DOI:
10.1039/C7RA02689G
(Paper)
RSC Adv., 2017,
7, 23313-23318
Disposal of hexabromocyclododecane (HBCD) by grinding assisted with sodium persulfate
Received
5th March 2017
, Accepted 24th April 2017
First published on 27th April 2017
Abstract
Hexabromocyclododecane (HBCD) has been widely used as a flame retardant in polystyrene and textiles, and is ubiquitous in all kinds of environmental media. The sound disposal of HBCD wastes should be made a priority because of their toxicity, bioaccumulation and persistence. Mechanochemical methods for waste disposal have proven to be effective, environmentally friendly and simple in their execution; thus, in this work, one such method was employed to destroy HBCD. Sodium persulfate (PS) was used as a co-milling reagent and the milling balls were made of zirconia. During the experiment, HBCD, PS, and sodium hydroxide (NaOH) were put into a planetary ball mill, wherein the reactions took place. The grounded samples were analyzed via ion chromatography (IC) and liquid chromatography-mass spectrometry (LC-MS). Fourier transform infrared spectroscopy (FTIR), Raman spectroscopy, X-ray powder diffraction (XRD), and thermogravimetry (TG) analyses were employed as assistant measures. Results showed that the degradation and bromine recovery efficiency of HBCD were 95% and 100%, respectively, after 2 h milling. Furthermore, FTIR revealed the breakage of “–C–Br–” and “–C–H–” bonds, and the Raman spectra indicated the generation of amorphous and graphitic carbon. In short, PS–NaOH proved to be an effective co-milling reagent for the treatment of HBCD.
1. Introduction
HBCD has been widely used in expanded and extruded polystyrene, textiles, electronic appliances, and other equipment as an additive flame retardant.1 Owing to its characteristics of persistence and bioaccumulation, it has been detected in various environmental media, such as air containing air-conditioning filter dust, surface soil near industrialized regions, and river water.2–4 Studies on fish and marine invertebrates have revealed that HBCD has been detected in these species.5 In addition, HBCD has been found in human milk and serum worldwide.1 Toxicity testing of HBCD has detected cardiovascular, developmental, and reproductive effects on animals.6–8 Therefore, an effective disposal of HBCD wastes must be made a priority.
Incineration is an effective method for the treatment of HBCD, with a destruction efficiency of above 99%, but toxic byproducts, such as polybrominated dibenzodioxins/dibenzofurans (PBDDs/Fs) are generated when the input of HBCD is high.9 Zero-valent iron nanoparticle aggregates, polysulfide and bisulfide, and photodegradation have been utilized to destroy HBCD; however, these methods either required complicated reaction conditions, or were incomplete in terms of the debromination and destruction of the HBCD.10–12
Attention has turned to mechanochemical methods in recent years because they are simple in their execution effective, and environmentally friendly. Co-milling reagents play a significant role in the destruction of pollutants, and the most commonly used co-milling reagents are metal oxides, metals a mixture of metal and metal oxides. PS can be activated by heat/UV, metal (usually Fe2+) or alkali to generate sulfate radicals (SO4˙−) and hydroxyl radicals (˙OH), which can be used to degrade various kinds of pollutants because of their strong oxidizability.13–17 However, since researches have usually focused on the treatment of contaminants in aqueous phase, for the disposal of solid wastes, conventional technologies do not seem to be the best choice. Therefore, a combination of PS and a mechanochemical technique is required. When used as a co-milling reagent, PS may be activated by the process of ball milling, during which mechanical forces, such as impact, stress, fraction, and deformation, can create transient high temperature.18,19 The subsequently generation radicals, such as SO4˙− and ˙OH, should be effective in the destruction of pollutants, at least in theory.
Liu et al. used Fe0 activated PS as a co-milling reagent to dispose of sulfamethoxazole (SMX) and trimethoprim (TMP) and attributed the degradation of SMX and TMP to the generation of SO4˙− and ˙OH during the milling process.20 In addition, the mixture of PS and CaO was effective in the treatment of TBBPA.21 Huang et al. reported the destruction of decabromodiphenyl ether (DBDE) by using mechanochemically activated PS and found that the DBDE converted into CO2 and Br2, with mineralization finally realized.22
Our previous study revealed that NaOH activated PS as a co-milling reagent is effective for the treatment of a chlorinated polyfluorinated ether sulfonate (F-53B).23 Thus, in terms of HBCD, NaOH activated PS might be feasible as well because the bond energy of “–C–Br–” bond is weaker than “–C–F–” bond. Therefore, in the present study, PS was employed as a co-milling reagent to destroy HBCD and NaOH was used as an activator. The degradation efficiency was evaluated by changing the key parameters in the experiment. Fourier transform infrared spectroscopy (FTIR) and TG analyses were carried out in order to verify the variations of chemical bonds and functional groups. Finally, the degradation mechanism and possible reaction pathways are proposed.
2. Materials and methods
2.1 Materials
HBCD (>95%) and methanol were purchased from J&K Scientific (Beijing, China). PS, NaOH, sodium sulfate (Na2SO4), sodium bromide (NaBr), and potassium bromide (KBr) were obtained from Sinopharm (Beijing, China). All solutions were prepared with Milli-Q deionized water (DI; 18.2 MΩ). All organic solvents and chemical reagents were at least analytical grade and used as received, without further purification.
2.2 Experimental procedure
A planetary ball mill (QM-3SP2, Nanjing University Instrument Corporation, China) equipped with four zirconia milling pots (100 mL) was employed in the experiment. Co-milling reagents (PS, NaOH, and PS–NaOH) were mixed with HBCD and then put into the pots along with 60 g zirconia balls, having a diameter of 9.6 mm. The rotation speed was set at 275 rpm and the operation cycle was 30 on, 5 min off to prevent overheating. The milled samples were collected and then preserved in a dry and hermetic apparatus for further analysis.
2.3 Sample analysis
For HBCD analysis, 0.10 g of each milled sample was dissolved in 5 mL methanol with 20 min vibration time at 25 °C. After that, the extract was centrifuged at 3000 rpm for 10 min and the solution was filtered by a 0.22 μm nylon filter. The separated solid residue was extracted once more in the same manner and a mixture of the solution was subjected to instrumental analysis. HBCD was measured via LC-MS. Separation was done by an Ultimate 3000 HPLC system (Dionex, Germering, Germany) equipped with a Zorbax Eclipse Plus C18 column (150 mm × 4.6 mm, 5 μm, Agilent, Santa Clara, CA, USA). The target compound was detected by an API 3200 triple quadruple mass spectrometer (Applied Biosystems, Foster City, USA) in the negative mode of ionization. A 10 μL volume of the sample solution was injected by the automatic sampling system. The flow rate of the mobile phase was 1 mL min−1 and the column unit was held at 30 °C. Methanol/H2O (90/10, v/v) was used as the mobile phase. Q1 and Q3 were set at 640.6 and 79.0 respectively. The ionization was set at an ion spray voltage of −4.5 kV and a temperature of 450 °C, using nitrogen for drying. The declustering potential, entrance voltage, and collision pressure were set at −45, −6 and −35 V respectively and the ion source gas 1 and ion source gas 2 were set at 55 psi.
For ion analysis, 0.10 g of each sample was extracted using ultrapure water in the same manner as described above. The concentration of bromine ions (Br−) was analyzed with an ion chromatography system (ICS-1100, Dionex, USA) equipped with a guard column and a separation column (Dionex IonPac AS19 Analytical, 4 mm × 250 mm, USA). The injection volume was 20 μL and the column temperature was held at 35 °C. The mobile phase was 20 mM KOH and the flow rate was 1 mL min−1. The debromination efficiency can be expressed as (measured soluble bromine ion)/(calculated bromine from the initial HBCD).
2.4 Characterization of the milled sample
The milled mixture collected at specific times was characterized by using various approaches. XRD, used to identify the crystalline products, was conducted via an X-ray diffractometer (X' Pert PRO MPD, PANalytical, Holland) with Cu Kα radiation at a speed of 6° min−1 with 2θ = 20–80°. FTIR spectra were obtained using a Nicolet 6700 (NEXUS 670, East branch instrument, USA) via the KBr disk method from 250 to 4250 cm−1. A Raman spectrometer (Lab RAM ARAMIS, HORIBA Jobin Yvon, France) was used with a 532 nm Ar-laser beam and scan range from 400 cm−1 to 2200 cm−1. TG analysis of the milled samples was carried out on a Rigaku Thermo plus TG 8120 thermobalance. Less than 10 mg of sample was placed in an open alumina pan and heated up to 800 °C at a constant heating rate of 10 °C min−1. Analysis was conducted in a flow of nitrogen at a constant rate of 50 mL min−1.
3. Results and discussion
3.1 Effect of different co-milling reagents
PS–NaOH was selected as a co-milling reagent because of its good performance in the destruction of F-53B. PS (4.29 g), NaOH (1.18 g) and PS–NaOH (PS 4.29 g, NaOH 1.18 g) were co-ground with HBCD (0.5 g) for 2 h, and the residual HBCD and bromine ions were detected (as shown in Fig. 1).
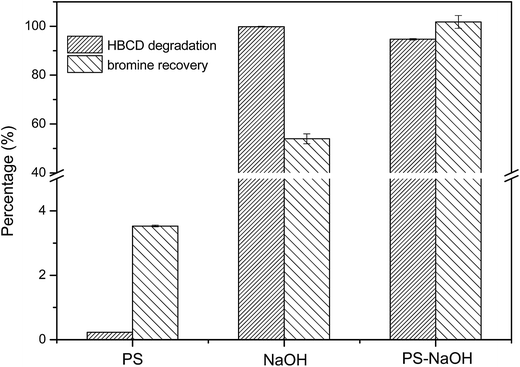 |
| Fig. 1 Effect of different co-milling reagents on the destruction of HBCD (HBCD 0.50 g, 2 h milling). | |
As can be seen, the degradation and debromination efficiency of HBCD were extremely low when using PS as the co-milling reagent. NaOH was quite effective in the degradation of HBCD, as there was almost no HBCD left after 2 h milling. However, the performance was not so good in terms of debromination (the bromine recovery of HBCD was only 54% after 2 h treatment), thus, the HBCD probably existed in the form of bromine intermediates. When PS–NaOH was used as the co-milling reagent, the degradation efficiency of HBCD was 95% and the bromine recovery was nearly 100%. It should be noted that, normally, the degradation efficiency should be higher than the debromination efficiency; this abnormal phenomenon observed could be attributed to the impurities in HBCD. Therefore, we chose PS–NaOH as the co-milling reagent to conduct our experiments.
3.2 Effect of different PS to NaOH mass ratios
Our previous study23 revealed that the mass ratio of PS to NaOH played a significant role in the destruction of F-53B, so, the degradation efficiency of HBCD was evaluated by changing the mass ratio of PS to NaOH in the present study. During the experiment, the mass of PS was kept constant at 4.29 g whereas the mass of NaOH varied from 0.62 g to 1.44 g, and the results are shown in Fig. 2. As can be seen, the degradation and debromination efficiencies of HBCD were low when the mass of NaOH was 0.66 g. When the mass of NaOH increased to 0.92 g, there was an obvious promotion in both the degradation efficiency and bromine recovery. When we continued to increase the mass of NaOH, the degradation and debromination efficiencies of HBCD remained high. This is probably because an increase of NaOH promotes for the activation of PS. From the perspectives of effectiveness and economy, the relatively proper mass ratio of PS to NaOH was 4.29
:
1.18.
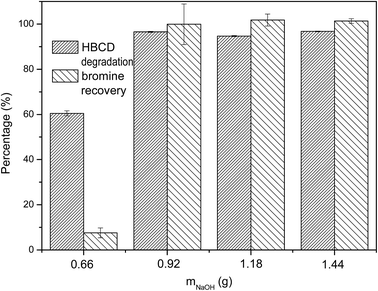 |
| Fig. 2 Effect of the mass ratio of PS to NaOH on the destruction of HBCD (PS 4.29 g, HBCD 0.50 g, changing NaOH, 2 h milling). | |
Further studies were conducted to explore the reaction mechanism. During the experiment, NaOH was kept constant at 1.18 g whereas the mass of PS varied from 2.14 g to 6.42 g, and the results are shown in Fig. 3. As can be seen, when we changed the mass of PS, the degradation efficiency of HBCD remained high. However, the bromine recovery of HBCD increased firstly and then decreased, and a peak was obtained when the mass of PS was 4.29 g. The high degradation efficiency is not difficult to explain because we have already shown that NaOH is effective for the degradation of HBCD. Our previous study revealed that there were SO4˙− and ˙OH were generated in a mechanochemical system that used Fe0-activated PS as a co-milling reagent.20 Therefore, we infer that SO4˙− and ˙OH might exist in the NaOH–PS system, and the fluctuation of bromine recovery can be interpreted as follows. When the mass of PS was low, NaOH played a major role in the degradation and debromination of HBCD, so, the results were close to the situation in which NaOH was used alone. When we increased the mass of PS, the active materials increased correspondingly in theory, which promoted the debromination of HBCD, thus, the high bromine recovery was achieved. However, when we continued to increase the mass of PS, the high concentration of the radicals in the system may have accelerated radical scavenging reactions,24 causing PS wasting, so, debromination efficiency began to decrease. Obviously, the relatively optimal mass of PS was around 4.29 g.
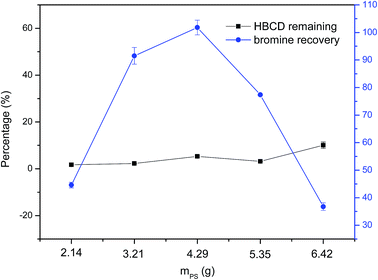 |
| Fig. 3 Effect of the mass ratio of PS to NaOH on the destruction of HBCD (NaOH 1.18 g, HBCD 0.50 g, changing PS, 2 h milling). | |
3.3 Effect of mass ratio of co-milling reagents on HBCD
The mass ratio of co-milling reagents to target pollutants (the mass of the co-milling reagents/the mass of the target pollutants) is one of the most important parameters in mechanochemical destruction. Further research was conducted to optimize the mass ratio of co-milling reagents to HBCD. During the experiment, the mass ratio of PS to NaOH was kept constant and the mass ratio of co-milling reagents to HBCD varied from 2.74 to 10.94; the results are shown in Fig. 4. As can be seen, the degradation and debromination efficiencies increased with the increase of the mass ratio. Both the degradation and debromination of HBCD were over 95% when the mass ratio was 10.94, therefore, we chose 10.94 as the relatively optimal mass ratio. Additionally, when the mass ratio was low, the difference between bromine recovery and HBCD degradation was significant, so, we infer that many brominated intermediates existed in the milled samples.
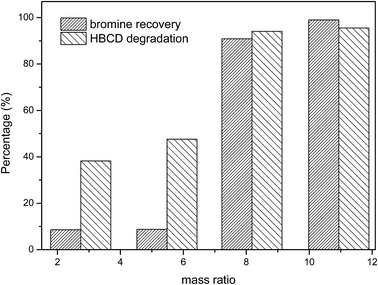 |
| Fig. 4 Effect of the mass ratio of co-milling reagents to HBCD on the destruction of HBCD (PS : NaOH = 4.29 : 1.18, HBCD 0.50 g, 2 h milling). | |
3.4 Effect of milling time
Generally, destruction efficiency increased with an increase of milling time. However, an increase of the milling time means an increase of energy. Therefore, we should weigh the destruction efficiency and the milling time when conducting mechanochemical destruction experiments.
The best PS to NaOH mass ratio (PS
:
NaOH = 4.19
:
1.18) and the relatively optimal mass ratio of co-milling reagents to target pollutants (10.94) were adopted to evaluate the effect of milling time on the destruction efficiency of HBCD, and the results of which are shown in Fig. 5. As can be seen, both the degradation efficiency and bromine recovery increased with the increase of milling time. During the first hour, 87% bromine recovery and 90% HBCD degradation occurred (in the case of Fe–SiO2, both the degradation and debromination efficiencies were less than 70% after 1 h milling25), and the degradation and debromination efficiencies were 95% and 100%, respectively, after 2 h milling. Therefore, 2 h milling was appropriate for the effective destruction of HBCD.
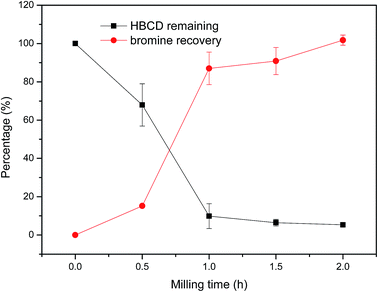 |
| Fig. 5 Effect of the milling time (PS 4.29 g, NaOH 1.18 g, HBCD 0.50 g). | |
A considerable amount of SO42− was detected in the extracted solutions of the milled samples, and the agglomeration of the solid samples was high after 2 h milling. Thus, the trend of destruction (increased rapidly at first and then slowed down) may be attributed to the consumption of the active materials (NaOH and radicals) and the agglomeration of the samples. During the first hour, the degradation efficiency of HBCD was slightly higher than the debromination efficiency; however, in the second hour, the difference between the degradation and debromination efficiencies was negligible. This phenomenon may be due to the generation of brominated intermediates during the first hour and the rapid destruction of them in the second hour.
TG analysis was applied to further confirm the destruction of HBCD, and the TG patterns of the milled samples are shown in Fig. 6. TG analysis indicated that the weight loss temperature of HBCD was between 233 to 344 °C (data not shown), therefore, the mass changes in this temperature range can be used to describe the destruction of HBCD. As can be seen, the weight loss of the 10 min milled sample was higher than that of the 2 h milled sample in the temperature region of 233 to 344 °C (although the residual PS in the milled sample had weight loss in this temperature range of, the weight loss of the 10 min sample was apparently more than the weight of the pure PS). After 2 h milling, the weight loss was almost negligible.
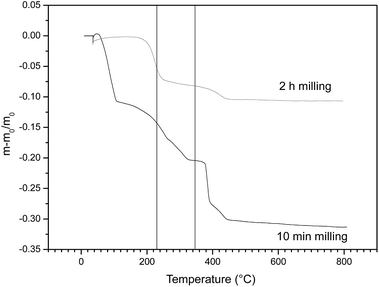 |
| Fig. 6 TG patterns of the milled samples (PS 4.29 g, NaOH 1.18 g, HBCD 0.50 g). | |
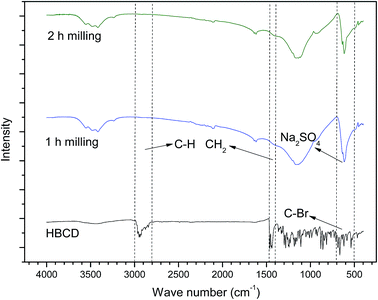 |
| Fig. 7 FTIR patterns of the milled samples (PS 4.29 g, NaOH 1.18 g, HBCD 0.50 g). | |
These results indicate that HBCD might have been destroyed into inorganic form.
3.5 Mechanism of the reaction
To confirm the products and to explore the mechanism of the reaction, FTIR, XRD and Raman analysis were conducted. The FTIR patterns of the milled samples are shown in Fig. 7. As can be seen, the peaks at around 2800–3000 cm−1, 1400–1470 cm−1, and 500–700 cm−1 come from the vibration of ν(C–H), δ(CH2), and ν(C–Br),25 respectively, which can be found in the spectra of HBCD. However, all of these peaks disappeared in the spectra of the 1 h milled and 2 h milled samples and a new peak at around 671 cm−1 appeared, which was assigned to Na2SO4. Peaks of Na2SO4 were also observed in the patterns of XRD (Fig. 8). As shown in Fig. 9, in the Raman spectra of the 0 h mixture and 0.5 h milled samples, the peak bands around 1350 cm−1 and 1585 cm−1, which are usually identified as amorphous and graphite carbons respectively,26 were not seen. Whereas, two peaks around 1350 cm−1 and 1585 cm−1 appeared after 2 h milling, which indicated that HBCD was destroyed and carbonization was realized. These results imply the breakage of “–C–H–”, “–C–Br–” and “–C–C–” bonds and the generation of amorphous and graphite carbons and Na2SO4.
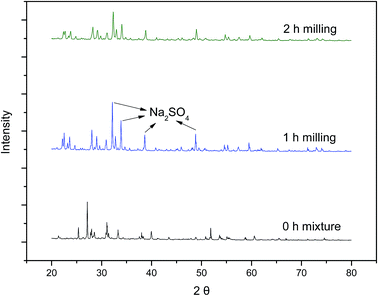 |
| Fig. 8 XRD patterns of the milled samples (PS 4.29 g, NaOH 1.18 g, HBCD 0.50 g). | |
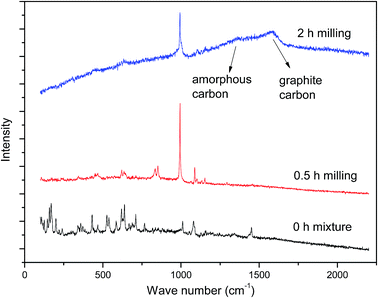 |
| Fig. 9 Raman patterns of the milled samples (PS 4.29 g, NaOH 1.18 g, HBCD 0.50 g). | |
According to instrumental analysis and characterization of the milled samples, the mechanism of the reaction is put forward as follows: ball milling provided the reaction with energy, and NaOH took part in the reaction and destroyed HBCD. Due to the activation of NaOH, PS was decomposed and SO4˙− or ˙OH were released. Then, a series of reactions occurred between HBCD and the active materials (NaOH and radicals); “–C–H–”, “–C–C–” and “–C–Br–” bonds were broken; and brominated intermediates were generated; subsequently, these intermediates continued to react with the active materials until HBCD was completely destroyed. Carbonization was finally realized, as PS was transformed into Na2SO4 and the organic bromine was converted into the ionic state.
4. Conclusions
HBCD was milled with PS–NaOH and 95% HBCD degradation and 100% bromine recovery can be obtained at the relatively optimal mass ratio. The destruction efficiency of HBCD increased with the increase of milling time and mass ratio. FTIR indicated the breakage of “–C–H–” and “–C–Br–” bonds, and Raman analysis demonstrated the carbonization of HBCD. Debromination and carbonization are proposed as the main pathways of HBCD destruction.
Acknowledgements
This study was supported by the Beijing Natural Science Foundation (Project No. 16L00073), the National Natural Science Foundation (Project No. 21547001) and the Ministry of Science and Technology (Project No. 2013AA06A305).
References
- C. C. Carignan, M. A.-E. Abdallah, N. Wu, W. Heiger-Bernays, M. D. McClean, S. Harrad and T. F. Webster, Environ. Sci. Technol., 2012, 46, 12146–12153 CrossRef CAS PubMed.
- H.-G. Ni and H. Zeng, Sci. Total Environ., 2013, 458, 15–19 CrossRef PubMed.
- J. Tang, J. Feng, X. Lia and G. Li, Environ. Sci.: Processes Impacts, 2014, 16, 1015–1021 CAS.
- M. Ichihara, A. Yamamoto, K.-i. Takakura, N. Kakutani and M. Sudo, Chemosphere, 2014, 110, 78–84 CrossRef CAS PubMed.
- M.-H. Son, J. Kim, E.-S. Shin, S.-H. Seo and Y.-S. Chang, J. Hazard. Mater., 2015, 300, 114–120 CrossRef CAS PubMed.
- C. H. Marvin, G. T. Tomy, J. M. Armitage, J. A. Arnot, L. McCarty, A. Covaci and V. Palace, Environ. Sci. Technol., 2011, 45, 8613–8623 CrossRef CAS PubMed.
- H. Hong, R. Shen, W. Liu, D. Li, L. Huang and D. Shi, Mar. Pollut. Bull., 2015, 101, 110–118 CrossRef CAS PubMed.
- M. Wu, Z. Zuo, B. Li, L. Huang, M. Chen and C. Wang, Ecotoxicology, 2013, 22, 1200–1207 CrossRef CAS PubMed.
- F. E. Mark, J. Vehlow, H. Dresch, B. Dima, W. Gruettner and J. Horn, Waste Manage. Res., 2015, 33, 165–174 CrossRef CAS PubMed.
- C.-p. Tso and Y.-h. Shih, J. Hazard. Mater., 2014, 277, 76–83 CrossRef CAS PubMed.
- K. W. Lo, S. C. Saha-Roy and U. Jans, Chemosphere, 2012, 87, 158–162 CrossRef CAS PubMed.
- D. Zhou, Y. Wu, X. Feng, Y. Chen, Z. Wang, T. Tao and D. Wei, Environ. Sci. Pollut. Res., 2014, 21, 6228–6233 CrossRef CAS PubMed.
- C. Qi, X. Liu, C. Lin, X. Zhang, J. Ma, H. Tan and W. Ye, Chem. Eng. J., 2014, 249, 6–14 CrossRef CAS.
- J. Yan, M. Lei, L. Zhu, M. N. Anjum, J. Zou and H. Tang, J. Hazard. Mater., 2011, 186, 1398–1404 CrossRef CAS PubMed.
- O. S. Furman, A. L. Teel and R. J. Watts, Environ. Sci. Technol., 2010, 44, 6423–6428 CrossRef CAS PubMed.
- C. Qi, X. Liu, W. Zhao, C. Lin, J. Ma, W. Shi, Q. Sun and H. Xiao, Environ. Sci. Pollut. Res., 2015, 22, 4670–4679 CrossRef CAS PubMed.
- C. D. Qi, X. T. Liu, J. Ma, C. Y. Lin, X. W. Li and H. J. Zhang, Chemosphere, 2016, 151, 280–288 CrossRef CAS PubMed.
- K. Zhang, J. Huang, H. Wang, G. Yu, B. Wang, S. Deng, J. Kano and Q. Zhang, RSC Adv., 2014, 4, 14719–14724 RSC.
- K. Zhang, J. Huang, G. Yu, Q. Zhang, S. Deng and B. Wang, Environ. Sci. Technol., 2013, 47, 6471–6477 CAS.
- X. Liu, X. Zhang, K. Shao, C. Lin, C. Li, F. Ge and Y. Dong, RSC Adv., 2016, 6, 20938–20948 RSC.
- X. Liu, X. Zhang, K. Zhang and C. Qi, Chemosphere, 2016, 150, 551–558 CrossRef CAS PubMed.
- A. Huang, Z. Zhang, N. Wang, L. Zhu and J. Zou, J. Hazard. Mater., 2016, 302, 158–165 CrossRef CAS PubMed.
- X. Yan, X. Liu, C. Qi, D. Wang and C. Lin, RSC Adv., 2015, 5, 85785–85790 RSC.
- C. Liang, Y. Y. Guo and Y. R. Pan, Int. J. Environ. Sci. Technol., 2014, 11, 483–492 CrossRef CAS.
- K. Zhang, J. Huang, H. Wang, K. Liu, G. Yu, S. Deng and B. Wang, Chemosphere, 2014, 116, 40–45 CrossRef CAS PubMed.
- H. Wang, J. Huang, K. Zhang, Y. Yu, K. Liu, G. Yu, S. Deng and B. Wang, J. Hazard. Mater., 2014, 264, 230–235 CrossRef CAS PubMed.
|
This journal is © The Royal Society of Chemistry 2017 |
Click here to see how this site uses Cookies. View our privacy policy here.