by reverse-phase HPLC (Fig. 4b) and LC-MS (Fig. 4c and d). The results showed that the biosurfactant extract contained at least 2 components. And the main peak showed ions at m/z of 1036.50 ([M + H]+), 1058.41 ([M + Na]+). The MS2 analysis of the mother ion 1036.50 showed a specific ion at m/z 685.08, which is a specific ion for surfactin type biosurfactant produced by Bacillus sp.37,45 We further compared the fragmentation ions from the MS2 spectrum generated from the mother ion 1036.50 with those reported MS2 data of surfactins.37,45 Taken all these together, the main biosurfactant produced by FA-2 was deduced to be C15 surfactin (Ile7 or Leu7).37
3.6 Measurement of CMC, emulsification capacity, biosurfactant stability
The crude surfactant reduced the surface tension of water from 64.7 to 31.2 mN m−1 as the biosurfactant concentration increased to 60 mg l−1 (Fig. 6). The surface tension did not decrease further with increasing biosurfactant concentration and therefore the CMC was estimated at 60 mg l−1; close to that of the biosurfactant obtained by Nitschke and Pastore.46
 |
| Fig. 5 Effect of temperature, pH, and salinity on the stability of the biosurfactant produced by FA-2. Data points are the mean value of triplicate samples. | |
 |
| Fig. 6 Relationship between surface tension and the crude biosurfactant concentration anaerobically produced by FA-2. | |
The results indicated the biosurfactant produced by FA-2 was capable of emulsifying linear, cyclic, long-chain and short chain hydrocarbons as indicated by the maximum emulsification index values obtained with crude oil (Table 2).
Table 2 Emulsification index (E24) of the biosurfactant solution tested against various hydrocarbons. Data are means ± standard deviation of 4 replicates
Hydrocarbon |
E24 (%) |
Liquid paraffin |
53.2 ± 1.3 |
n-Hexadecane |
50.0 ± 0.9 |
Kerosene |
43.6 ± 0.6 |
Olive oil |
53.3 ± 1.1 |
n-Heptane |
45.7 ± 1.3 |
Crude oil |
58.6 ± 1.8 |
Xylene |
49.1 ± 0.5 |
Incubation of cell-free broth at various temperatures did not show any remarkable effect on the surface activity of the biosurfactant solution, therefore the biosurfactant substrate was deemed thermally stable (Fig. 5). However, activity of the biosurfactant solution was affected by pH and salinity. When pH was too acid, the surface tension was high and the E24 values were low, comparable to surfactant-free controls with a surface tension of 66.9 mN m−1 and E24 of 0. The increase of salinity also decreased the surface activity of the biosurfactant.
3.7 Growth of FA-2 in anaerobic fermentor
During growth, OD600 increased slightly for the initial 21 hours and surface tension (ST) decreased from 62.9 to 55.6 mN m−1. After 21 hours, the OD600 began increasing exponentially and eventually entered a stationary phase at 51 h (Fig. 7). Meanwhile ST dropped dramatically and reached a minimum of 30.2 mN m−1 at 54 h. Afterwards, OD600 began to decrease and ST increased, because the death rate surpassed the reproduction rate. Biosurfactant yield was calculated by oil-spreading technique. The highest biosurfactant production rate occurred between 24 and 51 h with a largest diameter of 15 mm. The results of cell growth, surface tension, and biosurfactant yield are consistent. During the fermentation, dissolved oxygen (DO) was maintained at 0 mg l−1 and oxidation–reduction potential remained below 0 mV during the experiment, demonstrating that the fermentation was performed under strictly anaerobic conditions (Fig. 7).
 |
| Fig. 7 Growth of FA-2 and surface tension of the culture broth under anaerobic fermentation at 37 °C, and changes in pH, DO and ORP during the cultivation. | |
3.8 Recovery of crude oil from the physical simulating core
Water flooding resulted in an oil recovery of 1.07% and water cut of 98% (Table 3). A bacterial suspension of FA-2 supplemented with nutrients equivalent to one PV was injected after water flooding to assess the potential enhancement of oil recovery by the surfactant-producing bacteria. The injected engineered bacteria produced lipopeptide by consuming supplemented nutrients in the core which was demonstrated by the surface tension reduction in the flood water. The MEOR value of the core was 5.22%.
Table 3 Results of physical simulation flooding test. The columns were first flooded with injected water until the water cut of produced liquid was higher than 98% to get the enhanced oil recovery (EOR). JZ-14 2 was then flooded by bacterial culture and JZ-14 20 was flooded by water
Test number |
Test core |
EOR of water flooding (%) |
Injection volume (PV) |
Ultimate recovery (%) |
Enhanced recovery (%) |
1 |
JZ-14 2 |
56.82 |
FA-2 (1.0) |
62.04 |
5.22 |
2 |
JZ-14 20 |
53.63 |
CK (1.0) |
54.7 |
1.07 |
Most studies have been launched for lipopeptides production under aerobic moderate conditions, while more importance has been placed on biosurfactant production under extreme conditions.47 The yield of the lipopeptide C9-BS produced by B. subtilis C9 under O2-limited conditions was 3-fold higher than that under O2-sufficient conditions.48 C9-BS exhibited high surface tension reducing capacity, smaller CMC value, and high level of thermal stability. A thermophilic Bacillus strain was isolated from a hydrocarbon containing medium for lipopeptide production at up to 50 °C. Culture broth with produced lipopeptide had low surface and interfacial tension and recovered more than 95% of the residual oil from sandpack columns.9 The lipopeptide showed application potential in oil industries.
The anaerobically produced lipopeptide by FA-2 in this research had substantial surface reducing and emulsification capability for MEOR application. Youssef et al. studied the biosurfactant production by Bacillus in limestone petroleum reservoir and detected average 90 mg l−1 in produced fluids of inoculated wells which is about nine times the minimum concentration required to mobilize entrapped oil from sandstone cores.28 Further studies of Youssef et al.49 in two oil wells found that the increase in oil recovery corresponded directly with biosurfactant production and 52.5 m3 of additional oil occurred in the first 60 days of sampling. The results in our work and previous studies showed the feasibility of stimulating in situ biosurfactant production and increase oil recovery from oil reservoirs.
4 Conclusion
We describe here the first engineered bacterial strain for anaerobic biosurfactant production under extreme environmental conditions by polyethylene glycol-induced protoplast fusion. The engineered strain (FA-2) showed mixed physiological phenotypes of both parents, B. mojavensis JF-2 and P. stutzeri DQ1, such as positive Gram reaction, rod shape, anaerobic biosurfactant production, rapid anaerobic growth, and adaptability to extreme environments. FA-2 produced similar lipopeptide anaerobically with that of B. mojavensis JF-2. The capacity of lipopeptide production remained stable even subcultured continually which demonstrated stable genetic characteristics and reliable potential in field trial. The flexibility and adaptability of FA-2 under extreme conditions was emphasized, since this bacterial strain was aimed at in situ application for MEOR. FA-2 functioned well at temperatures up to 50 °C, pH range of 4.5–10.0, and salt concentrations as high as 10%. The fermentor tests confirmed the mass growth and biosurfactant production of FA-2 under anaerobic conditions. The MEOR value of the core flooding experiment simulating oil reservoir conditions was 5.22%. Therefore, bioaugmentation of FA-2 will be a promising approach for in situ MEOR.
Acknowledgements
The authors are grateful to Prof. Hanping Dong for his assistance in core flooding experiments. This research was financially supported by the National High Technology Research and Development Program of China (No. 2013AA064402) and the National Natural Science Foundation of China (31570121).
References
- I. M. Banat, A. Franzetti, I. Gandolfi, G. Bestetti, M. G. Martinotti, L. Fracchia, T. J. Smyth and R. Marchant, Appl. Microbiol. Biotechnol., 2010, 87, 427–444 CrossRef CAS PubMed.
- R. Sen, Prog. Energy Combust. Sci., 2008, 34, 714–724 CrossRef CAS.
- I. M. Banat, R. S. Makkar and S. S. Cameotra, Appl. Microbiol. Biotechnol., 2000, 53, 495–508 CrossRef CAS PubMed.
- D. A. Vaz, E. J. Gudiña, E. J. Alameda, J. A. Teixeira and L. R. Rodrigues, Colloids Surf., B, 2012, 89, 167–174 CrossRef CAS PubMed.
- Y. Al-Wahaibi, S. Joshi, S. Al-Bahry, A. Elshafie, A. Al-Bemani and B. Shibulal, Colloids Surf., B, 2014, 114, 324–333 CrossRef CAS PubMed.
- A. K. Yadav, S. Manna, K. Pandiyan, A. Singh, M. Kumar, H. Chakdar, P. L. Kashyap and A. K. Srivastava, Microbiology, 2016, 85, 56–62 CAS.
- A. Lawrance, M. Balakrishnan, T. C. Joseph, D. P. Sukumaran, V. N. Valsalan, D. Gopal and K. Ramalingam, Mar. Pollut. Bull., 2016, 82, 76–85 CrossRef PubMed.
- X. Li, A. Li, C. Liu, J. Yang, F. Ma, N. Hou, Y. Xu and N. Ren, Process Biochem., 2012, 47, 626–634 CrossRef CAS.
- I. M. Banat, Biotechnol. Lett., 1993, 15, 591–594 CrossRef CAS.
- P. Gao, H. Tian, Y. Wang, Y. Li, Y. Li, J. Xie, B. Zeng, J. Zhou, G. Li and T. Ma, Sci. Rep., 2016, 6, 20174 CrossRef CAS PubMed.
- A. Vigneron, E. B. Alsop, B. Chambers, B. P. Lomans, I. M. Head and N. Tsesmetzis, Appl. Environ. Microbiol., 2016, 82, 2545–2554 CrossRef CAS PubMed.
- W. Zhu, J. Zhao, H. Han, G. Sun and Z. Song, Energy Fuels, 2015, 29, 7866–7874 CrossRef CAS.
- J. Patel, S. Borgohain, M. Kumar, V. Rangarajan, P. Somasundaran and R. Sen, Renewable Sustainable Energy Rev., 2015, 52, 1539–1558 CrossRef CAS.
- H. Ghojavand, F. Vahabzadeh, M. Mehranian, M. Radmehr, K. A. Shahraki, F. Zolfagharian, M. A. Emadi and E. Roayaei, Appl. Microbiol. Biotechnol., 2008, 80, 1073–1085 CrossRef CAS PubMed.
- E. J. Gudiña, J. F. Pereira, L. R. Rodrigues, J. A. Coutinho and J. A. Teixeira, Int. Biodeterior. Biodegrad., 2012, 68, 56–64 CrossRef.
- J. F. Pereira, E. J. Gudiña, R. Costa, R. Vitorino, J. A. Teixeira, J. A. Coutinho and L. R. Rodrigues, Fuel, 2013, 111, 259–268 CrossRef CAS.
- D. R. Simpson, N. R. Natraj, M. J. McInerney and K. E. Duncan, Appl. Microbiol. Biotechnol., 2011, 91, 1083–1093 CrossRef CAS PubMed.
- S. M. Dastgheib, M. A. Amoozegar, E. Elahi, S. Asad and I. M. Banat, Biotechnol. Lett., 2008, 30, 263–270 CrossRef CAS PubMed.
- P. Gao, G. Li, Y. Li, Y. Li, H. Tian, Y. Wang, J. Zhou and T. Ma, Front. Microbiol., 2016, 7, 186 Search PubMed.
- D. H. Noh, J. B. Ajo-Franklin, T. H. Kwon and B. Muhunthan, J. Geophys. Res.: Biogeosci., 2016, 121, 1158–1177 CrossRef CAS.
- S. De, S. Malik, A. Ghosh, R. Saha and B. Saha, RSC Adv., 2015, 5, 65757–65767 RSC.
- L. J. Chai, F. Zhang, Y. H. She, I. M. Banat and D. J. Hou, Nat., Environ. Pollut. Technol., 2015, 14, 455 CAS.
- S. Sun, Y. Luo, S. Cao, W. Li, Z. Zhang, L. Jiang, H. Dong, L. Yu and W. Wu, Bioresour. Technol., 2013, 144, 44–49 CrossRef CAS PubMed.
- Y. Xu and M. Lu, J. Pet. Sci. Eng., 2011, 78, 233–238 CrossRef CAS.
- M. Folmsbee, K. Duncan, S. O. Han, D. Nagle, E. Jennings and M. McInerney, Syst. Appl. Microbiol., 2006, 29, 645–649 CrossRef CAS PubMed.
- G. E. Jenneman, M. J. McInerney, R. M. Knapp, J. B. Clark, J. M. Feero, D. E. Revus and D. E. Menzie, Dev. Ind. Microbiol., 1983, 24, 485–492 CAS.
- R. T. Armstrong and D. Wildenschild, Int. J. Petrol. Sci. Tech., 2012, 94, 155–164 CrossRef.
- N. Youssef, D. R. Simpson, K. E. Duncan, M. J. McInerney, M. Folmsbee, T. Fincher and R. M. Knapp, Appl. Environ. Microbiol., 2007, 73, 1239–1247 CrossRef CAS PubMed.
- M. J. Folmsbee, M. J. McInerney and D. P. Nagle, Appl. Environ. Microbiol., 2004, 70, 5252–5257 CrossRef CAS PubMed.
- S. C. Lin, K. S. Carswell, M. M. Sharma and G. Georgiou, Appl. Microbiol. Biotechnol., 1994, 41, 281–285 CrossRef CAS.
- M. Javaheri, G. E. Jenneman, M. J. McInerney and R. M. Knapp, Appl. Environ. Microbiol., 1985, 50, 698–700 CAS.
- X. Liang, F. Zhao, R. Shi, Y. Ban, J. Zhou, S. Han and Y. Zhang, Yingyong Shengtai Xuebao, 2015, 26, 2553–2560 CAS.
- N. H. Youssef, K. E. Duncan, D. P. Nagle, K. N. Savage, R. M. Knapp and M. J. McInerney, J. Microbiol. Methods, 2004, 56, 339–347 CrossRef CAS PubMed.
- M. E. Mercade, M. A. Manresa, M. Robert, M. J. Espuny, C. De Andres and J. Guinea, Bioresour. Technol., 1993, 43, 1–6 CrossRef CAS.
- J. B. Clark, D. M. Munnecke and G. E. Jenneman, Dev. Ind. Microbiol., 1981, 22, 695–701 CAS.
- D. G. Cooper, C. R. Macdonald, S. J. Duff and N. Kosaric, Appl. Microbiol. Biotechnol., 1981, 42, 408–412 CAS.
- Z. Ma and J. Hu, Appl. Biochem. Biotechnol., 2015, 177, 1520–1529 CrossRef CAS PubMed.
- A. Ghosh, R. Saha and B. Saha, J. Ind. Eng. Chem., 2014, 20, 345–355 CrossRef CAS.
- D. G. Cooper and B. G. Goldenberg, Appl. Microbiol. Biotechnol., 1987, 53, 224–229 CAS.
- L. R. Rodrigues, J. A. Teixeira, H. C. van der Mei and R. Oliveira, Colloids Surf., B, 2006, 49, 79–86 CrossRef CAS PubMed.
- M. Morikawa, Y. Hirata and T. Imanaka, Biochim. Biophys. Acta, Mol. Cell Biol. Lipids, 2000, 1488, 211–218 CrossRef CAS.
- C. Dejwatthanakomol, J. Anuntagool, M. Morikawa and J. Thaniyavarn, Sci. Asia, 2016, 42, 252–258 Search PubMed.
- A. F. de Faria, D. S. Teodoro-Martinez, G. N. de Oliveira Barbosa, B. G. Vaz, Í. S. Silva, J. S. Garcia, M. R. Tótola, M. N. Eberlin, M. Grossman, O. L. Alves and L. R. Durrant, Process Biochem., 2011, 46, 1951–1957 CrossRef.
- X. Huang, J. N. Liu, Y. Wang, J. Liu and L. Lu, Biotechnol. Biotechnol. Equip., 2015, 29, 381–389 CrossRef CAS PubMed.
- J. S. Tang, F. Zhao, H. Gao, Y. Dai, Z. H. Yao, K. Hong, J. Li, W. C. Ye and X. S. Yao, Mar. Drugs, 2010, 8, 2605–2618 CrossRef CAS PubMed.
- M. Nitschke and G. M. Pastore, Bioresour. Technol., 2006, 97(2), 336–341 CrossRef CAS PubMed.
- S. S. Cameotra and R. S. Makkar, Appl. Microbiol. Biotechnol., 1998, 50, 520–529 CrossRef CAS PubMed.
- H. S. Kim, B. D. Yoon, C. H. Lee, H. H. Suh, H. M. Oh, T. Katsuragi and Y. Tani, J. Ferment. Bioeng., 1997, 84, 41–46 CrossRef.
- N. Youssef, D. R. Simpson, M. J. McInerney and K. E. Duncan, Int. Biodeterior. Biodegrad., 2013, 81, 127–132 CrossRef CAS.
Footnotes |
† Co-first author. |
‡ Engineered FA-2 for in situ MEOR. |
|
This journal is © The Royal Society of Chemistry 2017 |
Click here to see how this site uses Cookies. View our privacy policy here.