DOI:
10.1039/C7RA02538F
(Paper)
RSC Adv., 2017,
7, 22409-22414
Control of ATP concentration in Escherichia coli using an ATP-sensing riboswitch for enhanced S-adenosylmethionine production
Received
1st March 2017
, Accepted 16th April 2017
First published on 24th April 2017
Abstract
ATP is the limiting precursor for S-adenosylmethionine (SAM) synthesis in Escherichia coli. In contrast to traditional optimization of fermentation processes, the riboswitch-based strategy, which was developed as a novel efficient regulation approach, has been applied for the control of the intracellular ATP concentration in order to promote the production of SAM. In this work, a newly discovered ATP-sensing riboswitch ydaO motif was used to balance the requirement of ATP in SAM biosynthesis. The results show that the RNA element ydaO from Bacillus subtilis could play a role in regulating gene expression in E. coli. The ydaO motif-based regulation strategy, which was induced by ATP, improved the ATP level as well as the titer of SAM. The intracellular SAM titer of the best-performing strain, AYV, produced the highest SAM titer (1.23 mg L−1) which was increased by about 55% compared with the control. Overall, the method enabled new insights into the regulation of ATP in E. coli. These results demonstrate a strategy for optimally employing an ATP-sensing riboswitch for the ATP balance of industrial ATP-driven bioprocesses.
Introduction
S-Adenosylmethionine (SAM) is a key active compound in many biological reactions of living organisms and serves as a major donor in many metabolic pathways, such as transmethylation, transsulfuration and aminopropylation.1–4 SAM has shown great clinical potential as a chemotherapeutic agent in various diseases, including arthritis,5 liver disease6,7 and depression.8,9 Accordingly, several attempts have been made to improve SAM production.10 Compared with enzyme catalysis and chemical synthesis, fermentation using yeast to produce SAM is more advantageous for industrial applications.
Generally, SAM is synthesized from L-methionine and ATP under the catalysis of methionine adenosyltransferase in living organisms. L-Methionine as the limiting precursor in the SAM biosynthesis is usually added directly to the medium as a supplement in the fermentation process.11–14 However, intracellular ATP as a precursor and energy carrier is also a main limiting factor for SAM production. As ATP is difficult to manipulate in the same simple way, optimization of the fermentation process is an alternative option to enhance ATP supply.15,16 But beyond that, there is a lack of reliable and efficient methods for ATP regulation.
Increased concentration of ATP would enhance SAM production whereas excessive levels of ATP can inhibit cell growth.17 We have weakened specific metabolic pathways of by-products by a synthetic sRNA-based regulation strategy to control the intracellular ATP level in previous study.18 However, the regulation of this strategy is limited, because it can only up-regulate or down-regulate the intracellular ATP level. The development of riboswitch has offered a novel approach utilizing a ATP-sensing riboswitch to regulate the intracellular ATP level dynamically.19,20 To the best of our knowledge, a riboswitch-based strategy has not previously been used for the regulation of ATP in metabolites biosynthesis.
The ydaO motif is a kind of natural RNA which can specifically bound ATP molecular and regulate expression of endogenous genes in response to ATP concentrations in Bacillus subtilis.19 The ydaO motif as other all known riboswitches acts within feedback loops, modulating expression of specific enzymes in response to on-pathway metabolites. When the intracellular ATP concentration is higher than a certain level, the ydaO motif and the ATP molecules form a stem loop structure of RNA which hinders the transcription of the certain gene downstream of ydaO. On the contrary, when the intracellular ATP concentration is lower, ydaO motif itself can act as a transcriptional promoter element on downstream genes. Therefore, the rational use of ATP sensing riboswitch ydaO motif will be possible to achieve the dynamic control of ATP concentration at a certain level, which is most beneficial to the synthesis of intracellular ATP dependent products (Fig. 1).
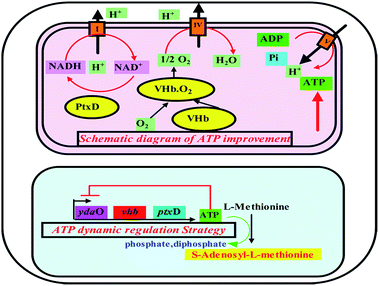 |
| Fig. 1 Schematic diagram of ATP improvement by vhb and ptxD and dynamic regulation strategy using ydaO motif in E. coli ydaO: a kind of riboswitch which can specifically bound ATP molecular and regulate expression of endogenous genes in response to ATP concentration; vhb: encoding Vitreoscilla hemoglobin VHb that could bind oxygen, particularly under low O2 conditions, and deliver it to the respiratory chain by direct interaction with the terminal respiratory cytochrome; ptxD: encoding phosphite dehydrogenase PTDH that could catalyze the nearly irreversible oxidation of phosphite to phosphate, with the concomitant reduction of NAD+ to NADH. | |
Here, we proposed an ATP-sensing riboswitch-based strategy which could be applied to regulate the intracellular ATP concentration in E. coli. The effects of strategy on the SAM production and energy metabolism were investigated.
Materials and methods
Strains, plasmids and growth conditions
The E. coli strains were cultivated in Luria–Bertani (LB) medium (1% tryptone, 0.5% yeast extract, 1% NaCl) supplemented with appropriate antibiotics. Overnight cell culture (1%, v/v) was inoculated into 100 mL M9 mineral medium containing 100 μg mL−1 kanamycin or ampicillin. A defined M9 medium (per liter) contained glucose 20 g, Na2HPO4·2H2O 7.52 g, KH2PO4 3 g, NaCl 0.5 g, NH4Cl 0.5 g, MgSO4·2H2O 0.25 g, CaCl2·2H2O 0.15 g, biotin 1 g, thiamin-HCl 1 g and 10 mL 100× trace elements solution. The 100× trace elements solution (per liter) contained EDTA 5 g, FeCl3·6H2O 0.83 g, ZnCl2 84 mg, CuCl2·2H2O 13 mg, CoCl2·2H2O 10 mg, H3BO3 10 mg, MnCl2·4H2O 1.6 mg. Triple experiments were carried out.
Plasmid construction
E. coli Top 10 was used for plasmid cloning and maintenance. E. coli BL21 (DE3) was used as parent strain for the SAM production. The strains and plasmids used in this study are listed in Table 1. All primers used in this study are listed in Table 2. The schematic overview of the plasmids design and construction are shown in Fig. 2. The ydaO motif was amplified from chromosomal DNA of strain Bacillus subtilis with primers Xba I-ydaO-F and BamH I-ydaO-R. The DNA fragment was digested with Xba I and BamH I, and cloned into the vector pRSFDuet-1. The EGFP fragment was obtained by PCR with primers BamH I-EGFP-F and Xho I-EGFP-R using plasmid pET28a-EGFP as a template. The constructed plasmid pRSFDuet-ydaO and the EGFP fragment were both digested with BamH I and Xho I, and designated as pRSFDuet-ydaO-EGFP.
Table 1 Strains and plasmids in this study
Strains |
Description |
Source |
E. coli TOP10 |
Cloning host |
Biomed |
E. coli BL21 (DE3) |
Expression host |
Biomed |
Control Y |
E. coli BL21 harboring pRSFDuet-ydaO |
This study |
Control G |
E. coli BL21 harboring pRSFDuet-EGFP |
This study |
AYG |
E. coli BL21 harboring pRSFDuet-ydaO-EGFP |
This study |
AYV |
E. coli BL21 harboring pRSFDuet-ydaO-vhb |
This study |
AYP |
E. coli BL21 harboring pRSFDuet-ydaO-ptxD |
This study |
![[thin space (1/6-em)]](https://www.rsc.org/images/entities/char_2009.gif) |
Plasmids |
pET28a-EGFP |
Harboring EGFP |
Lab collection |
pUC57-ptxD |
AmpR, synthetic gene ptxD, optimized in S. cerevisiae codon |
Lab collection |
pUCK-vhb |
AmpR, synthetic gene vhb |
Sangon biotech |
pRSFDuet-ydaO |
Harboring ydaO |
This study |
pRSFDuet-EGFP |
Harboring EGFP |
This study |
pRSFDuet-ydaO-EGFP |
Harboring ydaO and EGFP |
This study |
pRSFDuet-ydaO-vhb |
vhb gene in pRSFDuet-ydaO |
This study |
pRSFDuet-ydaO-ptxD |
ptxD gene in pRSFDuet-ydaO |
This study |
Table 2 Primers used for PCR in this studya
Constructs |
Oligo name |
Oligonucleotides (5′-3) |
The italic characters indicated restriction sites for plasmid construction. |
ydaO |
Xba I-ydaO-F |
TACTCTAGAGATTTTAGCCTCTG |
BamH I-ydaO-R |
GATGGATCCAATCAAAAAACGTTTGA |
EGFP |
BamH I-EGFP-F |
ATTGGATCCATCGCCACCATGGTGAG |
Xho I-EGFP-R |
GTGCTCGAGTGCGGCCGCTTTACTTGTAC |
ptxD |
BamH I-ptxD-F |
CGGGATCCATGCTGCCTAAACTGGTCATCA |
Xho I-ptxD-R |
CCGCTCGAGTTAGCACGCCGCAGGTTCCGCTTT |
Vhb |
BamH I-vhb-F |
CGGGATCCATGTTAGACCAGCAAACCATTAACA |
Xho I-vhb-R |
CCGCTCGAGTTATTCAACCGCTTGAGCGT |
qPCR primers |
qgapdh-F |
CTCCGCTGGCTAAAGT |
qgapdh-R |
GGACGGGATGATGTTCT |
qEGFP-F |
CCAGGAGCGCACCATCT |
qEGFP-R |
TGCCGTTCTTCTGCTTGTC |
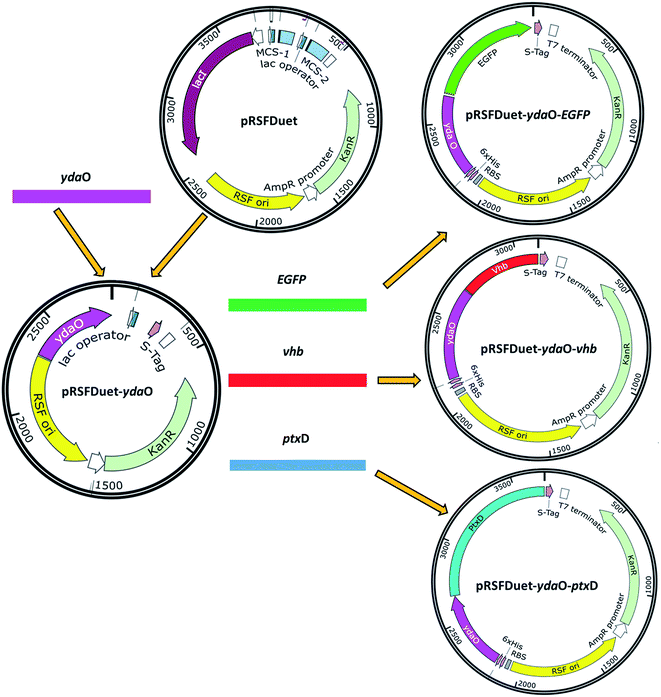 |
| Fig. 2 Schematic overview of the plasmids design and construction. | |
The PCR products of vhb gene and ptxD gene were amplified using synthesized plasmid pUCK-vhb and pUC57-ptxD with primers BamH I-vhb-F/Xho I-vhb-R and BamH I-ptxD-F/Xho I separately. The purified PCR products and vector pRSFDuet-ydaO were digested with BamH I and Xho I. The relative fragments were then cloned into plasmid pRSFDuet-ydaO, designated as plasmids pRSFDuet-ydaO-vhb and pRSFDuet-ydaO-ptxD, respectively.
Analytical procedures
The cell growth was analyzed by measuring the optical density of the fermentation broth at 600 nm using a spectrophotometer (Thermo Scientific, USA). SAM was extracted from the culture broth with 10% (w/v) perchloric acid for 1 h at 30 °C. For ATP and ADP determination, the harvested cells were re-suspended in 0.4 mol L−1 perchloric acid. Subsequent analysis of SAM and ATP/ADP were all carried out by HPLC according to the previous study.18 The fluorescence intensity was measured by flow cytometer (BD, USA).
Isolation of RNA was carried out using total RNA extract kit (Tiangen Biotech, China) and synthesis of cDNA from mRNA was performed using the Quantscript RTFirst Strand cDNA Synthesis Kit (Beijing TransGen Biotech Company, China) according to the manufacturer's instructions. The quantitative real-time PCR (qPCR) was performed on a Rotor-Gene Q system (Analytikjena, Germany) using the Trans Start Top Green qPCR Supermix (Beijing TransGen Biotech Company, China) according to the precious study.21 Each qPCR reaction was performed with three biological replicates. The qPCR primers were listed in Table 2.
Results and discussion
Verification the expression of ydaO motif in E. coli
Flow cytometry and qPCR were performed to determine the fluorescence intensity of the strain AYG which harbors ydaO motif and enhanced green fluorescent protein (EGFP). EGFP without ydaO fragment was used to construct the strain Control G. As shown in Fig. 3A, the cell growth of the strain Control G was a little higher than the strain AYG. However, the fluorescence intensity of AYG was significantly higher than that of the control strain in the fermentation of 24 h (Fig. 3B), which indicated that the ydaO motif could enhance the EGFP transcription in E. coli. On the whole, the fluorescence intensity of control strain maintained a relatively low level at the initial stage of fermentation process, and it increased and stabilized after 12 h fermentation. However, the changes of fluorescence intensity in the strain AYG differed with the control. Firstly, the fluorescence intensity changed with the fermentation time, which means the ydaO motif is indeed affected and regulated endogenous gene expression under changes of the intracellular environment. Secondly, it's clearly that the fluorescence intensity of strain AYG increased at first and then decreased in the initial stage of fermentation. Next, the fluorescence intensity increased continuously at the logarithmic phase, the fluorescence intensity decreased to a stable level until the late of stable phase.
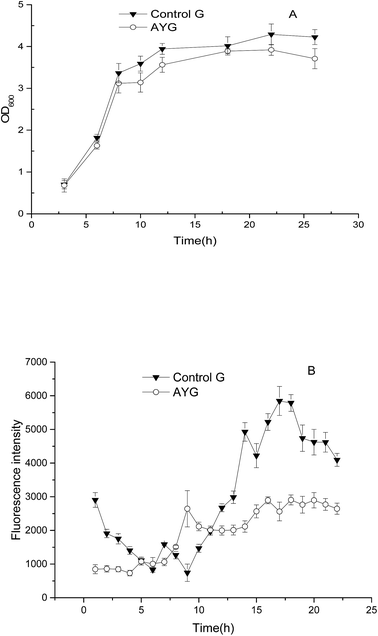 |
| Fig. 3 Comparison of cell growth (A) and fluorescence intensity (B) in the control and the recombinant E. coli under different cultivating time. | |
The mRNA relative expression level was analyzed by qPCR. The mRNA level of the target genes in all other strains was normalized by reference gene gapdh (encoding glyceraldehyde-3-phosphate dehydrogenase) in strain Control G at 12 h, and the normalized mRNA level was assumed to be 1.0. As shown in Fig. 4, the qPCR results clearly indicated that the ydaO motif from Bacillus subtilis could enhance the gene transcription in E. coli.
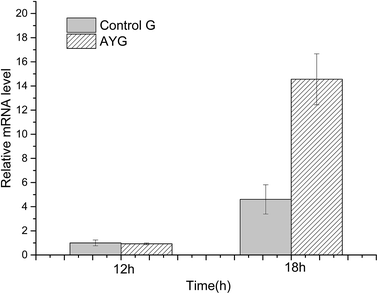 |
| Fig. 4 Comparison of mRNA level in the control and the recombinant E. coli under different cultivating time. | |
Effect of regulation of the ydaO motif on the ATP concentration and fluorescence intensity
The change of fluorescence intensity was due to the effect of ydaO motif. Actually, the change of intracellular ATP concentration contributed to the disturbance of fluorescence intensity according to the mechanism of riboswitch ydaO. The trends of fluorescence intensity were almost opposite with the level of ATP in AYG strain (Fig. 5). That is, when the ATP level was high, the fluorescence intensity was low, and vice versa. It's shown that the intracellular ATP level is lower in the initial stage of fermentation, thus higher fluorescence intensity was observed through driving the transcription of EGFP under regulation of riboswitch ydaO. As the intracellular ATP level reached a higher level gradually during the cell growth, the expression of EGFP was inhibited. The intracellular ATP was consumed to satisfy a large number of metabolic reactions when entering the logarithmic growth phase, thus activating the ydaO motif to drive the expression of EGFP again. The fluorescence intensity decreased slightly from the death phase, probably due to decline caused by the reduction of E. coli. This phenomenon is consistent with the principle that the riboswitch ydaO motif is in response to intracellular concentration of ATP.
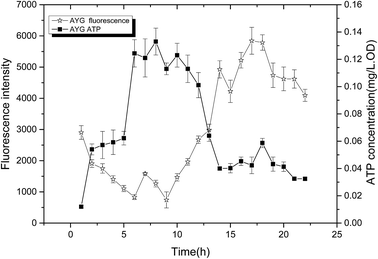 |
| Fig. 5 Comparison of fluorescence intensity and ATP concentration in the strain AYG under different cultivating time. | |
Effect of the ATP sensing riboswitch-based regulation strategy on SAM production
In our previous work, specific metabolic pathways of by-products in the SAM metabolic pathway were weakened by a synthetic sRNA-based regulation strategy. The intracellular ATP level was enhanced and the highest SAM titer could reach to 1.21 mg L−1 in the best performing strain Anti-argB.18 By comparison, the synthetic sRNA-based regulation strategy and the novel method proposed in this study obtained almost the same SAM titer (Fig. 6). However, the regulation of synthetic sRNA-based regulation strategy could only up-regulate or down-regulate the intracellular ATP level. The development of ATP-sensing riboswitch ydaO has offered a novel approach to regulate the intracellular ATP level dynamically.
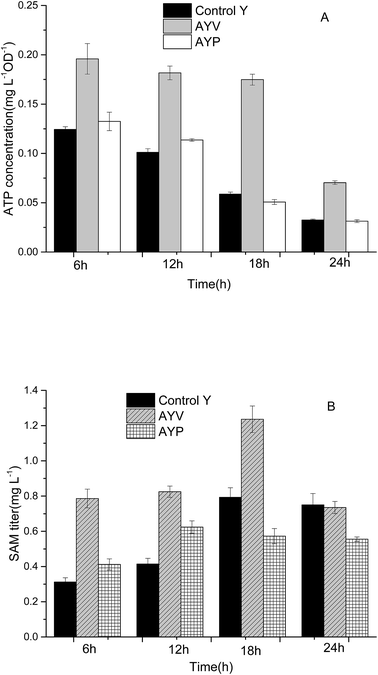 |
| Fig. 6 The effect of ATP dynamic regulation on ATP (A) and SAM (B) titer in flask fermentation of the control and the recombinant E. coli. | |
As the most important electron donor in oxidative phosphorylation, increased NADH level could promote the synthesis of ATP effectively.22,23 The phosphite dehydrogenase (PTDH) from Pseudomonas stutzeri catalyzes the nearly irreversible oxidation of hydrogen phosphonate (phosphite) to phosphate with the concomitant reduction of NAD+ to NADH.24,25 Vitreoscilla hemoglobin (VHb) was first studied in the 1960s. VHb could bind oxygen, particularly under low O2 conditions, and deliver it to the respiratory chain by direct interaction with the terminal respiratory cytochrome.26,27 In this way, it likely enhances aerobic oxidative phosphorylation when O2 is scarce, thus promoting the synthesis of ATP. The PTDH and VHb were always manipulated to increase the intracellular ATP level recently.28–30
As can be seen from Fig. 6, the SAM titer of strain AYP increased at the first 12 h and then no longer increased or even decreased slightly. The change of SAM titer in AYP was consistent with the change of ATP (Fig. 5). The reason may be due to the ptxD gene was optimized in S. cerevisiae codon, which is not suitable for expression in E. coli. In addition, introduction of PTDH changes the ATP level as well as NADH level in vivo. And the SAM tier was not only tightly associated with the ATP level but also affected by NADH level. The change of these cofactors in concentration or form could disturb the intracellular redox balance and energy status, affect the key enzyme in central carbon metabolism and redistribute the metabolites.31
Compared with the strain Control Y and strain AYP, the intracellular ATP level of the strain AYV was higher, and was relatively stable during the whole fermentation cycle. It indicated that the ydaO motif plays a role in intracellular regulation of ATP concentration and maintains the intracellular ATP level at 0.17 mg L−1 OD−1 stably. The intracellular ATP concentration of AYV strain increased by 57%, 80%, 198% and 117%, respectively after fermentation for 6 h, 12 h, 18 h, 24 h comparing with the strain Control Y. In addition, the concentration of intracellular SAM of recombinant strain AYV was also significantly higher than that of the control strain. Especially in the 18 h, the SAM concentration of the AYV strain was increased by about 55%, reaching to 1.23 mg L−1.
Although the ydaO motif is able to regulate the expression of downstream genes in response to the concentration of ATP, it can't regulate intracellular ATP levels to varying degrees. It can only maintain the intracellular ATP concentration on a certain level according to its own response mechanism. And the experimental results showed that the stable ATP level (0.17 mg L−1 OD−1) obtained by ydaO regulation is still too low to meet the requirement of SAM synthesis for ATP in E. coli.
Conclusion
Control of ATP concentration is critical in the industrial ATP-driven bioprocesses.32 We set out to develop such a regulation platform based on the ATP-sensing riboswitch ydaO, which would enable balance of intracellular ATP concentration dynamically. This platform was designed based upon the mechanism that low-level ATP could promote the gene transcription. In developing and testing this platform, we designed a GFP marked recombinant strain in E. coli. The expression of ydaO from Bacillus subtilis in E. coli was confirmed. And it's also shown that the RNA element ydaO could play a role in regulating gene expression in E. coli. The ydaO motif, which was induced by ATP, was further applied to regulate ATP concentration. The strategy improved the ATP level as well as the titer of the SAM, which proved that the ydaO motif had a certain positive effect on the maintenance of intracellular ATP level.
The ydaO motif-based regulation strategy can be a potential tool to balance ATP level for the production of ATP-driven metabolites. However, there is still room for further research aiming at modifying the ydaO motif to broaden its response range to satisfy the SAM production. However, ATP regulation can disturb the whole metabolic network, thus combining with metabolomics analysis for improving the SAM production would be a better way. In addition, the codon optimized gene of ptxD for expression in E. coli or other genes related in the ATP synthesis could be applied in the ydaO motif-based regulation system for a better productivity of ATP-driven products.
Acknowledgements
This work was supported by the National Nature Science Foundation of China (21606073, 21390202, 21436002), the National Basic Research Program of China (973 program) (2013CB733600, 2012CB725200) and the National Key Scientific Instruments and Equipment Development Special Fund (2012YQ0401400302).
References
- P. Giulidori, M. Galli-Kienle, E. Catto and G. Stramentinoli, J. Biol. Chem., 1984, 259, 4205 CAS.
- S. C. Lu, Int. J. Biochem. Cell Biol., 2000, 32, 391–395 CrossRef CAS PubMed.
- M. A. Grillo and S. Colombatto, Amino Acids, 2008, 34, 187–193 CrossRef CAS PubMed.
- S. Gregoire, M. Millecamps, L. Naso, S. D. Carmo, A. C. Cuello, M. Szyf and L. S. Stone, Pain, 2017, 158, 802–810 CrossRef CAS PubMed.
- H. J. H. Blewett, Crit. Rev. Food Sci. Nutr., 2008, 48, 458–463 CrossRef PubMed.
- C. S. Lieber and L. Packer, Am. J. Clin. Nutr., 2002, 76, 1148S–1150S CAS.
- Q. M. Anstee and C. P. Day, J. Hepatol., 2012, 57, 1097–1109 CrossRef CAS PubMed.
- D. Mischoulon and M. Fava, Am. J. Clin. Nutr., 2002, 76, 1158S–1161S CAS.
- G. van der Watt, J. Laugharne and A. Janca, Curr. Opin. Psychiatr., 2008, 21, 37–42 CrossRef PubMed.
- M. Kanai, M. Mizunuma, T. Fujii and H. Iefuji, Appl. Microbiol. Biotechnol., 2017, 101, 1351–1357 CrossRef CAS PubMed.
- S. Shiozaki, S. Shimizu and H. Yamada, Agric. Biol. Chem., 1984, 48, 2293–2300 CAS.
- K. Mincheva, V. Kamburova and V. Balutzov, Biotechnol. Lett., 2002, 24, 1773–1777 CrossRef CAS.
- J. Zhang, X. Wang, E. Su, G. Fang, Y. Ren and D. Wei, Biochem. Eng. J., 2008, 41, 74–78 CrossRef CAS.
- W. Zhao, F. Shi, B. Hang, L. Huang, J. Cai and Z. Xu, Appl. Biochem. Biotechnol., 2016, 178, 1263–1272 CrossRef CAS PubMed.
- H. R. Kant, M. Balamurali and S. Meenakshisundaram, J. Biotechnol., 2014, 188, 112–121 CrossRef PubMed.
- M. H. Li, X. M. Meng, E. J. Diao, F. L. Du and X. Y. Zhao, J. Chem. Technol. Biotechnol., 2012, 87, 1379–1384 CrossRef CAS.
- Y.-A. Na, J.-Y. Lee, W.-J. Bang, H. J. Lee, S.-I. Choi, S.-K. Kwon, K.-H. Jung, J. F. Kim and P. Kim, J. Ind. Microbiol. Biotechnol., 2015, 42, 915–924 CrossRef CAS PubMed.
- Y. Chen, S. Lou, L. Fan, X. Zhang and T. Tan, FEMS Microbiol. Lett., 2015, 362, fnv115 CrossRef PubMed.
- P. Y. Watson and M. J. Fedor, Nat. Chem. Biol., 2012, 8, 963–965 CrossRef CAS PubMed.
- A. Serganov and E. Nudler, Cell, 2013, 152, 17–24 CrossRef CAS PubMed.
- M. Wang, L. Hu, L. Fan and T. Tan, Energy Fuels, 2015, 29, 1823–1829 CrossRef CAS.
- X. Zhu, Z. Tan, H. Xu, J. Chen, J. Tang and X. Zhang, Metab. Eng., 2014, 24, 87–96 CrossRef CAS PubMed.
- J. Y. Lee, C. D. Kang, S. H. Lee, Y. K. Park and K. M. Cho, Biotechnol. Bioeng., 2015, 112, 751–758 CrossRef CAS PubMed.
- J. M. Vrtis, A. K. White, W. W. Metcalf and W. A. van der Donk, J. Am. Chem. Soc., 2001, 123, 2672–2673 CrossRef CAS PubMed.
- R. Woodyer, W. A. van der Donk and H. M. Zhao, Biochemistry, 2003, 42, 11604–11614 CrossRef CAS PubMed.
- B. C. Stark, K. R. Pagilla and K. L. Dikshit, Appl. Microbiol. Biotechnol., 2015, 99, 1627–1636 CrossRef CAS PubMed.
- P. Y. Chi, D. A. Webster and B. C. Stark, Microbiol. Res., 2009, 164, 267–275 CrossRef CAS PubMed.
- S. M. S. Reshamwala, S. K. Pagar, V. S. Velhal, V. M. Maranholakar, V. G. Talangkar and A. M. Lali, J. Biosci. Bioeng., 2014, 118, 628–631 CrossRef CAS PubMed.
- G. Han, X. Hu and X. Wang, Enzyme Microb. Technol., 2015, 78, 27–33 CrossRef CAS PubMed.
- T.-X. Zhao, M. Li, X. Zheng, C.-H. Wang, H.-X. Zhao, C. Zhang and X.-H. Xing, J. Biosci. Bioeng., 2017, 123, 109–115 CrossRef CAS PubMed.
- X. L. Chen, S. B. Li and L. M. Liu, Trends Biotechnol., 2014, 32, 337–343 CrossRef CAS PubMed.
- K. Y. Hara and A. Kondo, Microb. Cell Fact., 2015, 14, 9 CrossRef PubMed.
|
This journal is © The Royal Society of Chemistry 2017 |
Click here to see how this site uses Cookies. View our privacy policy here.